DOI:
10.1039/C6RA15051A
(Paper)
RSC Adv., 2016,
6, 71628-71637
Exploring saccharinate-tetrazoles as selective Cu(II) ligands: structure, magnetic properties and cytotoxicity of copper(II) complexes based on 5-(3-aminosaccharyl)-tetrazoles†
Received
9th June 2016
, Accepted 18th July 2016
First published on 22nd July 2016
Abstract
The role of copper in the proliferation of cancer cells is under investigation and has been explored in the context of cancer chemotherapy. The evidence that proliferation of cancer cells requires a higher abundance of Cu(II) than their normal counterparts has prompted the development of new copper chelators that can avidly bind copper ions, forming redox active metal complexes that ultimately lead to harmful reactive oxygen species (ROS) in neoplasms. In this context, the mandatory properties of the chelators for medical applications are safety (neglectable cytotoxicity), high binding affinity and selectivity towards Cu(II). We report the synthesis, structure (calculations and single crystal X-ray diffraction), spectroscopic (IR; UV-Vis) and magnetic properties of two novel copper(II) complexes based on 5-(3-aminosaccharyl)-tetrazoles (TS and 2MTS), as well as their in vitro cytotoxicity against the human hepatic carcinoma cell line HepG2. Quite interestingly, we found that the saccharinate-tetrazoles tested exhibit strong binding selectivity to Cu(II), over Fe(II) and Ca(II). Additionally, the corresponding copper complexes have shown a huge increase in the in vitro cytotoxicity against tumoral cells, compared to the corresponding nontoxic ligands. Thus, the new ligands may be viewed as potential precursors of selective cytotoxic agents, acting as non-cytotoxic pro-drugs that can be activated inside neoplastic cells, known to be richer in Cu(II) than the corresponding normal cells.
Introduction
The design of ligands remains a topic of intense research interest due to the applications of coordination chemistry in catalysis, supramolecular chemistry, molecular magnetism, optical materials, environmental remediation and medicine.1–4 The discovery of the antiproliferative properties of platinum complexes in the late 1960s stimulated the interest in metal-based compounds as drug candidates for cancer chemotherapy.5,6 Further investigations led to transition metal-based antitumor and antiviral drugs.7–9 Cisplatin [cis-diamminedichloroplatinum(II)] was the first transition metal-based alkylating agent approved for cancer chemotherapy, and was rapidly followed by other platin-based drugs with optimized properties. However, these drugs show severe side effects, such as nephrotoxicity, emetogenesis or neurotoxicity, arising mostly from their indiscriminate attack on all rapidly dividing cells,10–12 and their efficacy is compromised due to relatively fast development of resistance by cancer cells,13,14 calling for the development of coordination compounds with antineoplastic properties and better pharmacological profile.
Copper is an essential trace element in the human body, where it is involved in a variety of vital redox processes.15,16 However, alterations of its normal metabolism convert it into a toxic agent. The potential toxicity of copper arises from its ability to generate reactive oxygen species (ROS) that may react with biomolecules, such as lipids, proteins or nucleic acids.15,17 Elesclomol, a drug candidate currently under clinical evaluation for cancer chemotherapy, is known to exert its potent antineoplastic activity by increasing the levels of ROS in neoplasms. The apoptotic properties evidenced by Elesclomol are ascribed to its coordination to copper(II), that ultimately leads to generation of harmful ROS.18
Studies focused on determining the concentrations of zinc, iron, selenium and copper have shown that, while the zinc, iron and selenium concentrations decrease significantly in serum and tumour tissues of cancer patients when compared to those observed in healthy subjects, copper concentrations generally increase significantly.19–21 These data may support the development of selective tools for cancer chemotherapy.
Copper chelation is also used as a straightforward approach to mitigate impairments due to copper overload or accumulation in organisms. The typical clinical use of copper chelators focuses on reduction of the bioavailability of copper in the organ system. Some candidates are in clinical trials for the treatment of several diseases, including the Wilson's disease.22 In this scenario, the selectivity of a chelator for copper over other metal cations is of upmost relevance.
We synthesized tetrazole-saccharinates (Scheme 1) and investigated their structure in detail.23–25 Studies were then conducted to evaluate the chelation potential of these conjugates towards a range of divalent cations. Quite interestingly, we found that some of the nitrogen-linked conjugates tested act readily as selective copper chelators. Furthermore, the copper complexes have shown a considerably higher in vitro cytotoxicity against tumoral cells than the corresponding ligands. Therefore, the free ligands may be viewed as potential precursors of selective cytotoxic agents, acting as non-cytotoxic pro-drugs that can be activated inside cancer cells, where such ligands may bind intracellular copper ions (present in higher concentration, compared to normal cells), generating complexes that can lead to cell death. This possible mode of action would in fact parallel that proposed for Elesclomol, whose apoptotic properties are ascribed to its coordination with intracellular copper(II) ions, ultimately leading to formation of harmful ROS.
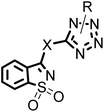 |
| Scheme 1 | |
In this paper we report the synthesis, structural, spectroscopic (IR; UV-Vis) and magnetic properties of two copper(II) complexes (1 and 2; Scheme 2) based on tetrazole 5-aminosaccharinate (TS) and 2-methyltetrazole-5-aminosaccharinate (2MTS), as well as their in vitro cytotoxicity against the human hepatic carcinoma cell line HepG2. We also show that the ligands exhibit strong binding selectivity to Cu(II) over Fe(II) and Ca(II). The knowledge on the spectroscopic properties of the complexes is of fundamental importance to elucidate details of their structures and may serve in future biological studies aimed at unravelling the bioaccumulation, biological targets and mode of action. The magnetic characterization is crucial for biological studies on these compounds using electron paramagnetic resonance (EPR) spectrometry.
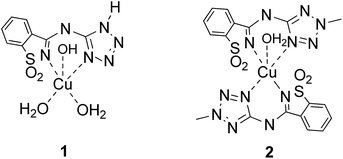 |
| Scheme 2 | |
Experimental
Materials and analytical equipment; general information
All chemicals (analytical grade) were used as purchased. When required, solvents (technical grade) were freshly distilled from appropriate drying agents before use. Melting points were recorded on a Stuart Scientific SMP3 melting point apparatus and are uncorrected. Elemental analyses were performed using a Fisons Instruments EA 1108 CHNS-O elemental analyser. Electronic absorption spectra were recorded on a Varian CARY 50 Bio UV-visible spectrophotometer, using quartz cells (1 cm in diameter). Absorbance changes at selected UV lengths were measured using a 96-well microplate and a Biotek Synergy 4 Microplate Reader. Mass spectra were obtained on a VG 7070E mass spectrometer by electron ionization (EI) at 70 eV. NMR spectra were obtained on a Bruker AM-400 spectrometer, using TMS as the internal reference (δ = 0.0 ppm). IR spectra were obtained using a Bruker FTIR-TENSOR 27 spectrometer equipped with a DTGS detector and Zn/Se optics. Data collection was performed with 64 scans and 4 cm−1 spectral resolution. Single crystal X-ray diffraction (XRD) data were collected on a Bruker APEXII CCD diffractometer using graphite monochromatized Mo Kα radiation (λ = 0.71073 Å). Magnetic measurements were performed with a Quantum Design Dynacool PPMS 9T system, equipped with a vibrating sample magnetometer (VSM) option. Samples in powder form were packed in standard plastic Quantum Design sample holders. Calibration of the magnetometer was performed with a Pd standard sample. Appropriate corrections for the diamagnetic sample holder background signal were applied to the raw data.
