DOI:
10.1039/C6RA15320H
(Paper)
RSC Adv., 2016,
6, 80595-80603
A new visible-light driving nanocomposite photocatalyst Er3+:Y3Al5O12/MoS2–NaTaO3–PdS for photocatalytic degradation of a refractory pollutant with potentially simultaneous hydrogen evolution†
Received
13th June 2016
, Accepted 7th August 2016
First published on 8th August 2016
Abstract
A new visible-light-sensitive photocatalyst, Er3+:Y3Al5O12/MoS2–NaTaO3–PdS, was prepared by hydrothermal, liquid boiling and deposition methods. The prepared photocatalysts were all characterized using an X-ray diffractometer (XRD), energy dispersive X-ray spectroscopy (EDX), scanning electron microscopy (SEM), X-ray photoelectron spectroscopy (XPS), and high-resolution transmission electron microscopy (HRTEM). The photocatalytic activity of the prepared photocatalysts was evaluated via photocatalytic degradation of amaranth as a refractory pollutant with simultaneous hydrogen evolution under visible-light irradiation. Moreover, the effects of the visible-light irradiation time and molar ratio of Er3+:Y3Al5O12 and NaTaO3 on the photocatalytic capability of Er3+:Y3Al5O12/MoS2–NaTaO3–PdS were studied. The results show that the use of Er3+:Y3Al5O12 can improve the utilization ratio of visible light (solar light) for NaTaO3. The optimum ratio of Er3+:Y3Al5O12 and NaTaO3 is 0.45
:
1.00. In addition, a study of extended reusability shows that the prepared photocatalyst can be effectively recycled for three repetitive cycles without an apparent deactivation of the photocatalytic activity. A visible-light photocatalytic degradation mechanism of amaranth with simultaneous hydrogen evolution is also proposed.
1. Introduction
Environmental pollution has become a serious issue that humans must face in the course of societal development.1–3 In recent decades, water pollution has had a great impact, for example from the pollutants that endanger human health through drinking water. Thus, increasing attention needs to be paid to the remediation of water pollution. In particular, some refractory pollutants with stable structures, high toxicity and electron-withdrawing groups are produced by the dyestuffs industry.4 If these refractory pollutants cannot be eliminated immediately, they may persist chronically in the water. This can cause severe and continuous environmental problems.5 A range of conventional technologies, including biological, physicochemical, flocculation and adsorption treatments, have been used to remove aqueous pollutants. Although biological methods have been widely applied in the treatment of various organic pollutants, it requires a long time for microorganisms to degrade refractory pollutants; moreover, some biological methods are obviously not suitable for the treatment of refractory pollutants. In these cases, refractory pollutants might be treated by a non-biodegradation approach. Besides, the aforementioned conventional methods just degrade the pollutants without developing a more efficient and environmentally friendly system for refractory pollutant treatment. Furthermore, degradation of these refractory pollutants without their being utilized is also a waste of resource. Thus, an economic and feasible method should be developed to solve this problem; that is, many refractory pollutants should be considered as an available resource, through an appropriate method that can degrade pollutants whilst simultaneously producing renewable energy. After all, the energy crisis has become a problem of global concern.6–9 Therefore, photocatalysis has recently been recognized as a potential strategy for environmental remediation and at the same time as an opportunity for the production of clean energy, using a suitable semiconductor photocatalyst and solar energy.10–13 If the degradation of organic pollutants and hydrogen evolution are combined through the use of a photocatalytic technique, not only can environmental pollution be avoided, but also hydrogen evolution can be realized at the same time.
Amaranth is a kind of azo compound that is mainly used as colorant and dye in the food and textile industries.14 However, amaranth is a suspected carcinogen. Although there is no reported toxicity to humans to date, it has been forbidden in the food industry in some countries. Amaranth is difficult to degrade completely because its structure contains a nitrogen–nitrogen double bond (–N
N–) and more than one electron-withdrawing group (–SO3Na) (see Fig. 1). In order to eliminate amaranth and produce hydrogen at the same time, we must choose a highly active photocatalyst that has simultaneous strong oxidation and reduction capabilities. In particular, NaTaO3 is one of the widest band gap (Ebg = 4.0 eV) semiconductors and it has attracted considerable attention in the field of photocatalytic degradation and hydrogen generation due to its high performance in photocatalytic reactions (ECB (electric potential of conduction band) = −1.04 eV and EVB (electric potential of valence band) = +2.96 eV).15–18 Therefore, the degradation of amaranth together with simultaneous hydrogen evolution can be realized under irradiation light-matched to the band gap (Ebg = 4.0 eV) of NaTaO3. This photocatalytic reaction process not only effectively degrades organic pollutants, but also provides a convenient technology of hydrogen evolution, which achieves the dual purposes of environmental remediation and the production of clean energy.19,20 However, two negative factors will affect the efficiency of photocatalysis using NaTaO3. One is the low utilization rate of solar light.21–23 Only a small ultraviolet fraction of solar irradiation, less than 5.0%, can be utilized by NaTaO3 because of its wide band gap (Ebg = 4.0 eV), which limits the utilization of cheap solar energy.24–26 Therefore, much research has been undertaken to make NaTaO3 responsive to visible light.27–30 We have made NaTaO3 combine with an up-conversion luminescence agent to improve the utilization of solar light. Recently, we have studied some up-conversion luminescence agents such as NaYF4:Yb/Er, Er3+:YAlO3, Er3+:Y3Al5O12 and Y2SiO5:Pr3+Li, which have been widely used in many fields due to their high-conversion luminescence efficiency.31–35 Er3+:Y3Al5O12, as a highly efficient ultraviolet-visible up-conversion luminescence agent, can emit ultraviolet light under excitation with visible light, and therefore it may be used, in combination with NaTaO3, to achieve the photocatalytic degradation of amaranth with simultaneous hydrogen evolution under solar light irradiation.36–40 For most semiconductor photocatalysts, another negative factor is the rapid recombination of photo-generated electron–hole (e−–h+) pairs. Thus, suitable co-catalysts are selected to load on the surface of the photocatalyst for capturing photogenerated electrons or holes, thereby decreasing the recombination probability of the photogenerated electron (e−)–hole (h+) pairs and enhancing the photocatalytic reaction rate.41–43 Considering the specificity of this reaction, we use dual co-catalysts at the same time, that is, a valence band co-catalyst and a conduction co-catalyst. The valence band co-catalyst can increase the degradation efficiency of the amaranth, and the conduction band co-catalyst can enhance the hydrogen evolution amount.44,45 Based on the above views, we make NaTaO3 combine with Er3+:Y3Al5O12, and then PdS and MoS2 as co-catalysts were loaded onto the surface of the photocatalyst to form a composite photocatalytic system. Therefore, the system can use solar energy and also enhance the efficiency of the degradation of amaranth with simultaneous hydrogen evolution.
