DOI:
10.1039/C6RA14894H
(Paper)
RSC Adv., 2016,
6, 92092-92103
Syntheses, structures and catalytic properties of organic–inorganic hybrid materials constructed from Evans–Showell-type polyoxometalates and zinc–organic coordination units†
Received
8th June 2016
, Accepted 13th September 2016
First published on 13th September 2016
Abstract
Four new organic–inorganic hybrid compounds based on the polyoxoanion [Co2Mo10H4O38]6−, namely [Zn2(H2O)5(4,4′-bipy)3]H2[Co2Mo10H4O38]·5H2O 1, [Zn2(H2O)2(bpe)3]H2[Co2Mo10H4O38] 2, [Zn(H2O)4(H2bpp)2][Co2Mo10H4O38]·2H2O 3 and [Zn3(Trz)6(H2O)6][Co2Mo10O38H6]·12H2O 4 (4,4′-bipyridine = 4,4′-bipy, 1,2-bis(4-pyridyl)ethane = bpe, 1,3-di(4-pyridyl)propane = bpp, 1,2,4-triazole = Trz), have been synthesized and characterized by elemental analysis, IR spectroscopy, TG analysis, solid diffuse reflective spectroscopy, powder X-ray diffraction and single crystal X-ray diffraction. Crystal analysis reveals that compound 1 exhibits a 1D staggered ladder-like chain constructed from [Co2Mo10H4O38]6− and zinc-4,4′-bipy complexes. Compound 2 shows a 1D zig-zag polymeric chain by the linkage of zinc–bpe complexes and [Co2Mo10H4O38]6−. In compound 3, [Co2Mo10H4O38]6− units are linked by zinc ions to form a 1D inorganic chain, protonated H2bpp molecules can be connected together by directional hydrogen-bonding interactions to construct a 3D supramolecular framework. Compound 4 consists of linear trinuclear zinc units bridging six Trz ligands and a [Co2Mo10H4O38]6− unit as the counter anion, extensive hydrogen bonds exist in the clusters forming a 3D supramolecular framework. Compounds 1–2 represent the first extended organic–inorganic hybrid structures based on Evans–Showell-type POMs. These compounds act as heterogeneous Lewis acid catalysts not only for the cyanosilylation of different carbonyl compounds with trimethylsilyl cyanide but also for recovery and reuse without displaying any significant loss of activity.
Introduction
Polyoxometalates (POMs), as one of the most widely used inorganic components, not only exhibit versatile structures, but also have a wide range of potential applications in catalysis, electrical conductivity, photochemistry, medicine and magnetism.1–8 POMs as versatile inorganic building blocks can be combined with different metal–organic units to construct organic–inorganic hybrid compounds. Such hybrid compounds can provide new structural motifs and serve as excellent candidates for catalytic studies.9–11 Therefore, much attention has been paid to the design and development of functional POM-based hybrids using different transition metal complexes (TMCs) and organic ligands.12–19 The TMCs can play several roles: (1) charge-compensating coordinated units, (2) covalently bound subunits of the POM framework itself and (3) bridging ligands linking polyanions into infinite extended networks. Many chemists have been focusing on choosing various TMCs to extend POM chemistry.20–26 So far, lots of POM-based TMCs with novel structures and various properties have been obtained, most of which rest on classical polyoxoanions, such as Keggin, Wells–Dawson and isopolymolybdate-type polyoxoanions.27–31 Examples of the organic–inorganic hybrids built from Evans–Showell-type POM [Co2Mo10H4O38]6− and transition metal complexes are still rare. There is only one literature that reported some new hybrid compounds based on [Co2Mo10H4O38]6− polyoxoanion, (4-H2pya)4[M(H2O)6][Co2Mo10H4O38]·5H2O (M = Co, Ni, Cu, Zn, Cd).32 The Evans–Showell-type POM [Co2Mo10H4O38]6−, as an important member of POM family, similar to Anderson POMs,33–35 not only owns two terminal oxygen atoms on each molybdenum center which makes it have strong coordination ability to transition metal cations forming new inorganic architectures, but also possess highly catalytic properties in organic oxidation.36 Thus, the [Co2Mo10H4O38]6− anions can be used as ideal building blocks to construct versatile organic–inorganic hybrid compounds.