Synthesis of the ligands
The ligands were synthesized by coupling saccharyl chloride (1.3 g; 6.45 mmol), previously prepared from saccharin, with 5-aminotetrazole monohydrate or 2-methyltetrazole-5-amine (6.45 mmol), through nucleophilic displacement of chloride, as previously described.24,26 The compounds were purified by recrystallization from a mixture of acetone/ethanol (1
:
1).
N-(1H-Tetrazol-5-yl)-N-(1,1-dioxo-1,2-benzisothiazol-3-yl)amine (TS). Colourless crystals, yield (1.28 g, 80%); mp 270–271 °C (lit,26 270 °C); IR (KBr, cm−1) 3222 (NH), 3090 (NH), 1625 (CN), 1609 (CNC), 1322 (SO2), 1174 (SO2), 1037, 951 (NS) (lit,23,26 3221, 3091, 1623, 1608, 1323, 1172, 1037, 951); 1H NMR (400 MHz, DMSO) δ 8.59–8.47 (m, 1H), 8.22–8.09 (m, 1H), 7.93 (dd, J = 5.5, 3.0 Hz, 2H); MS (EI): m/z: 250 [M]+.
N-(2-Methyl-2H-tetrazol-5-yl)-N-(1,1-dioxo-1,2-benzisothiazol-3-yl)amine (2MTS). Colourless crystals, yield (1.26 g, 74%); mp 280–282 °C (lit,24 285 °C); IR (KBr, cm−1) 3231/3081 (NH; Fermi resonance), 1613 (CN), 1590 (CN), 1523 (CN), 1326 (SO2), 1170 (SO2), 956 (CNC), 836 (lit,24 3231, 3080, 1613, 1590, 1523, 1326, 1169, 956, 835); 1H NMR (400 MHz, DMSO): δ 8.36–8.30 (m, 1H), 8.14–8.09 (m, 1H), 7.97–7.91 (m, 2H), 4.04 (s, 3H); 13C NMR (101 MHz, DMSO): δ 159.86, 149.97, 141.51, 134.34, 133.80, 127.45, 124.28, 121.73, 34.40; MS (EI): m/z 264 [M]+.
Synthesis of the complexes
The complexes (1 and 2; Scheme 2) were prepared from the reaction of the corresponding ligands, TS and 2MTS, with Cu(ClO4)2·6H2O, in acetone and water [Caution! Perchlorate salts of metal complexes with organic ligands are potentially explosive. Only a small amount of material should be prepared, and it must be handled with care].
[Cu(TS)(H2O)2(OH)] (1). A solution of TS (0.050 g, 0.20 mmol) in acetone (20 mL) was added slowly to a stirring solution of Cu(ClO4)2·6H2O (0.074 g, 0.20 mmol) in acetone (5 mL). After addition, the color changed to green and the final solution was stirred for 30 min at room temperature. Distilled water (10 mL) was then added, resulting in instantaneous formation of a green precipitate, which was filtered. The filtrate was solubilized in acetone, then a few drops of H2O were added and the resulting mixture was left for slow evaporation, affording a turquoise crystalline material, albeit of poor quality for single crystal X-ray diffraction. Yield: (0.059 g, 81%); elemental analysis: found: C, 26.06; H, 2.72; N, 22.85; S, 8.59. Calc. for C8H10CuN6O5S: C, 26.27; H, 2.76; N, 22.97; S, 8.77%; IR (KBr pellet, cm−1): 3566, 3175, 1602 (CN), 1568 (CNC), 1275, 1153 (SO2), 981 (NS), 817, 767.
[Cu(2MTS)2(H2O)]·H2O (2). Complex 2 was obtained as described for complex 1. From 2MTS (0.050 g, 0.19 mmol) in acetone (20 mL), added slowly to a stirring solution of Cu(ClO4)2·6H2O (0.070 g, 0.19 mmol) in acetone (5 mL). Green coloured crystals were collected after 2 weeks, filtered, dried under reduced pressure, and characterized by X-ray diffraction. Yield: (0.046 g, 77%); elemental analysis: found C, 34.49; H, 2.87; N, 26.80 S, 9.94. Calc. for C18H18CuN12O6S2: C, 34.53; H, 2.90; N, 26.85; S, 10.24%; IR (KBr pellet, cm−1): 3635, 3526, 1605, 1576 (CN), 1503 (CN), 1388, 1371, 1301 (SO2), 1262, 1161 (SO2), 1114, 960 (CNC), 792, 765, 705.
X-ray crystallography
Data collection was performed on a block-shaped, light green, small single crystal of 2 with dimensions 0.44 × 0.17 × 0.11 mm3, glued on the top of a glass fibre. The unit cell determined from the first 36 CCD frames was found to be triclinic, with cell parameters a = 8.4669(3) Å, b = 11.3079(4) Å, c = 12.7311(4) Å, α = 97.439(2)°, β = 98.638(2)°, γ = 91.496(2)°. Data reduction, including Lorentz, polarization and absorption corrections, were performed using the SAINT and SADABS27 suit of programs. The crystallographic structure was solved by direct methods, using SHELXT-2014/4.28 The solution was found to correspond to the centrosymmetric space group P
. Refinements were carried out with the SHELXL-2014/7 package.29 All refinements were made by full-matrix least-squares on F2 with anisotropic displacement parameters for all non-hydrogen atoms (see Table S1 for details†). All hydrogen atoms could be located on a difference Fourier synthesis. Their positions were refined as riding on parent atoms with an isotropic displacement parameter using SHELXL-2014 defaults that constrain these atoms to idealized positions, except for those of the water molecules that were freely refined with an isotropic displacement parameter equal to 1.5× that of the O atom. The refined structural model gave a final R1 factor of 0.0306 for 4517 reflections with I > 2σ and Rall of 0.0408 for 5483 reflections and 366 parameters. Molecular, crystal packing plots and analysis of intra and intermolecular interactions were performed with PLATON.30
Crystallographic data for the structure of complex 2 have been deposited with the Cambridge Crystallographic Data Centre as a supplementary publication, with no. CCDC 1443606.†
Quantum chemistry calculations
Quantum chemistry calculations for the complex 2 have been performed using the GAMESS (US)31 program, both at the Restricted Open Shell Hartree–Fock (ROHF) and unrestricted density functional theory with the hybrid B3LYP functional (UB3LYP), with a spin multiplicity of 2 (S = 1/2). For the metal atom, the LANL2DZ effective core pseudopotential and basis set32–34 were used. For non-metal atoms, the standard 6-31G(d,p) basis set was used. The geometry of the complex was optimized starting from the observed geometry in the crystal, determined from XRD. Tight conditions for convergence on both energy (SCF cycles) and energy gradient (10−7 atomic units) were employed.