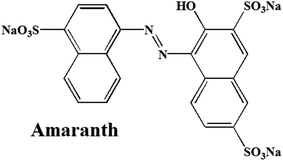 |
| Fig. 1 The structure of amaranth. | |
In this study, an effective up-conversion luminescence agent, Er3+:Y3Al5O12, was prepared by a sol–gel method and nano-sized NaTaO3 particles were obtained by a hydrothermal method. A new visible-light photocatalytic system, Er3+:Y3Al5O12/MoS2–NaTaO3–PdS, was successfully prepared by liquid boiling and deposition methods. The effects of different molar ratios of Er3+:Y3Al5O12 and NaTaO3 on the degradation of amaranth with simultaneous hydrogen evolution under visible-light irradiation were investigated. The experimental results showed that the optimum molar ratio of Er3+:Y3Al5O12 and NaTaO3 is 0.45
:
1.00, under which conditions the rate of photocatalytic degradation and hydrogen evolution can reach a maximum. Furthermore, the reusability of the Er3+:Y3Al5O12/MoS2–NaTaO3–PdS nanocomposite photocatalyst was examined by measuring the visible-light photocatalyzed degradation of amaranth along with simultaneous hydrogen evolution under repeated cycles of use.
2. Experimental
2.1. Materials and reagents
Erbium oxide (Er2O3, 99.999%), yttrium oxide (Y2O3, 99.999%) and aluminum nitrate nonahydrate ((AlNO3)3·9H2O, analytical grade), citric acid (C6H8O7, analytical grade) and nitric acid (HNO3, 65%, analytical pure, Veking Company, China) were used to synthesize the up-conversion luminescence agent (Er3+:Y3Al5O12). Ta2O5 (99.99% purity, Aldrich) and NaOH (99.5%, Sinopharm Chemical Reagent Co. Ltd) were used to prepare the photocatalyst (NaTaO3). Molybdenum sulfide (MoS2, 98%, Sinopharm Chemical Regent Co, Ltd, China) and PdS obtained from sodium sulfide nonahydrate (Na2S·9H2O, 98%, Aladdin Industrial Corporation, China) and palladium chloride (PdCl2, analytical grade, Sinopharm Chemical Reagent Co., Ltd, China) were used as the dual co-catalysts. Amaranth (analytical grade, Shanghai Macklin Biochemical Co., Ltd, China) is used as a target pollutant and an electron donor (the molecular structure is shown in Fig. 1).
2.2. Preparation of Er3+:Y3Al5O12/MoS2–NaTaO3–PdS composite as a visible-light photocatalyst
2.2.1. Synthesis of Er3+:Y3Al5O12 as an up-conversion luminescence agent. Er3+:Y3Al5O12 was synthesized by the sol–gel method. The detailed process is displayed in ESI†.
2.2.2. Preparation of Er3+:Y3Al5O12/NaTaO3. NaTaO3 was prepared by a hydrothermal method. In the process of preparation, 0.8770 g of Ta2O5 and 1.5875 g of NaOH were added to 25 mL of deionized water and stirred for one hour. Different amounts of Er3+:Y3Al5O12 were added to the above solution and continuously stirred for half an hour. The suspension was then transferred to a Teflon-lined stainless steel autoclave (50 mL capacity) that was filled with water to 60% of the volume. The autoclave was maintained at 180 °C for 12 h. After hydrothermal processing, the resulting precipitates were collected by filtering and washed with deionized water several times, and then dried at 80 °C for 4.0 h. Finally, nano-sized sodium tantalate samples with different amounts of up-conversion luminescence agents (Er3+:Y3Al5O12/NaTaO3) were obtained and stored for preparing the visible-light Er3+:Y3Al5O12/MoS2–NaTaO3–PdS photocatalysts.
2.2.3. Preparation of Er3+:Y3Al5O12/MoS2–NaTaO3–PdS. Preparation of Er3+:Y3Al5O12/NaTaO3 with MoS2 (0.20 wt%) and PdS (0.13 wt%) as dual co-catalysts was realized by liquid boiling and deposition methods. The photocatalysts were prepared by the following processes. Firstly, specific amounts of Er3+:Y3Al5O12/NaTaO3 powders were placed in 100 mL of ethanol solution, and 0.20 wt% of commercial MoS2 co-catalyst powder was added. The suspension was then thoroughly dispersed using ultrasound of 80 kHz frequency and 50 W output power for 30 min. It was then heated to boiling point and kept at a constant temperature for 30 min, and then dried at 80 °C for 5.0 h. Next, a PdCl2 aqueous solution (1.10 mmol L−1) was added dropwise to the suspension of Er3+:Y3Al5O12/MoS2–NaTaO3 powder dispersed in Na2S aqueous solution (0.50 mol L−1) and stirred magnetically. After centrifugation, the precipitate was washed thoroughly with deionized water and dried at 60 °C for 10 h. Finally, after thorough grinding, the Er3+:Y3Al5O12/MoS2–NaTaO3–PdS powders were obtained as visible-light photocatalysts.
2.3. Characterization of photocatalysts
The synthesized Er3+:Y3Al5O12, prepared NaTaO3 and Er3+:Y3Al5O12/MoS2–NaTaO3–PdS were characterized by powder X-ray diffraction (XRD, D-8, Bruker-axs, Germany) using Ni filtered Cu Kα radiation in the range of 2θ from 10° to 70°, scanning electron microscopy (SEM, JEOL JSM-5610LV, Hitachi Corporation, Japan), energy dispersive X-ray spectroscopy (EDX, JEOL JSM-5610LV, Hitachi Corporation, Japan), X-ray photoelectron spectroscopy (XPS, Escalab 250XI, Thermo, America) and high-resolution transmission electron microscopy (HRTEM, JEOL-2010 transmission electron microscope, using an accelerating voltage of 200 kV, America).