Cyanosilylation of carbonyl compounds provides a convenient route to cyanohydrins, which are versatile intermediates in the synthesis of fine chemicals and pharmaceuticals.37,38 However, among many different types of POMs, there are very rare examples of cyanosilylation reactions catalyzing by POM-based TMCs up to now. Mizuno's group have synthesized a series of isostructural lacunary silicotungstate dimers with lanthanide cations, [{Ln(H2O)2(acetone)}2(γ-SiW10O36)2]10− (Ln = Y3+, Nd3+, Eu3+, Gd3+, Tb3+, or Dy3+) and effectively promoted cyanosilylation of carbonyl compounds with trimethylsilyl cyanide as homogeneous catalysts.39 Niu and coworkers have successfully isolated one [H2W11O38]8−-based metal organic framework {[Cu2(bpy)(H2O)5.5]2[H2W11O38]·3H2O·0.5CH3CN},40 which can be used as a Lewis acid catalyst through a heterogeneous manner to drive cyanosilylation with good efficiency. The development of efficient heterogeneous catalysts for cyanosilylation of carbonyl compounds with trimethylsilyl cyanide is still a significant work in current research.
In this paper, we select similar organonitrogen species such as the rigid and/or flexible N-donor ligands [4,4′-bipyridine = 4,4′-bipy, 1,2-bis(4-pyridyl)ethane = bpe, 1,3-di(4-pyridyl)propane = bpp, 1,2,4-triazole = Trz] used for our synthetic strategy41–44 and further explore their different properties on the catalytic activity. The organic ligands used in the TMC decorated [Co2Mo10H4O38]6− through covalently bonded not only fine-tune the material properties but also bring about novel synergistic effects between inorganic clusters and organic segments.45 Zinc(II) ion is very flexible in adopting various coordination geometries in its complexes. It is more easily coordinated with various N-containing organic functionalities and oxygen donors located at the surface of the polyanions, which make it possible to adopt zigzag, T-type, and linear coordination geometries and so on. So zinc(II) ion is a good candidate for the assembly of POM-based organic–inorganic hybrid compounds.
On the basis of the aforementioned points, we have successfully synthesized four novel organic–inorganic hybrid frames [Zn2(H2O)5(4,4′-bipy)3]H2[Co2Mo10H4O38]·5H2O 1, [Zn2(H2O)2(bpe)3]H2[Co2Mo10H4O38]·6H2O 2, [Zn(H2O)4(H2bpp)2][Co2Mo10H4O38]·2H2O 3 and [Zn3(Trz)6(H2O)6][Co2Mo10O38H6]·12H2O 4 (4,4′-bipyridine (4,4′-bipy), 1,2-bis(4-pyridyl)ethane (bpe), 1,3-di(4-pyridyl)propane (bpp), 1,2,4-triazole (Trz)). In compound 1, each two rigid bipy molecules link four Zn ions into paralleled unbent Zn-bipy coordination chains, which are further covalently connected by two [Co2Mo10H4O38]6− clusters resulting in staggered parallel double chains. Compound 2 consists of one-dimensional polyanionic chain of [Co2Mo10H4O38]6− clusters through Zn–bpe–Zn linkages. Compound 3 shows a 1-D polyanionic chain structure through zinc ions bridging, with the organic ligands bpp dissociating around the chain. Compound 4 possesses [Co2Mo10H4O38]6− clusters and trinuclear zinc moieties which create a unique infinite 3D supramolecular framework. All four compounds as Lewis acid catalysts are very efficient in the cyanosilylation of carbonyl compounds, and the reactions can be carried out under solvent-free conditions using only a low loading of the catalysts, which can be recovered and reused without displaying any significant loss of activity.
Experimental section
Materials and measurements
All chemicals were commercially purchased and used without further purification. (NH4)6[Co2Mo10H4O38]·7H2O was synthesized according to the literature,46,47 and characterized by means of IR spectroscopy. Elemental analyses (C, H and N) were performed on a Perkin-Elmer 2400 CHN elemental analyzer; Co, Mo and Zn were analyzed on a PLASMA-SPEC (I) ICP atomic emission spectrometer. IR spectra were recorded in the range 400–4000 cm−1 on an Alpha Centaur FT/IR Spectrophotometer using KBr pellets. TG analyses were performed on a Perkin-Elmer TGA7 instrument in flowing N2 with a heating rate of 10 °C min−1. PXRD patterns of the samples were recorded on a Rigaku Dmax 2000 X-ray diffractometer with graphite monochromatized Cu-Kα radiation (λ = 0.154 nm) and 2θ varying from 5 to 50°. UV-vis spectra were acquired on an Analytik Jena SPECORD S600 spectrophotometer equipped with a diode-array detector and an immersible fiber-optic probe. The GC analyses were performed on Techcomp GC-7900 with a flame ionization detector (FID) equipped with a SE-54 (internal diameter = 0.32 mm, length = 30 m).