Metal chelating activity
The ligands TS and 2MTS were assayed for their Cu2+, Fe2+ and Ca2+ chelating activity, at different concentrations, and these activities were compared with the chelating activity exhibited by the metal chelator EDTA (ethylenediamine tetraacetic acid)35 or, for calcium ions, EGTA (ethylene glycol tetraacetic acid). EGTA shows a much higher affinity for Ca2+, compared to other cations,36 and is currently used when Ca2+ specific chelation is desired.
The Fe2+, Cu2+ and Ca2+ chelating activities were separately evaluated by spectrophotometry, using selected chelating indicators that produce coloured complexes, following standard methods described in the literature.37,38 The metal ion chelating activities were determined by measuring the colour changes due to the Fe2+/ferrozine complex,39,40 Cu2+/pyrocatechol violet (PV),41–43 and Ca2+/o-cresolphthalein complexone,44,45 respectively. The change in colour of the solutions was measured at selected wavelengths λ (nm), using a microplate reader, and the chelating activity (%) was calculated using eqn (i)41 relative to a control without the ligand under study.
|
 | (i) |
Copper. Solutions of the ligand (30 μL, at 1, 5 and 10 mM) were added to a mixture comprising 100 μL of 0.2 mM CuSO4 and 6 μL of 4 mM pyrocatechol violet, dissolved in 200 μL of 50 mM sodium acetate buffer (pH 6.0), in 96-well microplates. The change in absorbance of the PV solution was measured at 632 nm.
Iron. Solutions of the ligand (30 μL, at 1, 5 and 10 mM) were added to a mixture of 100 μL of 0.5 mM FeCl2 and 12.5 mL of ferrozine solution (40 mM), dissolved in 250 μL of sodium acetate buffer (pH 4.9), in 96-well microplates. The change in colour was measured at 562 nm.
Calcium. Solutions of the ligand (60 μL at 1, 5 and 10 mM) were added to a mixture of 30 μL of 0.5 mM CaCO3 and 20 μL of 0.47 mM o-cresolphthalein complexone, dissolved in 200 μL of ammonium chloride buffer (pH 9.96), in 96-well microplates. The change in colour was measured at 575 nm.Additional details concerning determination of chelating activities are provided in the ESI.†
Toxicity evaluation in vitro
The hepatocellular carcinoma cells (HepG2; ATCC® HB-8065™) were maintained in Dulbecco's modified eagle medium (DMEM) supplemented with 10% heat-inactivated fetal bovine serum (FBS), 1% L-glutamine (2 mM), and 1% penicillin (50 U mL−1)/streptomycin (50 μg mL−1), at 37 °C in a humidified atmosphere with 5% CO2.
Cell viability assay. Exponentially growing HepG2 cells were plated in 96-well tissue plates at a density of 5 × 103 cells per well and incubated for 24 h. Compounds were then applied at various concentrations (0–100 μM) for 72 h. Control cells were treated with dimethyl sulfoxide (DMSO) at the highest concentration used in test wells (0.5%), and cell viability was determined by the MTT [3-(4,5-dimethylthiazol-2-yl)-2,5-diphenyltetrazolium bromide] colorimetric assay.46 Briefly, 2 hours prior to the end of the incubation period 20 μL of MTT (5 mg mL−1 in PBS (phosphate-buffer saline)) were added to each well and further incubated at 37 °C. Then, 150 μL of DMSO were added to each well in order to dissolve the formazan crystals and absorbance was measured at 590 nm (Biotek Synergy 4). Results were expressed in terms of IC50 values of cell viability (μM). All experiments were run in triplicate. The IC50 values were calculated by sigmoidal fitting of the data in the GraphPad Prism V 5.0 program (GraphPad Software, La Jolla, CA, USA).
Results and discussion
X-ray crystal structure analysis
We were not successful in obtaining crystals of complex 1 suitable for XRD single crystal structure determination. On the other hand, proper crystals were obtained for complex 2.
Single-crystal XRD structure determination of 2 showed that the Cu(II) ion is coordinated by two bidentate 2MTS ligands and one water molecule (Fig. 1). In addition, the structure contains a non-coordinated extra water molecule that plays a significant role in the supramolecular arrangement of the complexes in the crystal structure via a network of hydrogen bonds. In the complex, the methyltetrazole moiety of the 2MTS ligand has the methyl group oriented in the trans conformation with respect to the thiazole group (Fig. 1), whereas in the crystal of the ligand it adopts the syn conformation24 (see Fig. S2†). As the energy of the two conformers of the ligand are quite similar,24 steric hindrance in the complex likely determines the preference for the trans form.
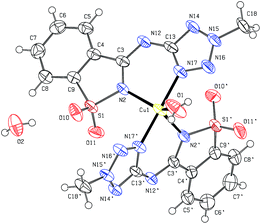 |
| Fig. 1 ORTEP plot of complex 2, showing the atom numbering scheme. Anisotropic displacement ellipsoids are depicted at the 50% probability level. | |
The Cu2+ 5-coordination is intermediate between a trigonal bipyramidal (TP) and a square pyramidal (SP) geometry, and such geometry can be described by a Berry pseudo-rotation with N2′ as pivot atom.47,48 The observed coordination geometry is at 23.8% along the TP (D3h) → SP (C4v) pathway, assuming a standard default value for the trans basal angle of 150°. The τ geometric descriptor,49 that has a value of 1 for TP and 0 for SP coordination geometries, takes a value of 0.75 in the present case. The Cu(II)–N distances are in the range 1.942–2.116 Å, within typical values for such distances. The Cu(II)–O distance to the water molecule within the coordination shell is 2.071 Å. Comparing the geometry of the anionic form of the ligand to that found in the published crystal form of the neutral molecule24 shows that deprotonation of the amine N12 atom has significantly shortened both N12–C13/N12′–C13′ and N12–C3/N12′–C3′ distances, from 1.386 Å to 1.353/1.360 Å and from 1.348 Å to 1.302/1.308 Å, respectively, and decreased the angle C3–N12–C13/C3′–N12′–C13′ from 124.66 to 120.35/120.08°, whereas the N2–C3/N2′–C3′ distance increased from 1.299 Å to 1.364/1.357 Å. The torsion angles around the N12–C13 and N12–C3 bonds are smaller than ∼6° in the complex, compared to a maximum value of ∼15° in the crystal of the neutral molecule. Accordingly, the aromatic rings of benzisothiazole and tetrazole are closer to coplanarity, with angles between least-squares planes of the rings reduced from 17.2° to 1.1°/9.2° for the two ligand molecules of the complex.