2.4. Photocatalytic degradation of a refractory pollutant (amaranth) with simultaneous hydrogen evolution
The photocatalytic activities of the prepared photocatalysts were evaluated by the degradation of amaranth as a refractory pollutant in an aqueous solution under visible-light irradiation. During the photocatalytic degradation experiment, 25 mg of photocatalyst was added into 25 mL of amaranth aqueous solution, the initial concentration of which was 5.00 mg L−1. Before visible-light irradiation, the suspension was magnetically stirred in the dark for 30 min to ensure the adsorption–desorption equilibrium between the photocatalyst and amaranth. Then the suspension was irradiated for 8.0 h by using a 300 W xenon lamp with a cut-off filter (LX-300, Deruifeng Hardware Electrical Appliance Businesses, China). The irradiation light wavelength was controlled by a combination of a cold mirror (CM-1) and a water filter (350 nm < λ < 800 nm). For visible-light irradiation, a cut-off filter (L42) was fitted to the aforementioned light source (420 nm < λ < 800 nm). The pollutant concentration of the obtained solution was analyzed using a UV-vis spectrophotometer (Hitachi, U-3310) by checking the absorbance at 521 nm for amaranth. The decolorization efficiency was determined by (1 − At/A0) × 100% formula, in which At was the observed absorbance value of the resultant amaranth solution after a particular irradiation period, and A0 was the initial absorbance value of the amaranth solution.
The visible-light photocatalytic hydrogen evolution experiment was carried out in a 500 mL Pyrex reactor. The headspace of the reactor was connected to an inverted burette which was filled with water at atmospheric pressure, which allowed the measurement of the evolved hydrogen gas. In each run, a specified amount of photocatalyst (1.00 g L−1) was dispersed using a magnetic stirrer in aqueous solution containing amaranth (50 mg L−1). Before visible-light irradiation, the system was refilled with argon for 30 min to remove the air inside and to ensure that the reaction system was under anaerobic conditions. Then, the suspension was exposed to visible-light irradiation for 8.0 h under magnetic stirring. The visible-light source was the same as that for the experiment on photocatalytic degradation. The formation of hydrogen was confirmed by injecting 0.5 mL of the reactor headspace gas in a gas chromatograph (GC-8A, MS-5A column, TCD, Ar Carrier, Shimadazu, Japan).
3. Results and discussion
3.1. XRD, EDX, XPS, SEM and TEM of Er3+:Y3Al5O12, NaTaO3 and Er3+:Y3Al5O12/MoS2–NaTaO3–PdS
The XRD patterns of synthesized Er3+:Y3Al5O12 as an up-conversion luminescence agent, prepared pure NaTaO3 nanoparticles and Er3+:Y3Al5O12/MoS2–NaTaO3–PdS nanocomposite particles with different molar ratios of Er3+:Y3Al5O12 and NaTaO3 are all shown in Fig. S1 (ESI†). As shown in Fig. S1(a) (ESI†), the diffraction peaks of Er3+:Y3Al5O12 were consistent with the values in the standard card (JCPDS card 33-0040). This reveals that the Y3Al5O12 phase had formed and that the Er3+ ions had entered the crystal lattice, partially replacing the Y3+ ions. In Fig. S1(b) (ESI†), from the obvious characteristic diffraction peaks at 2θ = 22.85° (100), 2θ = 32.55° (110), 2θ = 40.23° (111), 2θ = 46.67° (200) and 2θ = 52.58° (210), it can be estimated that all of the peaks of the sample prepared by the hydrothermal synthesis method can be readily indexed to pure NaTaO3 with a cubic structure (JCPDS card 25-0863).46,47 In Fig. S1(c)–(g) (ESI†), the XRD patterns of Er3+:Y3Al5O12/MoS2–NaTaO3–PdS nanocomposite particles with different molar ratios (from 0.00
:
1.00 to 0.65
:
1.00) of Er3+:Y3Al5O12 and NaTaO3 are shown. The main characteristic diffraction peaks of Er3+:Y3Al5O12 and NaTaO3 can be found, which demonstrate that the crystal conformations of NaTaO3 and Er3+:Y3Al5O12 are not changed in Er3+:Y3Al5O12/NaTaO3 nanocomposite particles. Furthermore, all the diffraction peaks of Er3+:Y3Al5O12 in Er3+:Y3Al5O12/MoS2–NaTaO3–PdS nanocomposite particles obviously weaken as against those (Fig. S1(a) (ESI†)) of pure Er3+:Y3Al5O12 particles, but they become conspicuous with an increase in the Er3+:Y3Al5O12 amount. In Fig. S1(c)–(g) (ESI†), it is difficult to observe the characteristic diffraction peaks of MoS2 and PdS, mainly because the amount and scale of MoS2 (0.20 wt%) and PdS (0.13 wt%) are so small that there are insufficient to produce strong diffraction peaks.
The EDX spectra of synthesized Er3+:Y3Al5O12 particles, prepared pure NaTaO3 nanoparticles and Er3+:Y3Al5O12/MoS2–NaTaO3–PdS nanocomposite particles are all shown in Fig. S2 (ESI†). In Fig. S2(a) (ESI†), the result displays the main peaks of Er, Y, Al and O elements, which demonstrates the composition of Er3+:Y3Al5O12 as an up-conversion luminescence agent. Moreover, the atomic ratio is almost consistent with the stoichiometric ratio of Er3+:Y3Al5O12. From Fig. S2(b) (ESI†) the peaks of Na, Ta and O elements which belong to the NaTaO3 nanoparticles can be clearly observed. Based on Fig. S2(c) (ESI†), the constituents of Er3+:Y3Al5O12 and NaTaO3 can be found, but it is hard to find the characteristic peaks of some elements (Mo, Pd and S), which may be covered by other peaks.