Synthesis of [Zn2(H2O)5(4,4′-bipy)3]H2[Co2Mo10H4O38]·5H2O (1)
In a typical synthesis procedure for 1, Zn(NO3)2·6H2O (0.0297 g, 0.1 mmol) and (NH4)6[Co2Mo10H4O38]·7H2O (0.096 g, 0.05 mmol) were dissolved in 5 mL water. Then 5 mL alcohol which dissolved bipy (0.0156 g, 0.1 mmol) was added to the above solution. The mixture was heated at 80 °C for 6 h and dark green plate-shaped crystals of 1 were collected in about 39% yield (based on Mo). Elemental analyses: Mo, 38.62; Co, 4.82; Zn, 5.11; C, 14.39; N, 3.29; H, 1.89 (%). Found: Mo, 38.87; Co, 4.78; Zn, 5.26; C, 14.57; N, 3.40; H, 2.02 (%). FTIR data (cm−1): 3307(s), 3233(s), 3064(m), 1637(s), 1607(m), 1507(s), 1243(w), 1206(w), 942(vs), 905(vs), 747(vs), 699(vs), 636(vs), 604(vs) and 514(m).
Synthesis of [Zn2(H2O)2(bpe)3]H2[Co2Mo10H4O38]·6H2O (2)
The preparation of 2 was similar to that of 1 except that bpe (0.0184 g, 0.1 mmol) was used instead of bipy. Crystals of compound 2 were obtained in about 31% yield (based on Mo). Elemental analyses: Mo, 38.21; Co, 4.73; Zn, 5.12; C, 17.06; N, 3.47; H, 2.19 (%). Found: Mo, 38.10; Co, 4.68; Zn, 5.19; C, 17.15; N, 3.33; H, 2.30 (%). FTIR data (cm−1): 3392(vs), 3091(s), 2098(w), 1617(s), 1554(m), 1518(m), 1470(m), 1417(m), 1221(s), 1180(m), 1069(w), 942(vs), 905(vs), 878(vs), 837(s), 810(s), 689(vs), 631(vs), 605(vs) and 520(s).
Synthesis of [Zn(H2O)4(H2bpp)2][Co2Mo10H4O38]·2H2O (3)
The preparation of 3 was similar to that of 1 except that bpp (0.02 g, 0.1 mmol) was used instead of bipy. Crystals of compound 3 were obtained in about 46% yield (based on Mo). Elemental analyses: Mo, 42.39; Co, 5.14; Zn, 2.95; C, 13.68; N, 2.45; H, 2.25 (%). Found: Mo, 42.40; Co, 5.21; Zn, 2.89; C, 13.79; N, 2.47; H, 2.12 (%). FTIR data (cm−1): 3471(s), 3091(m), 1616(s), 1556(w), 1506(m), 1431(m), 1390(w), 1280(w), 1221(m), 1069(m), 1026(m), 947(vs), 910(vs), 842(vs), 699(vs), 641(vs), 599(vs), 557(vs) and 519(s).
Synthesis of [Zn3(Trz)6(H2O)6][Co2Mo10O38H6]·12H2O (4)
The preparation of 4 was similar to that of 1 except that Trz (0.0069 g, 0.1 mmol) was used instead of bipy. Crystals of compound 4 were obtained in about 46% yield (based on Mo). Elemental analyses: Mo, 36.65; Co, 4.57; Zn, 7.59; C, 5.62; N, 9.75; H, 2.16 (%). Found: Mo, 36.54; Co, 4.49; Zn, 7.47; C, 5.49; N, 9.60; H, 2.28 (%). FTIR data (cm−1): 3413(s), 3112(s), 2991(s), 2890(s), 1623(m), 1528(s), 1423(m), 1301(s), 1216(w), 1174(m), 1148(w), 1095(m), 1058(m), 932(vs), 869(s), 689(vs), 662(vs), 631(vs) and 562(s).