Stabilization of the crystal structure is achieved mainly by an extensive network of hydrogen bonds involving the two water molecules (Table 1). The water molecule coordinated to the metal ion is involved in an intramolecular hydrogen bond with the N16 atom, and also acts as proton donor to a neighbour uncoordinated water molecule that, itself, joins two molecules via hydrogen bonds to atoms O10 and N12′, such pattern propagating as an infinite chain of hydrogen bonds along the a-axis (Fig. 2). In addition to these hydrogen bonds involving the water molecules, there are other weaker C–H⋯O, C–H⋯N bonds and π–π interactions between the electron clouds of the aromatic rings. The latter occur between stacked aromatic rings related by an inversion centre (Fig. 3).
Table 1 Distances and angles of inter and intramolecular hydrogen bonds involving the water molecules in the crystal of complex 2a
|
D–H (Å) |
H⋯A (Å) |
D⋯A (Å) |
D–H⋯A (°) |
Symmetry codes: (i)1 − x, −y, 1 − z; (ii)−x, −y, 1 − z. In addition, weaker intermolecular interactions between C–H groups as donors and N and O atoms as acceptors with D⋯A distances in the range 2.895 and 3.377 Å are spotted in the structure (details given in the ESI Table S2). |
O1–H1A⋯O2(i) |
0.85(4) |
1.78(4) |
2.623(3) |
172(4) |
O1–H1B⋯N16 |
0.63(4) |
2.60(5) |
2.948(3) |
117(5) |
O2–H2A⋯N12′(ii) |
0.84(4) |
2.14(4) |
2.966(2) |
168(3) |
O2–H2B⋯O10 |
0.77(4) |
2.11(4) |
2.886(2) |
176(4) |
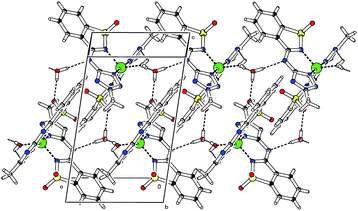 |
| Fig. 2 Intermolecular hydrogen bond network involving water molecules. | |
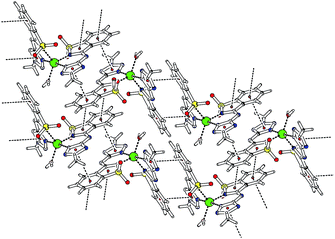 |
| Fig. 3 Network of interactions between π-electron clouds of the aromatic rings. | |
Quantum chemistry calculations
In order to gain further insight on the stability of the new Cu(II) complexes as isolated species from the crystalline structure, we performed ROHF and DFT/UB3LYP quantum chemistry calculations on complex 2, optimizing its geometry starting from that observed in the crystal, as given by the XRD data (Table 2). The isolated complex is stable at both levels of theory, although the uncoordinated water molecule, that was also included in the calculations, is only loosely bound to the complex and drifts considerably from the starting position, as it could be anticipated. Calculated coordination bond distances in the isolated complex do not differ very much from those observed in the crystal, except for the copper–water (Cu–O1) distance, that is predicted by both theoretical methods to be significantly longer for the isolated species than found in the crystal. Concerning the coordination valence angles, the calculations favour larger N2–Cu–N2′ and O1–Cu–N2 at the expense of shorter O1–Cu–N2′ angles. A graphical comparison of the calculated and observed geometries, where superposition of the compared structures is maximized, is provided as ESI (Fig. S3†).
Table 2 Comparison of the calculated equilibrium geometry (ROHF and DFT/UB3LYP) parameters of the isolated complex 2 with those observed in the crystal form (XRD)a
|
XRD |
ROHF |
UB3LYP |
Distances in Å; bond angles in degrees. See Fig. 1 for atom numbering. |
Cu–N2 |
2.072(2) |
2.119 |
2.097 |
Cu–N2′ |
2.116(2) |
2.153 |
2.153 |
Cu–O1 |
2.071(2) |
2.261 |
2.249 |
Cu–N17 |
1.951(2) |
2.047 |
2.000 |
Cu–N17′ |
1.942(2) |
2.050 |
2.005 |
N2–Cu–N2′ |
112.24(7) |
120.51 |
123.20 |
O1–Cu–N2′ |
117.11(8) |
86.76 |
86.31 |
O1–Cu–N2 |
130.65(8) |
152.10 |
150.11 |
N17–Cu–N17′ |
175.81(7) |
171.34 |
175.12 |
Infrared spectroscopy of the solid polycrystalline compounds
The IR spectra of the free ligands, TS and 2MTS, and respective metal–ligand systems (1 and 2) are shown in Fig. 4. TS and 2MTS have been studied previously by X-ray crystallography and infrared spectroscopy.23,24 In those studies, TS and 2MTS were found to exist in the crystals in an amino-bridged tautomeric form (see Fig. S1 and S2 in the ESI†). In the infrared spectra of the two crystals, the νNH stretching bands could be easily identified, since this vibration gives rise to bands in a very characteristic frequency range, 3300–3050 cm−1. For TS, with a NH group as spacer and a hydrogen substituent in the tetrazole ring, two main bands were observed, at 3222 and 3090 cm−1. These bands were ascribed to the νNH stretching vibrations of the bridge and on tetrazolyl ring NH groups, respectively. With the formation of complex 1, the bridged nitrogen atom deprotonates and, accordingly, the band observed at 3222 cm−1 in the spectrum of the free ligand has no equivalent. In turn, the band due to the νNH stretching vibration of the tetrazole NH bond is observed slightly shifted to a higher frequency, compared to its position in the spectrum of the free ligand, appearing at 3132 cm−1. For 2MTS, the νNH stretching vibration of the bridging NH group is observed as a Fermi doublet at 3230/3081 cm−1. As expected, this feature disappears upon complexation, due to deprotonation. In the high-frequency spectral range, one should also notice the presence of additional bands (within the 3700–3200 cm−1 region) in the spectra of both complexes 1 and 2, due to the νOH stretching vibrations present in these species.
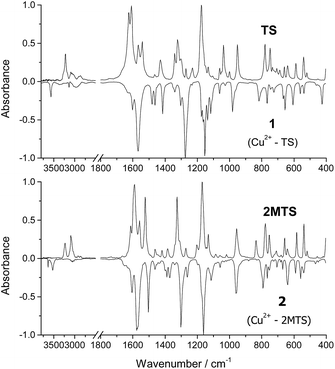 |
| Fig. 4 Top: Room temperature IR spectra of ligand TS (positive bands) and of complex 1 (negative bands). Bottom: Room temperature IR spectra of ligand 2MTS (positive bands) and of complex 2 (negative bands). All spectra were obtained for the compounds in a KBr pellet. | |
In the low-frequency spectral range, the absorption profiles observed in the spectra of the free ligands were also modified upon complexation. The most pronounced changes are observed in the 1650–1540 cm−1 region, where characteristic vibrations due to the bridging groups absorb. This reflects the changes in the electron density of the bridging system (NCNCN) caused by deprotonation of the amine moiety and formation of a conjugated system. It is also a direct evidence for the involvement of this delocalized system in metal binding. The frequencies of the major bands in the IR spectra of the free ligands and of the complexes are provided as ESI (Fig. S4–S7†). For the complexes, a short summary of the IR bands observed is also given in the Experimental section of this article.