The XPS spectra can be used to evaluate the surface condition of the Er3+:Y3Al5O12/MoS2–NaTaO3–PdS nanocomposite photocatalyst. As shown in Fig. S3 (ESI†), the sample is mainly composed of Na, Ta, Mo, Pd, S, Er, Y, Al and O elements. The peaks of Ta (4f) at 25 eV and Na (1s) 1073.4 eV can be clearly observed. The S element is positioned at 166.5 eV (2p), which is confirmed as the S2− ion. And the binding energy peaks of Pd (3d) and Mo (3d) are 337.9 eV and 229 eV, respectively, which indicate that MoS2 and PdS are loaded on the surface of Er3+:Y3Al5O12/NaTaO3 nanocomposite particles. Moreover, Er (4d) at 169 eV, Al (2p) at 72.1 eV, Y (3d) at 156 eV and O (1s) at 531 eV are also shown in Fig. S3 (ESI†). All the peaks of the photocatalyst emerge with a shift due to the charge transfer between adjacent components during the synthetic reaction.
The SEM images of synthesized Er3+:Y3Al5O12 up-conversion luminescence agents, prepared NaTaO3 nanoparticles, Er3+:Y3Al5O12/NaTaO3 nanoparticles and Er3+:Y3Al5O12/MoS2–NaTaO3–PdS nanocomposite particles are all given in this section. From the image of Er3+:Y3Al5O12 depicted in Fig. 2(a), it can be seen that there are numerous homogeneous spherical particles with an average size of 50 nm. As seen in Fig. 2(b), the pure NaTaO3 is well-crystallized in cubic shape with an average size of 180 nm and the exposed crystal faces are considered to be (100) and (110) facets.48 It is a typical morphology for hydrothermally prepared NaTaO3.46,49 In Fig. 2(c) and (d), the morphologies of these two nanoparticles are nearly similar, in which for both it can be found that there are some relatively small spherical particles dispersed on the surface of cube-like crystal particles. Nevertheless, in Fig. 2(d), the MoS2 and PdS particles are not easily observed from the images, due to the scale of the MoS2 and PdS particles, which are very small or highly dispersed on the surface of Er3+:Y3Al5O12/NaTaO3 nanoparticles.
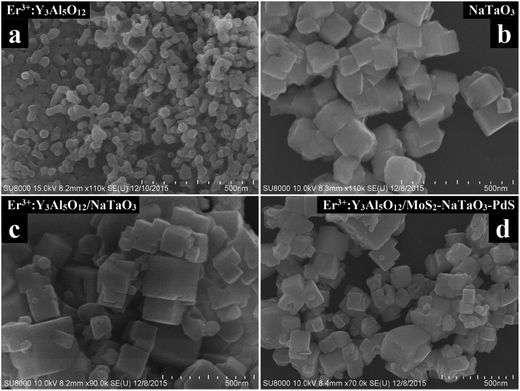 |
| Fig. 2 SEM images. (a) Er3+:Y3Al5O12 powder (heat-treated at 1100 °C for 120 min), (b) pure NaTaO3, (c) Er3+:Y3Al5O12/NaTaO3 nanocomposite photocatalyst (with a 0.45 : 1.00 molar ratio of Er3+:Y3Al5O12 and NaTaO3) and (d) Er3+:Y3Al5O12/MoS2–NaTaO3–PdS nanocomposite photocatalyst (with 0.13 wt% PdS and 0.20 wt% MoS2 contents and a 0.45 : 1.00 molar ratio of Er3+:Y3Al5O12 and NaTaO3). | |
To obtain further insight into the morphology of the photocatalyst, the Er3+:Y3Al5O12/MoS2–NaTaO3–PdS (with a 0.45
:
1.00 molar ratio of Er3+:Y3Al5O12 and NaTaO3) that exhibited the best photocatalytic activity was chosen for HRTEM characterization. Fig. 3 gives an overview of a typical HRTEM image of Er3+:Y3Al5O12/MoS2–NaTaO3–PdS. It can be seen that the Er3+:Y3Al5O12 particles closely adhere to the surface of NaTaO3, resulting in intimate contact. The phase with the lattice fringes (0.389 nm) may be observed, which correspond to the (100) plane of NaTaO3. The lattice fringes (0.250 nm) agree well with the (024) plane of Y3Al5O12. The two inserts in the HRTEM image are the local enlargement of the Er3+:Y3Al5O12/MoS2–NaTaO3–PdS sample. The partial images exhibit two lattice fringes (0.65 nm and 0.61 nm), which correspond to the (100) plane of PdS and (002) plane of MoS2, respectively. The HRTEM micrographs demonstrate the existence of PdS and MoS2 phases, which cannot be detected by XRD measurements.
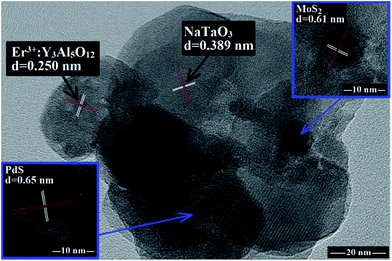 |
| Fig. 3 Partial TEM image of an Er3+:Y3Al5O12/MoS2–NaTaO3–PdS nanocomposite photocatalyst (with 0.13 wt% PdS and 0.20 wt% MoS2 contents and 0.45 : 1.00 molar ratio of Er3+:Y3Al5O12 and NaTaO3). | |
3.2. Photocatalytic activity of the prepared Er3+:Y3Al5O12/MoS2–NaTaO3–PdS in the photocatalytic degradation of refractory pollutants with simultaneous hydrogen evolution
Visible-light photocatalytic hydrogen evolution was evaluated using various Er3+:Y3Al5O12/MoS2–NaTaO3–PdS samples in amaranth aqueous solution as an electron donor. Fig. 4 shows that the visible-light irradiation time impacts on the photocatalytic hydrogen evolution of Er3+:Y3Al5O12/MoS2–NaTaO3–PdS nanocomposite photocatalysts. Moreover, the photocatalytic hydrogen evolution is also related to the molar ratios (from 0.00
:
1.00 to 0.65
:
1.00) of Er3+:Y3Al5O12 and NaTaO3 in Er3+:Y3Al5O12/MoS2–NaTaO3–PdS nanocomposites. For all these five Er3+:Y3Al5O12/MoS2–NaTaO3–PdS photocatalysts, it can be found that the amounts of hydrogen evolution increase substantially with the lengthening of the visible-light irradiation time. At any visible-light irradiation time, the photocatalytic hydrogen evolution increases with increasing content of Er3+:Y3Al5O12 from a 0.00
:
1.00 molar ratio to a 0.45
:
1.00 molar ratio of Er3+:Y3Al5O12 and NaTaO3. This indicates that, in the presence of Er3+:Y3Al5O12, ultraviolet-light can be emitted under excitation with the visible light, which provides more ultraviolet light to make NaTaO3 effectively carry out the photocatalytic hydrogen evolution. That is, compared with the pure NaTaO3, the presence of Er3+:Y3Al5O12 can obviously improve the visible-light photocatalytic hydrogen evolution activity of the NaTaO3 catalyst. And that the photocatalytic activity of the Er3+:Y3Al5O12/NaTaO3 catalyst increases along with the increase of Er3+:Y3Al5O12 content. However, a further increase of the content of Er3+:Y3Al5O12 to a 0.65
:
1.00 molar ratio of Er3+:Y3Al5O12 and NaTaO3 leads to a reduction in the hydrogen evolution. Therefore, a suitable molar ratio of Er3+:Y3Al5O12 and NaTaO3 in the Er3+:Y3Al5O12/MoS2–NaTaO3–PdS composite was necessary for the enhancement of the photocatalytic hydrogen evolution activity. In addition, Fig. 4 also shows the photocatalytic hydrogen evolution with as-obtained photocatalysts with no co-catalysts under visible-light illumination for 8.0 h. It can be clearly seen that the Er3+:Y3Al5O12/NaTaO3 (with a 0.45
:
1.00 molar ratio of Er3+:Y3Al5O12 and NaTaO3) displays relatively poor visible-light-induced hydrogen evolution, indicating that the co-catalysts play a vital role during the photocatalytic reaction process.