X-ray crystallography
The crystallographic data of four compounds were collected on the Bruker Smart CCD diffractometer with Mo Kα radiation (λ = 0.71073 Å) by ω and θ scan modes. Empirical absorption correction was applied. The structures of 1–4 were solved by the direct method and refined by the full-matrix least squares on F2 using the SHELXTL-97 software.48 All of the non-hydrogen atoms were refined anisotropically in 1–4. All of the non-hydrogen atoms except for partial crystal water molecules were refined anisotropically in 1–4. The hydrogen atoms attached to water molecules were not located in 1–4. Positions of the hydrogen atoms attached to carbon atoms and those attached to nitrogen atoms were fixed in ideal positions in 1–4. The detailed crystal data and structure refinements for 1–4 are given in Table 1. Notably, compound 4 is in the form of sheet, the diffraction spots obtained from one of its diffractive surface are fewer. Owing to the poor crystal quality, R1 (all data) value of 4 is high. Selected bond lengths and angles of 1–4 are respectively listed in Table 2. Crystallographic data for the structures reported in this paper have been deposited in the Cambridge Crystallographic Data Center with CCDC reference number: 1449669 for 1, 1449670 for 2, 1449671 for 3, 1449672 for 4.†
Table 1 Crystal data and structure refinement for 1–4
Complex |
1 |
2 |
3 |
4 |
R1 = ∑||F0| − |FC||/∑|F0|. wR2 = ∑[w(F02 − FC2)2]/∑[w(F02)2]1/2. |
Formula |
[Zn2(H2O)5(4,4′-bipy)3]H2[Co2Mo10H4O38]·5H2O |
[Zn2(H2O)2(bpe)3]H2[Co2Mo10H4O38]·6H2O |
[Zn(H2O)4(H2bpp)2][Co2Mo10H4O38]·2H2O |
[Zn3(Trz)6(H2O)6][Co2Mo10O38H6]·12H2O |
Formula weight |
2470.80 |
2518.88 |
2263.31 |
2626.15 |
T (K) |
293(2) |
296(2) |
296(2) |
273(2) |
Crystal system |
Triclinic |
Monoclinic |
Monoclinic |
Monoclinic |
Space group |
P![[1 with combining macron]](https://www.rsc.org/images/entities/char_0031_0304.gif) |
C2/c |
P21/n |
C2/c |
a (Å) |
11.4998(2) |
23.2424(17) |
18.9648(16) |
15.368(4) |
b (Å) |
13.7588(3) |
15.4023(12) |
12.2090(10) |
16.952(4) |
c (Å) |
22.6844(5) |
22.1388(15) |
26.576(2) |
25.834(7) |
α (°) |
73.4340(10) |
90.00 |
90.00 |
90.00 |
β (°) |
80.4120(10) |
115.004(4) |
107.8990(10) |
99.678(3) |
γ (°) |
65.5150(10) |
90.00 |
90.00 |
90.00 |
U (Å3) |
3126.02(11) |
7182.6(9) |
5855.6(9) |
6635(3) |
Z |
2 |
4 |
4 |
4 |
μ (mm−1) |
3.314 |
2.885 |
3.123 |
3.494 |
Reflections collected |
18 574 |
25 341 |
31 741 |
16 787 |
Independent reflections |
10 957 |
8341 |
13 407 |
5833 |
R(int) |
0.0246 |
0.0476 |
0.0375 |
0.0416 |
GOF on F2 |
1.022 |
1.036 |
0.983 |
1.199 |
R1a [I > 2σ(I)] |
0.0270 |
0.0416 |
0.0374 |
0.0702 |
wR2b [I > 2σ(I)] |
0.0682 |
0.1037 |
0.0879 |
0.1460 |
R1 (all data) |
0.0336 |
0.0644 |
0.0587 |
0.0831 |
wR2 (all data) |
0.0721 |
0.1159 |
0.1004 |
0.1510 |
Table 2 Selected bond lengths (Å) and angles (°) for 1–4
Symmetry transformations used to generate equivalent atoms: #1 −x, −y, −z #3 −x − 1/4, −y − 1/4, z. Symmetry transformations used to generate equivalent atoms: #1 −x + 2, −y, −z + 1 #4 −x + 2, y + 1/2, −z + 3/2. Symmetry transformations used to generate equivalent atoms: #1 −x−1, −y + 2, −z + 1 #2 −x, −y + 1, −z + 1. |