Magnetometry results
Magnetization was measured as function of temperature for complex 2 under an applied magnetic field of 0.1 T, in the temperature range 1.8–300 K. A plot of the magnetic susceptibility as function of temperature is shown in Fig. 5 (left). A fit to the data of a modified Curie–Weiss law, χm = C/(T − θ) + χ0, where χ0 is a temperature independent term and C and θ are the molecular Curie–Weiss constant and temperature, respectively, gave values of C = 0.438 cm3 mol−1 K−1, θ = −0.53 K, and χ0 = 5.69 × 10−5 cm3 mol−1. The compound shows paramagnetic behaviour down to low temperature, with an increase of the susceptibility above Curie–Weiss law observed below T ≈ 5 K. From the fitted value of the molecular constant, a value of the effective magnetic moment of the Cu ion of 1.87 μB is obtained, close to the spin-only theoretical value of a Cu(II) ion (1.73 μB). The M(H) magnetization curves (Fig. 5, right) confirm the paramagnetic character of the compound down to 2 K. The saturation magnetization at 9 T and 2 K is 1.066 μB per Cu atom corresponding to a g value of 2.14 for a S = 1/2 state, to be compared with the g = 2.16 determined from the paramagnetic effective moment obtained from the fit of a modified Curie–Weiss law to the M(T) data.
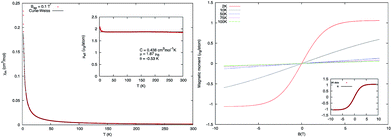 |
| Fig. 5 Left: Molar magnetic susceptibility of complex 2 as function of temperature measured with an applied magnetic field of 0.1 T. The line corresponds to the least-squares fit of the data to a modified Curie–Weiss law, χm = C/(T − θ) + χ0. The insert depicts the effective moment, where n = 1 is the number of magnetic ions per formula unit and θ and χ0 are given from the fit. Right: Magnetization as function of field, expressed as magnetic moment per Cu ion, for complex 2. The magnetization curves follow closely the paramagnetic Brillouin function (insert: fit to the Brillouin function of the 2 K data). At 2 K, the saturated paramagnetic moment at 9 T is 1.07 μB per Cu atom. | |
Magnetometry measurements for complex 1 showed similar paramagnetic behaviour (Fig. S8 and S9, provided as ESI†). Assuming a S = 1/2 state for the Cu2+ ion, the obtained data implies a 1
:
1 metal/ligand stoichiometry and, additionally, two water molecules and a OH− counterion per formula unit. For such stoichiometry, the fitted magnetic parameters of the Cu ion are μeff = 1.86 μB, θ = −0.88 K, χ0 = 1.32 × 10−5 cm3 mol−1, g = 2.03 and μ (9 T) = 1.02 μB per Cu atom.
UV-Vis titrations
Typical titration experiments were performed by sequential additions of 2–4 μL of a freshly made solution of the metal ion (2 mM) to 1.5 mL of a solution of the ligand (50 μM, from 1 mM stock solution), in a quartz cuvette. The mixture was equilibrated, at 25 °C, until no further spectroscopic changes were observed (ca. 1 min).
UV-Vis titrations of TS and 2MTS with Cu2+ were carried out in ethanol. With addition of Cu2+, the absorption band due to free TS (λmax = 258 nm) decreased rapidly in intensity and new absorption bands (λmax = 270, 287, 304 and 314 nm), due to complex 1, appeared and increased in intensity (Fig. 6, top). The presence of clear isosbestic points at 245 and 268 nm suggests a clean formation of complex 1. The inset of Fig. 6 (top) displays a plot of the absorbance of complex 1, at 314 nm, versus Cu2+ concentration, indicating that the complexation reaction occurs with formation of a 1
:
1 metal–ligand (M
:
L) complex, in consonance with the results from the magnetic measurements.
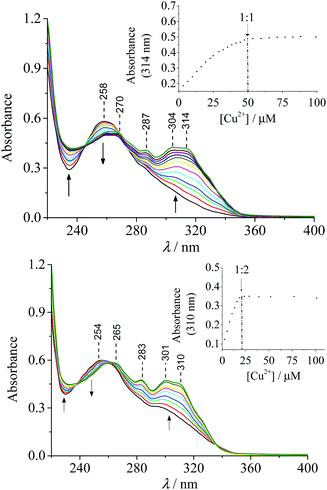 |
| Fig. 6 Titration of TS (top) and 2MTS (bottom) with Cu2+, in ethanol, by sequential additions of 2–4 μL of Cu2+ solution (2 mM) to the same 1.5 mL of ligand solution (50 μM). Insets: titration curves: top, absorbance of complex 1 at 314 nm versus Cu2+ concentration; bottom, absorbance of complex 2 at 310 nm versus Cu2+ concentration. | |
The titration of 2MTS with Cu2+ also revealed a decrease in intensity of the absorption band due to free 2MTS (λmax = 254 nm), concomitant with the increase in intensity of new absorption bands (λmax = 265, 283, 301 and 310 nm) due to complex 2. Two isosbestic points were observed, at 238 nm and 260 nm (Fig. 6, bottom). The titration curve of complex 2 at 310 nm revealed that, unlike for complex 1, beyond ca. 0.5 mol eq. of Cu2+ the spectrum remained virtually unaltered, which indicates that the complexation reaction occurs with formation of a 1
:
2 metal–ligand (M
:
L) complex, in consonance with both the XRD and magnetometry measurements.
The experimental absorbance values (A) obtained were used to calculate the molar absorptivity (ε) and formation constants (KC) of the complexes, using the Rose–Drago eqn (ii),50,51 an extended form of the Benesi–Hildebrand equation,52 where CA and CD are the initial concentrations of the Cu2+ and of the ligand (TS or 2MTS), respectively.
|
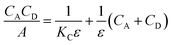 | (ii) |
|
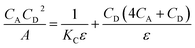 | (iii) |
A typical Rose–Drago plot, i.e. the plot of CACD/A vs. (CA + CD) for 1
:
1 stoichiometry, was drawn for the reaction of TS with Cu2+ (Fig. 7, left). The linearity of such plot is again in keeping with the formation of a 1
:
1 Cu2+–TS complex. In the plots, the slope and intercept are equal to 1/ε and 1/εKC, respectively. The values of both KC and ε are given in Table 3. On the contrary, the scattered experimental data for the Cu2+–2MTS complex suggest that the stoichiometry of the complex is different from 1
:
1. In fact, when using the equation for the 1
:
2 stoichiometry (iii),53,54 and drawing the plot of CACD2/A vs. CD(4CA + CD), a straight line was obtained (Fig. 7, right).