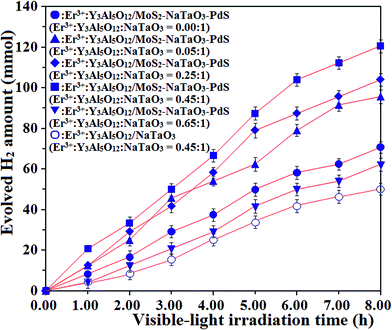 |
| Fig. 4 Effect of visible-light irradiation time on photocatalytic hydrogen evolution of Er3+:Y3Al5O12/MoS2–NaTaO3–PdS (with 0.13 wt% PdS and 0.20 wt% MoS2 contents and 0.00 : 1.00 to 0.65 : 1.00 molar ratio of Er3+:Y3Al5O12 and NaTaO3) and Er3+:Y3Al5O12/NaTaO3 (0.45 : 1.00 molar ratio of Er3+:Y3Al5O12 and NaTaO3) nanocomposite photocatalyst. | |
The degradation of amaranth with simultaneous hydrogen evolution experiment was also performed using Er3+:Y3Al5O12/MoS2–NaTaO3–PdS photocatalysts with different molar ratios of Er3+:Y3Al5O12 and NaTaO3 under visible-light irradiation. As shown in Fig. 5, similarly, the degradation rates of amaranth steadily enhance with an increase of molar ratio of Er3+:Y3Al5O12 and NaTaO3 from 0.00
:
1.00 to 0.45
:
1.00, reaching the maximum at a molar ratio of 0.45
:
1.00. It then decreases with further increases in the molar ratio of Er3+:Y3Al5O12 and NaTaO3. Moreover, the amount of hydrogen evolution continues to increase with increasing Er3+:Y3Al5O12 amount in the Er3+:Y3Al5O12/MoS2–NaTaO3–PdS photocatalysts, reaching the maximum at a molar rate of 0.45
:
1.00, but it also decreases with a further increase of the amount of Er3+:Y3Al5O12. The results are consistent with the photocatalytic degradation of amaranth. Therefore, it can be observed that the optimum molar ratio of Er3+:Y3Al5O12 and NaTaO3 is 0.45
:
1.00. This is because, as a photocatalyst, the NaTaO3 plays a decisive role in the visible-light photocatalytic reaction, but the relative content of NaTaO3 will decrease with the increase of Er3+:Y3Al5O12 content. As an effective up-conversion luminescence agent, the Er3+:Y3Al5O12 only provides more ultraviolet light for the requirement of NaTaO3, but it cannot carry out the photocatalytic reaction itself. Therefore, it is a disadvantage to add an excessive amount of Er3+:Y3Al5O12 to improve the visible-light photocatalytic activity of the Er3+:Y3Al5O12/MoS2–NaTaO3–PdS nanocomposite photocatalyst. Based on the above findings, it is proved that the molar ratio of Er3+:Y3Al5O12 and NaTaO3 can relatively affect the activity of the Er3+:Y3Al5O12/MoS2–NaTaO3–PdS nanocomposite photocatalyst in the visible-light photocatalytic degradation of refractory pollutants with simultaneous hydrogen evolution. In addition, the effect of co-catalysts on the degradation of amaranth with simultaneous hydrogen evolution was investigated after visible-light irradiation for 8.0 h (Fig. 5). Er3+:Y3Al5O12/NaTaO3 without loaded co-catalysts exhibited a low efficiency for the photocatalytic degradation of amaranth with simultaneous hydrogen evolution. Apparently, the presence of co-catalysts on the surface of NaTaO3 is very favorable for the enhancement of the photocatalytic activity of Er3+:Y3Al5O12/NaTaO3 in the degradation of organic pollutant with simultaneous hydrogen evolution.