Compound 1 |
Mo(1)–O(23) |
1.707(3) |
Mo(1)–O(1) |
1.711(3) |
Mo(1)–O(18) |
1.932(3) |
Mo(2)–O(4) |
1.904(3) |
Mo(2)–O(28) |
2.290(3) |
Mo(3)–O(25) |
1.703(3) |
Mo(2)–O(8) |
2.277(3) |
Mo(3)–O(4) |
1.960(3) |
Mo(4)–O(9) |
1.728(3) |
Mo(5)–O(21) |
1.707(3) |
Co(1)–O(5) |
1.866(3) |
Co(2)–O(16) |
1.901(3) |
Zn(1)–O(2W) |
2.081(3) |
Zn(1)–N(1) |
2.099(4) |
O(26)–Co(1)–O(5) |
83.60(12) |
O(5)–Co(1)–O(8) |
87.44(12) |
O(1)–Mo(1)–O(18) |
101.77(13) |
O(10)–Mo(3)–O(4) |
148.80(12) |
![[thin space (1/6-em)]](https://www.rsc.org/images/entities/char_2009.gif) |
Compound 2a |
Mo(1)–O(2) |
1.691(4) |
Mo(2)–O(8) |
1.730(4) |
Mo(2)–O(14) |
1.979(3) |
Mo(3)–O(5) |
1.734(4) |
Mo(4)–O(1) |
1.698(4) |
Co(1)–O(10) |
1.883(4) |
Co(2)–O(13)#1 |
1.866(3) |
Zn(1)–N(3)#3 |
2.088(4) |
O(2)–Mo(1)–O(16) |
97.33(19) |
O(10)–Co(1)–O(14) |
87.26(15) |
N(3)–Zn(1)–N(1)#1 |
98.69(19) |
N(3)–Zn(1)–O(1W) |
95.30(18) |
![[thin space (1/6-em)]](https://www.rsc.org/images/entities/char_2009.gif) |
Compound 3b |
Mo(1)–O(21) |
1.704(4) |
Mo(1)–O(14) |
1.724(4) |
Mo(2)–O(19) |
1.704(4) |
Mo(2)–O(13) |
1.710(4) |
Mo(3)–O(12) |
1.710(4) |
Mo(3)–O(10) |
1.906(4) |
Mo(3)–O(22) |
2.237(3) |
Mo(4)–O(16) |
1.720(4) |
Mo(5)–O(9) |
1.712(4) |
Mo(6)–O(6)#4 |
1.716(4) |
Co(1)–O(15) |
1.862(3) |
Co(1)–O(5) |
1.910(3) |
Zn(1)–O(1W)#1 |
2.099(4) |
|
|
O(12)–Mo(3)–O(8) |
105.5(2) |
O(15)–Co(1)–O(5)#1 |
87.50(15) |
O(1W)–Zn(1)–O(14) |
94.78(16) |
O(14)–Zn(1)–O(11) |
175.90(15) |
![[thin space (1/6-em)]](https://www.rsc.org/images/entities/char_2009.gif) |
Compound 4c |
Mo(1)–O(10) |
1.709(9) |
Mo(1)–O(18) |
2.300(2) |
Mo(1)–O(7) |
2.001(9) |
Mo(2)–O(6) |
1.692(10) |
Mo(3)–O(12) |
2.267(9) |
Mo(3)–O(9)#1 |
1.691(9) |
Mo(5)–O(14) |
1.923(9) |
Co(1)–O(2)#1 |
1.874(8) |
Zn(2)–N(5) |
2.197(10) |
Zn(1)–O(3W) |
2.142(9) |
Zn(1)–N(4)#2 |
2.109(11) |
Zn(1)–O(1W) |
2.159(9) |
O(10)–Mo(1)–O(7) |
95.7(4) |
O(2)–Co(1)–O(7)#1 |
87.1(2) |
N(4)–Zn(1)–N(1)#1 |
90.5(4) |
N(4)–Zn(1)–O(1W) |
175.2(4) |
Cyanosilylation
The detailed reaction conditions are shown in the captions of Table 3. A typical procedure for cyanosilylation of carbonyl compounds is as follows: a solution of an aldehyde (0.5 mmol) and trimethylsilyl cyanide (1.5 mmol) is stirred for 5 min and then catalyst 10 mg (2 mol%) is added, the reaction mixtures were stirred at room temperature under a N2 atmosphere, the conversion of benzaldehyde and the product yield were periodically determined by gas chromatography (GC) analysis. After the reaction was completed, the catalyst was removed by filtration and centrifugation of the reaction mixture. All products (cyanohydrin trimethylsilyl ethers) were confirmed by a comparison of their GC retention times, GC-MS spectra, and/or 1H spectra with those of authentic data. GC analysis was performed using HP6890/5973MS with a cross-linked (95%)-dimethyl-(5%)-diphenylpolysiloxane column of 30 m length.