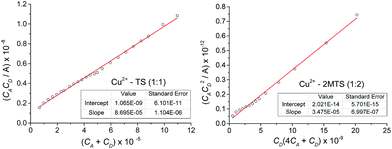 |
| Fig. 7 Left: Rose–Drago linear plot for the titration of TS with Cu2+, in ethanol. The plot was drawn considering the equation for the 1 : 1 stoichiometry (M : L). Right: Rose–Drago linear plot for the titration of 2MTS with Cu2+, in ethanol. The plot was drawn using the equation for the 1 : 2 stoichiometry (M : L). The slope and intercept are equal to 1/ε and 1/εKC, respectively. | |
Table 3 Apparent binding constants (KC) for Cu2+–TS and Cu2+–2MTS complexes in ethanol, at 25 °C, calculated form the maximum absorbance of complexes 1 and 2 at 314 and 310 nm, respectively
Complex |
Complex absorption (nm) |
(ε) L mol−1 cm−1 |
KC |
(1) Cu2+–TS (1 : 1) |
314 |
1.15 × 104 |
1.08 × 1013 (M−1) |
(2) Cu2+–2MTS (1 : 2) |
310 |
2.88 × 104 |
1.42 × 1018 (M−2) |
The UV-Vis spectra of both complexes show bands below 280 nm due to π → π* and n → π* transitions from the ligands (Fig. 6). The new bands observed at 270 nm, for complex 1, and at 265 nm, for complex 2, may be ascribed to a red-shift of the 258 nm TS absorption band and 254 nm 2MTS absorption band, respectively, due to the enhanced delocalization of the π electron system of the ligands upon coordination, induced by the positively charged metal centre. The new broad absorptions at 304 and 314 nm, for complex 1, and at 301 and 310 nm, for complex 2, are attributed to charge transfer interactions. These new bands show extinction coefficients ε314 nm ≈ 11
500 M−1 cm−1 for complex 1 and ε314 nm ≈ 28
777 M−1 cm−1 for complex 2. The large values of KC reflect the high stability of the complexes.
Metal chelating activities
The ligands TS and 2MTS were assayed for their Cu2+, Fe2+ and Ca2+ chelating activities, at different concentrations, and these activities were compared with the chelating activities exhibited by the metal chelator EDTA or, for calcium ions, the related EGTA chelator. EGTA shows a much higher affinity for Ca2+, compared to other cations,36 and is currently used when Ca2+ specific chelation is desired.
As shown in Table 4, both TS and 2MTS ligands show good chelating activity towards Cu2+, over 92% and 74%, respectively, at 5 mM concentrations. These values are high, even when compared with the value obtained for the standard chelator EDTA (88%, at 3.4 mM). Remarkably, no evidence for chelating ability of TS and 2MTS towards Fe2+ was observed, even at higher concentrations (10 mM), while the standard EDTA (3.4 mM) showed ca. 90% of chelating activity in the same conditions. Nevertheless, since this experiments were performed in competition with the strong Fe2+-chelator ferrozine (K = 3.65 × 1015), used as indicator,55 we further scrutinize the ability of the studied ligands to chelate Fe2+, through UV-Vis titration experiment for this metal. However, additions of Fe2+ to solutions of the ligands did not lead to spectral changes, confirming the absence of chelating ability of TS and 2MTS to Fe2+. Also, when tested for Ca2+ chelation, only 2MTS shows a minor chelating ability (ca. 2.7%), when using the highest concentration of calcium tested (10 mM), yet a negligible value if compared with the ca. 81% demonstrated for the standard EGTA, at 1 mM. Thus, TS and 2MTS exhibit high efficacy and selectivity as copper chelators.
Table 4 Chelating activities (%) of TS and 2MTS, on Cu2+, Fe2+ and Ca2+ ionsa
Compound |
Concentration (mM) |
Cu2+ |
Fe2+ |
Ca2+ |
Values are means ± SEM, n = (6); “—”, without evidence of chelating activity (no change in absorbance of metal chelating indicator); n/a, not applicable. EDTA was used as positive control for Cu2+ and Fe2+ chelating activity. EGTA was used as positive control for Ca2+ chelating activity. |
TS |
1 |
62.2 ± 0.74 |
— |
— |
5 |
92.1 ± 0.05 |
— |
— |
10 |
93.1 ± 0.02 |
— |
— |
2MTS |
1 |
24.9 ± 0.66 |
— |
— |
5 |
74.3 ± 0.43 |
— |
— |
10 |
86.8 ± 0.13 |
— |
2.7 ± 1.13 |
EDTAb |
3.4 |
88.5 ± 0.12 |
93.9 ± 0.32 |
n/a |
EGTAc |
1 |
n/a |
n/a |
80.7 ± 0.74 |
The investigation of cellular processes related to copper transport, storage and usage often involves strategic alterations of the bioavailability of copper ions by using metal chelating agents. When taking advantage of live cells in probing for biomolecules related to copper homeostasis, a typical approach is to change the availability of copper and search for subsequent changes in cell processes. Up-regulation or down-regulation of a specific set of genes can identify the proteins that are directly or indirectly related to copper handling. An accurate control of the ability of a chelator to limit the bioavailability of copper is thus crucial to the integrity of an experiment and to the interpretation of the results. In the studies of physiological and pathological activity of copper-dependent compounds, EDTA has often been used as a classic Cu2+ chelator in experiments to modulate copper concentrations in the cell, or to terminate biochemical processes of copper-containing compounds.56–58 However, chelators such as EDTA usually lack selectivity, easily causing the loss of beneficial ions, such as iron and calcium.59–61 In this context, due to its high selectivity to copper ions and their excellent chelator activity to this species, TS and 2MTS appear as promising chelators for investigations of copper-related processes in biological environment.
Cytotoxicity assays
Considering possible applications in medicine, the safety of TS and 2MTS was evaluated by means of cytotoxicity studies. The cytotoxicity of the compounds was tested using the hepatocellular carcinoma cell line (HepG2) and the standard MTT assay. Compounds were applied at 100 μM, for 72 h. Results were expressed as % of cell viability, relatively to the negative control (Table 5). None of the tested metal-free ligands TS and 2MTS exhibited cytotoxicity against the tumour cell lines (at 100 μM). However, complexes 1 and 2 were found to exhibit potent cytotoxicity, much higher than Cu2+ alone, clearly evidencing that there is a significant increase in cytotoxicity when the copper is chelated by the ligands TS or 2MTS.
Table 5 Cellular viability (% of the control) of the compounds (at 100 μM), tested against the human hepatic tumour cell line HepG2a
Negative control cells were treated with DMSO at the highest concentration used in test wells (0.5%). |
Compound |
TS |
2MTS |
1 |
2 |
CuSO4 |
HepG2 |
94.3 ± 3.6 |
95.1 ± 2.9 |
3.59 ± 0.09 |
3.56 ± 0.03 |
62.1 ± 4.0 |
The half maximal inhibitory concentration (IC50) for complexes 1 and 2 are 11.1 ± 0.1 μM and 18.4 ± 1.6 μM, respectively (Table 6). The cytotoxic activities of 1 and 2 can be compared with that of the known anticancer drug etoposide, used as a reference in our studies. The results thus show that complexes 1 and 2 and etoposide show cytotoxic properties against the human hepatic tumoral cell line HepG2 at low micromolar concentrations, although the cytotoxicity of etoposide is somewhat higher.