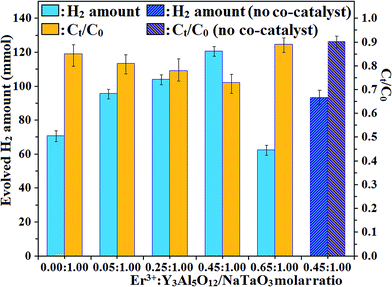 |
| Fig. 5 Effect of molar ratio of Er3+:Y3Al5O12 and NaTaO3 on visible-light photocatalytic degradation of amaranth with simultaneous hydrogen evolution activity of an Er3+:Y3Al5O12/MoS2–NaTaO3–PdS nanocomposite photocatalyst (with 0.13 wt% PdS and 0.20 wt% MoS2 contents) and the effect of a co-catalyst of Er3+:Y3Al5O12/NaTaO3 (with 0.45 : 1.00 molar ratio of Er3+:Y3Al5O12 and NaTaO3) on visible-light photocatalytic degradation of amaranth with simultaneous hydrogen evolution. | |
3.3. Effect of the number of times used on the visible-light photocatalytic degradation of refractory pollutants with simultaneous hydrogen evolution produced by Er3+:Y3Al5O12/MoS2–NaTaO3–PdS
It is worth pointing out that the stability of a photocatalyst is a crucial factor for its practical application. To confirm the stability of the obtained photocatalyst, experiments run for three cycles with Er3+:Y3Al5O12/MoS2–NaTaO3–PdS in the photocatalytic degradation of amaranth with simultaneous hydrogen evolution under visible-light irradiation were examined (as shown in Fig. 6). In this work, the molar ratio of Er3+:Y3Al5O12 and NaTaO3 was 0.45
:
1.00, and the photocatalyst was recycled three times for the same photocatalytic reactions. From Fig. 6, it can be found that there is no significant decrease in the photocatalytic degradation ratio (Fig. 6(a)) and simultaneous hydrogen evolution amount (Fig. 6(b)) after eight hours of irradiation time. That is to say, the photocatalytic degradation ratio and hydrogen evolution amount have a slight decrease as the number of times used increases. Overall, the Er3+:Y3Al5O12/MoS2–NaTaO3–PdS nanocomposites can be effectively recycled for three repetitive cycles without an apparent deactivation of their photocatalytic activity, which demonstrates its great potential as an efficient and stable visible-light photocatalytic material.
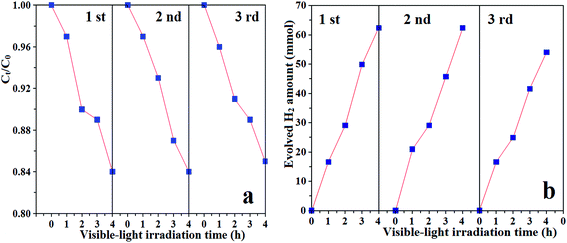 |
| Fig. 6 Effect of times used on the photocatalytic degradation of amaranth with simultaneous hydrogen evolution with the Er3+:Y3Al5O12/MoS2–NaTaO3–PdS nanocomposite photocatalyst (with 0.13 wt% PdS and 0.20 wt% MoS2 contents and 0.45 : 1.00 molar ratio of Er3+:Y3Al5O12 and NaTaO3). | |
3.4. Mechanism and process of visible-light photocatalytic degradation of refractory pollutants with simultaneous hydrogen evolution
In this experiment, a novel system of visible-light photocatalytic degradation of refractory pollutant with simultaneous hydrogen evolution has been designed. A highly active wide band gap semiconductor photocatalyst that has simultaneous strong oxidation and reduction abilities was chosen. However, such semiconductor photocatalyst can be excited only under ultraviolet-light irradiation, and only a small ultraviolet fraction in solar light can be used. Therefore, the utilization of an up-conversion luminescence agent can make photocatalysts increase the use of solar light. In addition, the use of co-catalysts can prevent the recombination of photogenerated electron–hole (e−–h+) pairs, which enhances the photocatalytic activity of the semiconductor photocatalyst. The mechanism for the photocatalytic degradation of refractory pollutants with simultaneous hydrogen evolution caused by an Er3+:Y3Al5O12/MoS2–NaTaO3–PdS nanocomposite photocatalyst under visible-light irradiation is illustrated in Fig. 7.
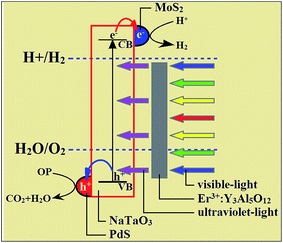 |
| Fig. 7 Principle and mechanism of the photocatalytic degradation of amaranth with simultaneous hydrogen evolution with the Er3+:Y3Al5O12/MoS2–NaTaO3–PdS nanocomposite photocatalyst (with 0.13 wt% PdS and 0.20 wt% MoS2 content and 0.45 : 1.00 molar ratio of Er3+:Y3Al5O12 and NaTaO3). | |
For photocatalytic degradation of refractory pollutants with simultaneous hydrogen evolution, NaTaO3 has been chosen as a suitable photocatalyst due to its wide band gap (Ebg = 4.0 eV), more negative conduction band (CB) and more positive valence band (VB). Based on the band gap positions, the conduction band (CB) and valence band (VB) edge potentials of NaTaO3 are at −1.04 eV and +2.94 eV. Such a negative conduction band (CB) and positive valence band (VB) are enough to easily perform oxidation and reduction reactions at the same time.
As an effective up-conversion luminescence agent, Er3+:Y3Al5O12 can absorb visible light and emit ultraviolet light. Considering these characteristics, Er3+:Y3Al5O12 can be used to combine with NaTaO3 for the requirement of ultraviolet light. For Er3+:Y3Al5O12, the up-conversion signals at 320–342 nm and 354–359 nm can be found by visible 647.2 nm and visible 657.8 nm laser excitations. Otherwise, when Er3+:Y3Al5O12 is pumped by a visible 488 nm Ar+ laser, the up-conversion signals at 271 nm (2H9/2 → 4I15/2) and 318.7 nm (2P3/2 → 4I15/2) and 381 nm (4G11/2 → 4I15/2) can be observed, which can effectively excite the NaTaO3 to produce photogenerated electron–hole (e−–h+) pairs.46,47 Thus, it can be seen that NaTaO3 can be excited under visible-light irradiation from Er3+:Y3Al5O12. As a result, the photogenerated electrons (e−) of NaTaO3 transits to the conduction band (CB), creating holes (h+) in the valence band (VB) at the same time. Then the photogenerated electrons (e−) and holes (h+) can be efficiently separated and transferred to improve the photocatalytic activity of NaTaO3 due to the presence of the dual co-catalysts, MoS2 on the conduction band (CB) and PdS on the valence band (VB), which are effective reduction and oxidation co-catalyst, respectively. Meanwhile, the dual co-catalysts deposited on the surface of NaTaO3 can accept photogenerated electrons (e−) and holes (h+) acting as active sites.48,49 The photogenerated electron (e−) transfers from the conduction band (CB) to the surface of MoS2, and then it can easily react with H+ to evolve hydrogen (H2) due to its high reducibility.50–52 At the same time, the photogenerated hole (h+) is captured by PdS and then it can oxidize the organic pollutant. Therefore, the degradation of refractory pollutants with simultaneous hydrogen evolution can be carried out by using the Er3+:Y3Al5O12/MoS2–NaTaO3–PdS nanocomposite photocatalyst under visible-light irradiation. Based on the above result, it can be found that a semiconductor photocatalyst with a relatively wide band gap is sufficient for application in the degradation of refractory pollutants with simultaneous hydrogen evolution, utilizing solar energy.