Table 3 Cyanosilylation of aldehydes catalyzed by compounds 1–4a
Results and discussion
Synthesis
The reactions of (NH4)6[Co2Mo10H4O38]·7H2O, Zn(NO3)2·6H2O and four different ligands have been investigated. Compounds 1–4 were synthesized under the same reaction conditions (the same Zn/[Co2Mo10H4O38]6−/ligand ratio (2
:
1
:
2), same temperature, same concentration of starting polyoxometalate and same pH) by the conventional solution method which possess reasonably good solubility and are environmental-friendly. In fact, many factors such as stoichiometry, solvent, reaction temperature, pH value, metallic salt and ligand can significantly affect the final structures of POM-based hybrid compounds. Many parallel experiments show that the solvent was the important factor in the formation of target compounds. When using water as single solvent, unfortunately, we could only obtain unidentified sediment rather than crystals. Moreover, when we choose acetonitrile, DMF or methanol, respectively, to mix with water, we also could not obtain the crystals. However, when we choose a mixture of water and alcohol as the reaction solvent, the title compounds 1–4 with different structures were obtained. In order to explore the role of alcohol on the synthesis of the title compounds, we had chosen different ratios of C2H5OH/H2O mixed solvents. However, when we used alcohol as a single solvent at the process of the reaction, we could only get some unidentified sediments. Only when we used different ratios of C2H5OH/H2O mixed solvents, we were able to obtain compounds 1–4. Furthermore, some attempts of using different ratios such as 2
:
1, 3
:
1, and 4
:
1 of the metal cations to the organic ligands were made, and we found that a higher ratio was inclined to obtain a higher yield of the title compounds, but the crystal qualities of compounds 1–4 were slightly poor. The optimal pH range of the reaction is from 3.8 to 4.1. In a low pH value (pH < 3.0), no reaction occurs and the colors of the solution turn to dark; while the hydrolysis of the zinc cations makes a mass of precipitates rather than the crystals in a high pH value (pH > 3.5). The optimal reaction temperature is about 80 °C. At higher temperature (such as 120 °C), crystals with poor quality can be obtained; at lower temperature (such as 25 °C), no crystals were observed. We use the zinc ion as the metallic linker because of it can exhibit versatile coordination modes in the construction of interesting structural motifs.49 When Zn(NO3)2·6H2O was replaced by another ZnII salts, such as ZnCl2, ZnSO4·7H2O or Zn(CH3COOH)2·2H2O, compounds 1–4 could not be afforded, implying that Zn(NO3)2·6H2O may serve as an additive or structural directing agent for the formation of 1–4. In summary, the solvent and the nature of the metal salt play important roles in the crystallization of 1–4.
Organic ligand effects
In these experiments, the 4,4′-bipy, bpe, bpp and Trz are picked out as the nitrogen donor ligands to assemble organic–inorganic hybrid structures based on the following considerations: the terminal N sites in the four molecules are advantageous for protonation to form N–H⋯O interactions with anions. The bpp molecules are usually regarded as excellent candidates for the construction of hybrid solids due to their flexible geometries.41 Compared with the flexible bpp, the rigid 4,4′-bipy and bpe can also produce unique structures with useful functional properties.42 In compounds 1–2, the short lengths of ligands make them possible provide two N atoms for connecting the zinc ion to another. The linear 4,4′-bipy and bpe ligands are functioning as bridging groups and directly bonding to the backbone of [Co2Mo10H4O38]6−. The length of the Zn–bpe (11.352 Å) is longer than that of Zn–bipy (9.183 Å), this will certainly lead to their structural differences. The large volumes of the clusters probably hinder compounds 1–2 to form higher dimensions. The flexible bpp ligands of compound 3 do not participate in the composition of the structure. Although the three ligands possess the same type of two-terminal donor N atoms, their final structures are different. In compound 4, the different coordination modes of Zn(1) and Zn(2) connect to each other by Trz forming a dissociative tripolymer. Trz ligands are observed to be in the chelating bidentate modes bonding to zinc metals and [Co2Mo10H4O38]6− clusters as an isolated charge-balancing anion. These results indicated that the different lengths and geometries of the ligands used in the four-reaction systems play important roles in the formation of the structures. Compared with previously reported (4-H2pya)4[M(H2O)6][Co2Mo10H4O38]·5H2O (M = Co, Ni, Cu, Zn, Cd)32 which possess same configuration, compounds 1–4 exhibit various coordination modes due to different ligands. If the ligands were properly controlled, inorganic oxide units and organic ligands could produce interesting compounds with distinctive frameworks. According to reported [Zn(en)2(H2O)][Zn(en)2(4,4′-bipy)Zn2As8V12O40(H2O)]·3H2O and [Zn2(en)5]{[Zn(en)2][(bpe)HZn2As8V12O40(H2O)]2}·7H2O (en = ethylenediamine),41 the different POMs also lead to structural differences, this is because the various coordination abilities of POMs.