Table 6 Cytotoxicity (IC50 μM) of complexes 1 and 2 and etoposide (positive control) against the human hepatic tumoral cell line HepG2a
Negative control cells were treated with DMSO at the highest concentration used in test wells (0.5%). |
Compound |
1 |
2 |
Etoposide |
IC50 (μM) |
11.1 ± 0.1 |
18.4 ± 1.6 |
2.34 ± 0.17 |
Conclusions
In the present study, new copper(II) complexes (1, 2) based on tetrazole-5-aminosaccharinate (TS) and 2-methyltetrazole-5-aminosaccharinate (2MTS), respectively, were prepared and their structural, spectroscopic and magnetic properties were investigated. The crystal structure of complex 2 was studied by single crystal X-ray diffraction, showing that the Cu(II) ion is coordinated by two bidentate 2MTS ligands and one water molecule. In addition, the structure contains a non-coordinated water molecule. Stabilization of the crystal structure is mainly due to an extensive network of hydrogen bonds involving the two water molecules. The stability of the Cu(II) complex 2 as isolated species from the crystalline structure was investigated by ROHF and DFT calculations. The isolated complex is stable at both levels of theory, and calculated coordination bond distances in the isolated complex did not differ very much from those observed in the crystal. Magnetic studies confirm the paramagnetic character of both Cu(II) complexes (1, 2). Assuming a S = 1/2 state for the Cu(II) ion, the magnetometry measurements for complex 1 imply a 1
:
1 metal/ligand stoichiometry and, additionally, two water molecules and an OH− counterion per formula unit, in agreement with the elemental analysis found for the compound. UV-Vis titrations of ligands TS and 2MTS with Cu2+ were carried out in ethanol. Also UV-Vis titration of the ligand TS with Cu2+ revealed that the complexation reaction occurs with formation of a 1
:
1 metal–ligand (M
:
L) complex, in consonance with the results from the magnetic measurements. On the other hand titration of ligand 2MTS, indicates that the complexation reaction occurs with formation of a 1
:
2 metal–ligand (M
:
L) complex, in consonance with both the XRD and magnetometry measurements.
TS and 2MTS were assayed for their metal ion chelating activities and were found to exhibit strong binding selectivity to Cu(II), over Fe(II) and Ca(II). Moreover, when tested in vitro against the hepatocellular carcinoma cell line HepG2, the complexes exhibited a huge increase in cytotoxicity, compared to the corresponding ligands. The half maximal inhibitory concentrations (IC50) measured were 11.1 ± 0.1 μM and 18.4 ± 1.6 μM, for complexes 1 and 2, respectively. Although the cytotoxicity of etoposide, used as reference, is somewhat higher (IC50 of 2.34 ± 0.17 μM), the low micromolar cytotoxic activity exhibited by complexes 1 and 2 against the human hepatic tumoral cell line HepG2 combined with the observed selectivity of TS and 2MTS ligands to copper ions, may offer perspectives for new leads in cancer chemotherapy, especially for tumours where neoplasm cells have a higher concentration of Cu2+ than normal cells.
Acknowledgements
The authors gratefully acknowledge Fundação para a Ciência e a Tecnologia (FCT), and COMPETE, through project UID/Multi/04326/2013. The Coimbra Chemistry Centre (CQC) is supported by FCT through project UI0313/QUI/2013. Access to TAIL-UC facility funded under QREN-Mais Centro project ICT_2009_02_012_1890 is gratefully acknowledged. A. Ismael thanks FCT for financial support through grant SFRH/BD/90435/2012. Catarina G. Pereira is acknowledged for kind assistance in some experiments.
References
- G. Aromí, L. Barrios, O. Roubeau and P. Gamez, Coord. Chem. Rev., 2011, 255, 485–546 CrossRef.
- H. Zhao, Z.-R. R. Qu, H.-Y. Y. Ye and R.-G. G. Xiong, Chem. Soc. Rev., 2008, 37, 84–100 RSC.
- E. J. Baran and V. T. Yilmaz, Coord. Chem. Rev., 2006, 250, 1980–1999 CrossRef CAS.
- J. M. Fisher, R. J. Potter and C. F. J. Barnard, Platinum Met. Rev., 2004, 48, 101–104 CrossRef.
- B. Rosenberg, L. Van Camp, E. B. Grimley and A. J. Thomson, J. Biol. Chem., 1967, 242, 1347–1352 CAS.
- D. A. Christie and E. M. Tansey, in Wellcome Witnesses to Twentieth Century Medicine, London, 2007, vol. 30, pp. 35–36 Search PubMed.
- S. Komeda and A. Casini, Curr. Top. Med. Chem., 2012, 12, 219–235 CrossRef CAS PubMed.
- B. M. Paterson and P. S. Donnelly, Chem. Soc. Rev., 2011, 40, 3005–3018 RSC.
- V. Brabec and O. Novakova, Drug Resist. Updates, 2006, 9, 111–122 CrossRef CAS PubMed.
- D. Wang and S. J. Lippard, Nat. Rev. Drug Discovery, 2005, 4, 307–320 CrossRef CAS PubMed.
- M. Apps, E. Choi and N. Wheate, Endocr.-Relat. Cancer, 2015, 22, 219–233 Search PubMed.
- A. Ruggiero, G. Trombatore, S. Triarico, R. Arena, P. Ferrara, M. Scalzone, F. Pierri and R. Riccardi, Anticancer Drugs, 2013, 24, 1007–1019 CrossRef CAS PubMed.
- T. C. Johnstone, G. Y. Park and S. J. Lippard, Anticancer Res., 2014, 34, 471–476 CAS.
- V. Brabec and J. Kasparkova, Drug Resist. Updates, 2005, 8, 131–146 CrossRef CAS PubMed.
- K. Jomova and M. Valko, Toxicology, 2011, 283, 65–87 CrossRef CAS PubMed.
- M. Valko, H. Morris and M. T. D. Cronin, Curr. Med. Chem., 2005, 12, 1161–1208 CrossRef CAS PubMed.
- M. Valko, C. J. Rhodes, J. Moncol, M. Izakovic and M. Mazur, Chem.-Biol. Interact., 2006, 160, 1–40 CrossRef CAS PubMed.
- M. Nagai, N. H. Vo, L. Shin Ogawa, D. Chimmanamada, T. Inoue, J. Chu, B. C. Beaudette-Zlatanova, R. Lu, R. K. Blackman, J. Barsoum, K. Koya and Y. Wada, Free Radical Biol. Med., 2012, 52, 2142–2150 CrossRef CAS PubMed.
- A. Gupte and R. J. Mumper, Cancer Treat. Rev., 2009, 35, 32–46 CrossRef CAS PubMed.