4. Conclusions
In this study, Er3+:Y3Al5O12/MoS2–NaTaO3–PdS nanocomposite photocatalysts for the visible-light photocatalytic degradation of refractory pollutants with simultaneous hydrogen evolution were successfully prepared by hydrothermal, liquid boiling and deposition methods. Under visible-light irradiation, the prepared Er3+:Y3Al5O12/MoS2–NaTaO3–PdS nanocomposite photocatalyst exhibits a significantly enhanced photocatalytic activity due to the presence of Er3+:Y3Al5O12 for the degradation of refractory pollutants with simultaneous hydrogen evolution. For high photocatalytic activity, the optimal molar ratio of Er3+:Y3Al5O12 and NaTaO3 was found to be 0.45
:
1.00. It can be confirmed that the Er3+:Y3Al5O12 as an up-conversion luminescence agent provides more ultraviolet light for the requirement of NaTaO3 and the co-catalysts MoS2 and PdS suppress the recombination of photogenerated electron (e−)–hole (h+) pairs. In addition, the prepared photocatalyst can be effectively recycled for three repetitive cycles without an apparent deactivation of the photocatalytic activity. Therefore, the Er3+:Y3Al5O12/MoS2–NaTaO3–PdS nanocomposite photocatalyst is suitable to be applied in wastewater treatment with simultaneous hydrogen evolution by utilizing solar energy.
Acknowledgements
The authors greatly acknowledge the National Science Foundation of China (21371084), the Key Laboratory Basic Research Foundation of Liaoning Provincial Education Department (L2015043), Liaoning Provincial Department of Education Innovation Team Projects (LT2015012), the Natural Science Foundation of Education Department of Liaoning Province (L2015205) of China, the Natural Science Foundation of Science and Technology Department of Liaoning Province (201602346) of China and Shenyang municipal science and technology plan project (F16-205-1-06) of China for financial support. The authors also thank our colleagues and other students for their participation in this work.
References
- M. Wang, J. Ioccozia, L. Sun, C. Lin and Z. Lin, Energy Environ. Sci., 2014, 7, 2182–2202 CAS.
- Z. F. Jiang, D. L. Jiang, Z. X. Yan, D. Liu, K. Qian and J. M. Xie, Appl. Catal., B, 2015, 170–171, 195–205 CrossRef CAS.
- K. Li, Z. X. Zeng, L. S. Yan, S. L. Luoa, X. B. Luo, M. X. Huo and Y. H. Guo, Appl. Catal., B, 2015, 165, 428–437 CrossRef CAS.
- S. Nadupalli, N. Koorbanally and S. B. Jonnalagadda, J. Phys. Chem. A, 2011, 115, 7948–7954 CrossRef CAS PubMed.
- Y. Cui, X. Y. Liu, T. S. Chung, M. Weber, C. Staudt and C. Maletzko, Water Res., 2016, 91, 104–114 CrossRef CAS PubMed.
- C. H. Ma, Y. Li, H. B. Zhang, Y. Chen, C. X. Lu and J. Wang, Chem. Eng. J., 2015, 273, 277–285 CrossRef CAS.
- F. G. Hernández, J. A. Wang, L. F. Chen, X. Bokhimi, R. Gómezd, A. Pérez-Lariosd and N. N. Entzana, J. Hazard. Mater., 2013, 263P, 11–19 CrossRef PubMed.
- G. H. Chen, S. Z. Ji, Y. H. Sang, S. J. Chang, Y. N. Wang, P. Hao, J. Claverie, H. Liu and G. W. Yu, Nanoscale, 2015, 7, 3117–3125 RSC.
- H. Liu, Z. T. Jin and Z. Z. Xu, Dalton Trans., 2015, 44, 14368–14375 RSC.
- X. B. Chen, S. H. Shen, L. J. Guo and A. S. Mao, Chem. Rev., 2010, 110, 6503–6570 CrossRef CAS PubMed.
- T. Y. Gao, G. C. Sun, F. Y. Cheng, K. Dai, H. Chen, K. J. Deng and Q. Y. Huang, RSC Adv., 2015, 5, 28973–28979 RSC.
- J. Tian, Y. H. Leng, H. Z. Cui and H. Liu, J. Hazard. Mater., 2015, 299, 165–173 CrossRef CAS PubMed.
- J. Liang, J. Xu, Q. Gu, Y. G. Zhou, C. C. Huang, H. X. Lina and X. X. Wang, J. Mater. Chem. A, 2013, 1, 7798–7805 CAS.
- S. Nadupalli, N. Koorbanally and S. B. Jonnalagadda, J. Phys. Chem. A, 2011, 115, 11682–11688 CrossRef CAS PubMed.
- C. X. Lu, Y. Chen, Y. Li, C. H. Ma, Y. W. Guo, Y. Li and J. Wang, Energy, 2015, 93, 749–757 CrossRef CAS.
- F. F. Li, D. R. Liu, G. M. Gao, B. Xue and Y. S. Jiang, Appl. Catal., B, 2015, 166–167, 104–111 CrossRef CAS.
- Y. Li, S. G. Li, Y. Li, Y. W. Guo and J. Wang, Int. J. Hydrogen Energy, 2014, 39, 17608–17616 CrossRef CAS.
- H. Husin, H. M. Chen, W. N. Su, C. J. Pan, W. T. Chuang, H. S. Sheu and B. J. Hwang, Appl. Catal., B, 2011, 102, 343–351 CrossRef CAS.
- J. Q. Li, H. Yuan and Z. F. Zhu, J. Mol. Catal. A: Chem., 2015, 410, 133–139 CrossRef CAS.
- Z. Khan, T. R. Chetia and M. Qureshi, Nanoscale, 2012, 4, 3543–3550 RSC.