Crystal structure of 1
X-ray crystallography analysis reveals that compound 1 crystallizes in triclinic P
space group and exhibits 1D double chains through the connecting of 4,4′-bipy. As shown in Fig. 1a, the asymmetric unit of 1 contains two zinc(II) ions, one [Co2Mo10H4O38]6− unit, three 4,4′-bipy ligands and six coordinated water molecules. In the [Co2Mo10H4O38]6− anion, the two Co atoms lie in the centre of the polyanion connected by two μ3-O atoms, while the ten octahedrally coordinated Mo atoms distribute around the Co2 cluster forming the [Co2Mo10H4O38]6− unit. The basic building unit [Co2Mo10H4O38]6− can be deduced from the planar Anderson ion [CoMo6H6O24]3− by removing one {MoO5} group from each of two [CoMo6H6O24]3− ions, turning one ∼45° around the anionic equatorial plane and joining them so that the two CoO6 octahedra share an edge. In the polyoxoanion, two unique oxygen atoms surrounding each Co3+ ion, are bound to hydrogen atoms, which are identified by the calculation of bond valence sums.50 Six kinds of oxygen atoms exist in the cluster according to the manner of oxygen coordination, that is terminal oxygen Ot, terminal oxygen Ot′ linked to Zn2+, double-bridging oxygen Ob, central oxygen Oc (μ3-OH atom of a Co and two Mo atoms), central oxygen Od (μ4-O atom of a Co and three Mo atoms), central oxygen Oq (μ4-O atom of two Co and two Mo atoms). Thus the Mo–O bond lengths fall into six classes: Mo–Ot 1.680(3)–1.724(3) Å, Mo–Ot′ 1.725(3)–1.728(3) Å, Mo–Ob 1.872(3)–1.953(3), Mo–Oc 2.250(3)–2.322(3) Å, Mo–Od 1.966(3)–2.369(3) Å and Mo–Oq 2.249(3)–2.278(3) Å in 1. The central Co–O distances are 1.867(3)–1.945(3) Å, and the O–Co–O angles are in the range of 83.6(2)–175.7(2)°. All bond lengths and bond angles are within the normal ranges.46,47 Both of the two Zn(II) ions exhibit the six-coordinated octahedral geometry, but have two distinct coordination environments. Zn1 is ligated by two nitrogen atoms from two 4,4′-bipy ligands, one oxygen atoms from [Co2Mo10H4O38]6− unit, and three coordinated water molecules, while Zn2 is coordinated to two nitrogen atoms from two 4,4′-bipy ligands, two coordinated water molecules and two oxygen atoms from one [Co2Mo10H4O38]6− unit. It is noteworthy that the two Zn2 ions are directly connecting to the adjacent [Co2Mo10H4O38]6− anions by two terminal oxygen (Ot) atoms of the two {MoO6} octahedron, and two 4,4′-bipy ligands act as bridges connected to adjacent Zn1 and Zn2 centers from the neighboring clusters into 1D infinite chains (Fig. 1c). The bond distances of Zn–N are 2.099(4)–2.124(4) Å, while the N–Zn–N angles are 92.06(14)–96.24(15)°. Each chain is further connected to other two parallel chains by hydrogen bonds between water molecules and the terminal oxygen atoms of the heteropolyanions to yield a pseudo-2D layer, then through the linkages of π–π stacking interactions and hydrogen bond contacts, extended 3D supramolecular network are formed (Fig. 1d). It is also striking that the structure of the title compound exhibits extensive hydrogen-bonding interactions among water molecules, 4,4′-bipy molecules, and polyoxoanions. Hydrogen bond distances are O34⋯O1W 2.803, O37⋯N6 2.928, O18⋯N2 2.672 Å. It is believed that the extensive hydrogen-bonding interactions play an important role in stabilizing the 3D framework.