- D. Denoyer, S. A. S. Clatworthy, S. Masaldan, P. M. Meggyesy and M. A. Cater, Prostate, 2015, 75, 1510–1517 CrossRef CAS PubMed.
- D. Denoyer, S. Masaldan, S. La Fontaine and M. A. Cater, Metallomics, 2015, 7, 1459–1476 RSC.
- X. Ding, H. Xie and Y. J. Kang, J. Nutr. Biochem., 2011, 22, 301–310 CrossRef CAS PubMed.
- A. Gómez-Zavaglia, A. Ismael, L. I. L. Cabral, A. Kaczor, J. A. Paixão, R. Fausto and M. L. S. Cristiano, J. Mol. Struct., 2011, 1003, 103–110 CrossRef.
- A. Ismael, J. A. Paixão, R. Fausto and M. L. S. Cristiano, J. Mol. Struct., 2012, 1023, 128–142 CrossRef CAS.
- A. Ismael, A. Borba, L. Duarte, B. M. Giuliano, A. Gómez-Zavaglia and M. L. S. Cristiano, J. Mol. Struct., 2012, 1025, 105–116 CrossRef CAS.
- L. M. T. T. Frija, R. Fausto, R. M. S. S. Loureiro and M. L. S. Cristiano, J. Mol. Catal. A: Chem., 2009, 305, 142–146 CrossRef CAS.
- Bruker, APEX2, SAINT and SADABS, Bruker AXS Inc., Madison, Wisconsin, USA, 2006 Search PubMed.
- G. M. Sheldrick, Acta Crystallogr., Sect. A: Found. Adv., 2015, 71, 3–8 CrossRef PubMed.
- G. M. Sheldrick, Acta Crystallogr., Sect. C: Struct. Chem., 2015, 71, 3–8 CrossRef PubMed.
- A. L. Spek, Acta Crystallogr., Sect. D: Biol. Crystallogr., 2009, 65, 148–155 CrossRef CAS PubMed.
- M. W. Schmidt, K. K. Baldridge, J. A. Boatz, S. T. Elbert, M. S. Gordon, J. H. Jensen, S. Koseki, N. Matsunaga, K. A. Nguyen, S. Su, T. L. Windus, M. Dupuis and J. A. Montgomery, J. Comput. Chem., 1993, 14, 1347–1363 CrossRef CAS.
- P. J. Hay and W. R. Wadt, J. Chem. Phys., 1985, 82, 270–283 CrossRef CAS.
- W. R. Wadt and P. J. Hay, J. Chem. Phys., 1985, 82, 284–298 CrossRef CAS.
- P. J. Hay and W. R. Wadt, J. Chem. Phys., 1985, 82, 299–310 CrossRef CAS.
- E. Wiberg, A. F. Holleman and N. Wiberg, Inorganic Chemistry, Academic Press, San Diego, 2001 Search PubMed.
- R. W. Schmid and C. N. Reilley, Anal. Chem., 1957, 29, 264–268 CrossRef CAS.
- C. Megías, E. Pastor-Cavada, C. Torres-Fuentes, J. Girón-Calle, M. Alaiz, R. Juan, J. Pastor and J. Vioque, Eur. Food Res. Technol., 2009, 230, 353–359 CrossRef.
- S. Hidaka, H. Nishimura, K. Nakajima and S. Y. Liu, J. Periodontal Res., 1996, 31, 408–413 CrossRef CAS PubMed.
- P. Carter, Anal. Biochem., 1971, 40, 450–458 CrossRef CAS PubMed.
- L. L. Stookey, Anal. Chem., 1970, 42, 779–781 CrossRef CAS.
- A. Egusa Saiga and T. Nishimura, Biosci., Biotechnol., Biochem., 2013, 77, 2201–2204 CrossRef PubMed.
- P. Taylor, H. M. N. H. Irving, G. Iwantscheff and W. Germany, CRC Crit. Rev. Anal. Chem., 2008, 37–41 CAS.
- J. Su, Y.-Q. Sun, F.-J. Huo, Y.-T. Yang and C.-X. Yin, Analyst, 2010, 135, 2918–2923 RSC.
- W. Stavropoulos, B. Thiegs and R. Mack, US Pat., US3938954 A, 1973.
- F. H. Pollard and J. V. Martin, Analyst, 1956, 81, 348 RSC.
- T. Mosmann, J. Immunol. Methods, 1983, 65, 55–63 CrossRef CAS PubMed.
- R. R. Holmes, Progress in Inorganic Chemistry, John Wiley & Sons, Inc., Hoboken, NJ, USA, 1984, vol. 32 Search PubMed.
- E. L. Muetterties and L. J. Guggenberger, J. Am. Chem. Soc., 1974, 96, 1748–1756 CrossRef CAS.
- A. W. Addison, T. N. Rao, J. Reedijk, J. van Rijn and G. C. Verschoor, J. Chem. Soc., Dalton Trans., 1984, 1349–1356 RSC.
- N. J. Rose and R. S. Drago, J. Am. Chem. Soc., 1959, 81, 6138–6141 CrossRef CAS.
- M. M. Ayad, J. Phys.
Chem., 1994, 187, 123–133 CAS.
- H. A. Benesi and J. H. Hildebrand, J. Am. Chem. Soc., 1949, 71, 2703–2707 CrossRef CAS.
- A. V. Zubkov and T. V. Ivanova, J. Solution Chem., 1982, 11, 699–717 CrossRef CAS.
- S. Baniyaghoob, M. M. Najafpour and D. M. Boghaei, Spectrochim. Acta, Part A, 2010, 75, 970–977 CrossRef PubMed.
- C. R. Gibbs, Anal. Chem., 1976, 48, 1197–1201 CrossRef CAS.
- N. Endo, K. Nishiyama, M. Okabe, M. Matsumoto, H. Kanouchi and T. Oka, Biochim. Biophys. Acta, 2007, 1770, 571–577 CrossRef CAS PubMed.
- S. Habtemariam and E. Dagne, Food Chem. Toxicol., 2009, 47, 1490–1494 CrossRef CAS PubMed.
- A. Rivero-Müller, A. De Vizcaya-Ruiz, N. Plant, L. Ruiz and M. Dobrota, Chem.-Biol. Interact., 2007, 165, 189–199 CrossRef PubMed.
- C. Oviedo and J. Rodríguez, Quim. Nova, 2003, 26, 901–905 CrossRef CAS.
- P. Allain, Y. Mauras, A. Premel-Cabic, S. Islam, J. P. Herve and J. Cledes, Br. J. Clin. Pharmacol., 1991, 31, 347–349 CrossRef CAS PubMed.
- K. L. Cheng, K. Ueno and T. Imamura, CRC Handbook of Organic Analytical Reagents, CRC Press, 2nd edn, 1992 Search PubMed.
Footnote |
† Electronic supplementary information (ESI) available. CCDC 1443606. For ESI and crystallographic data in CIF or other electronic format see DOI: 10.1039/c6ra15051a |
|
This journal is © The Royal Society of Chemistry 2016 |