- Y. Chen, C. X. Lu, L. Tang, S. N. Wei, Y. H. Song and J. Wang, Sol. Energy Mater. Sol. Cells, 2016, 149, 128–136 CrossRef CAS.
- L. L. Xu, X. L. Sun, H. Tu, Q. Jia, H. T. Gong and J. G. Guan, Appl. Catal., B, 2016, 184, 309–319 CrossRef CAS.
- X. Y. Wu, S. Yin, B. Liu, M. Kobayashi, M. Kakihana and T. Sato, J. Mater. Chem. A, 2014, 2, 20832–20840 CAS.
- X. Y. Wu, S. Yin, Q. Dong and T. Sato, Phys. Chem. Chem. Phys., 2013, 15, 20633–20640 RSC.
- C. C. Hu, Y. L. Lee and H. Teng, J. Mater. Chem., 2011, 21, 3824–3830 RSC.
- H. Husin, W. N. Su, H. M. Chen, C. J. Pan, S. H. Chang, J. Rick, W. T. Chuang, H. S. Sheuc and B. J. Hwang, Green Chem., 2011, 13, 1745–1754 RSC.
- P. Kanhere, P. Shenai, S. Chakraborty, R. Ahuja, J. W. Zheng and Z. Chen, Phys. Chem. Chem. Phys., 2014, 16, 16085–16094 RSC.
- P. D. Kanhere, J. Zheng and Z. Chen, J. Phys. Chem. C, 2011, 115, 11846–11853 CAS.
- L. M. Torres-Martínez, A. Cruz-López, I. Juárez-Ramírez and M. E. Meza-de la Rosa, J. Hazard. Mater., 2009, 165, 774–779 CrossRef PubMed.
- X. Li and J. L. Zang, Catal. Commun., 2011, 12, 1380–1383 CrossRef CAS.
- W. Peng, S. Zou, G. Liu, Q. Xiao, J. Meng and R. Zhang, J. Rare Earths, 2011, 29, 330–334 CrossRef CAS.
- L. P. Lu, X. Y. Zhang, Z. H. Bai, X. C. Wang, X. Y. Mi and Q. S. Liu, Adv. Powder Technol., 2006, 17, 181–187 CrossRef CAS.
- J. Wang, Y. P. Xie, Z. H. Zhang, J. Li, X. Chen, L. Q. Zhang, R. Xu and X. D. Zhang, Sol. Energy Mater. Sol. Cells, 2009, 93, 355–361 CrossRef CAS.
- J. Wang, R. H. Li, Z. H. Zhang, W. Sun, R. Xu, Y. P. Xie, Z. Q. Xing and X. D. Zhang, Appl. Catal., A, 2008, 334, 227–233 CrossRef CAS.
- Z. L. Wang, J. H. Hao and H. L. W. Chan, J. Mater. Chem., 2010, 20, 3178–3185 RSC.
- H. Y. Zhu, X. Chen, Z. F. Zheng, X. B. Ke, E. Jaatinen and J. C. Zhao, Chem. Commun., 2009, 45, 7524–7526 RSC.
- R. Dholam, N. Patel, M. Adami and A. Miotello, Int. J. Hydrogen Energy, 2008, 33, 6896–6903 CrossRef CAS.
- S. G. Li, Y. W. Guo, L. Zhang, J. Wang, Y. Li and B. X. Wang, J. Power Sources, 2014, 252, 21–27 CrossRef CAS.
- Y. W. Guo, Y. Li, S. G. Li, L. Zhang, Y. Li and J. Wang, Energy, 2015, 82, 72–79 CrossRef CAS.
- Y. Li, Y. W Guo, S. G. Li, Y. Li and J. Wang, Int. J. Hydrogen Energy, 2015, 40, 2132–2140 CrossRef CAS.
- C. X. Lu, Y. Chen, Y. Li, C. H. Ma, H. B. Zhang, Y. W. Guo and J. Wang, RSC Adv., 2015, 5, 54769–54776 RSC.
- C. Kong, S. X. Min and G. X. Lu, Chem. Commun., 2014, 50, 9281–9283 RSC.
- J. R. Ran, J. Zhang, J. G. Yu, M. Jaroniecc and S. Z. Qiao, Chem. Soc. Rev., 2014, 43, 7787–7812 RSC.
- F. Lin, D. Wang, Z. X. Jiang, Y. Ma, J. Li, R. G. Li and C. Li, Energy Environ. Sci., 2012, 5, 6400–6406 CAS.
- R. G. Li, H. X. Han, F. X. Zhang, D. Wang and C. Li, Energy Environ. Sci., 2014, 7, 1369–1376 CAS.
- X. Li and J. Zang, J. Phys. Chem. C, 2009, 113, 19411–19418 CAS.
- Y. Lee, T. Watanabe, T. Takata, J. N. Kondo, M. Hara, M. Yoshimura and K. Domen, Chem. Mater., 2005, 17, 2422–2426 CrossRef CAS.
- O. Vázquez-Cuchillo, A. Manzo-Robledo, R. Zanella and N. Elizondo-Villareal, Ultrason. Sonochem., 2013, 20, 498–501 CrossRef PubMed.
- J. S. Xu, D. F. Xue and C. L. Yan, Mater. Lett., 2005, 59, 2920–2922 CrossRef CAS.
- H. B. Zhang, C. H. Ma, Y. Li, Y. Chen, C. X. Lu and J. Wang, Appl. Catal., A, 2015, 503, 209–217 CrossRef CAS.
- L. N. Yin, Y. Li, J. Wang, Y. Zhai, Y. M. Kong, J. Q. Gao, G. X. Han and P. Fan, J. Lumin., 2012, 132, 3010–3018 CrossRef CAS.
- H. J. Yan, J. H. Yang, G. J. Ma, G. P. Wu, X. Zong, Z. B. Lei, J. Y. Shi and C. Li, J. Catal., 2009, 266, 165–168 CrossRef CAS.
Footnote |
† Electronic supplementary information (ESI) available: Synthesis of Er3+:Y3Al5O12 as up-conversion luminescence agent. XRD, EDX and XPS of Er3+:Y3Al5O12, pure NaTaO3 and Er3+:Y3Al5O12/MoS2–NaTaO3–PdS nanocomposite. See DOI: 10.1039/c6ra15320h |
|
This journal is © The Royal Society of Chemistry 2016 |