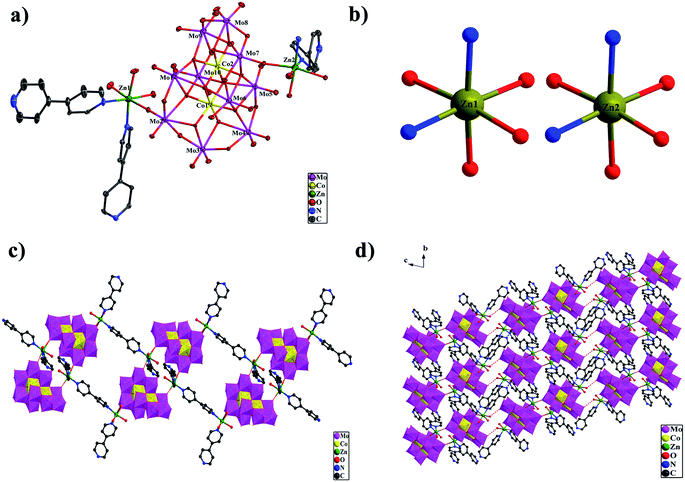 |
| Fig. 1 (a) ORTEP drawing of 1 with thermal ellipsoids at 50% probability. Free water molecules are omitted for clarity. (b) The coordination modes of Zn2+ cations in 1. (c) A view of the 1D chain in 1. (d) View of the 3D supramolecular framework of 1 along the c axis, (color code: Co, yellow; Mo, pink; Zn, green; O, red; N, blue; C, dark green; hydrogen bond, red). Free water molecules are omitted for clarity. | |
Crystal structure of 2
Compound 2 crystallizes in the monoclinic space group C2/c. In the asymmetric unit, there are half one crystallographically independent [Co2Mo10H4O38]6− polyanion, one zinc(II) ion, two bpe ligands and one coordinated water molecule (Fig. 2a). The [Co2Mo10H4O38]6− is a typical Evans–Showell type polyoxoanion. The Mo–O bond lengths also fall into six classes: Mo–Ot 1.692(4)–1.705(4) Å, Mo–Ot′ 1.707(4)–1.736(4) Å, Mo–Ob 1.884(4)–1.979(4) Å, Mo–Oc 2.255(3)–2.287(4) Å, Mo–Od 1.979(4)–2.324(4) Å and Mo–Oq 2.266(4)–2.328(4) Å in 2. The central Co–O distances are 1.865(3)–1.954(4) Å, and the O–Co–O angles are in the range of 85.2(2)–173.6(2)°. The Zn atom on the backbone of the cluster bonds to two N donor from the bpe ligands [Zn1–N1 = 2.105(2) Å and Zn1–N2 = 2.089(2) Å] and three μ3-O [Zn–O = 2.138(9)–2.315(8) Å] of [Co2Mo10H4O38]6− polyanion, together with one water molecules to furnish an octahedral coordination environment. The bond angles around the Zn(II) ion are 98.70(19) for N–Zn–N, 87.20(16)–174.10(17)° for O–Zn–N, 92.01(19)–95.32(18)° for OW–Zn–N, 82.22(14)–90.33(14)° for O–Zn–O. In 2, the bpe ligands exhibit two different coordination modes: one of them only conjugates with Zn atom by means of one N atom on one side, forming the metal–organic motif [Zn(bpe)]2+; the other serves as bridging ligand to link with two Zn atoms from the two neighboring clusters. The fragments [Zn(bpe)]2+ and the [Co2Mo10H4O38]6− polyanions are alternatively arranged in a 1D zigzag chain of [–{Zn2Co2Mo10H4O38}–bpe–{Zn2Co2Mo10H4O38}–]∞ arrays (Fig. 2c). In addition, the adjacent chains are linked together by other hydrogen-bonding interactions (N2⋯O16 2.669 Å in 2) to generate an interesting 2D supramolecular wavelike network. Furthermore, 2D supramolecular layers are connected to yield a 3D supramolecular framework via the strong hydrogen-bonding interactions (N2⋯O16 2.669 Å in 2) (Fig. 2d).
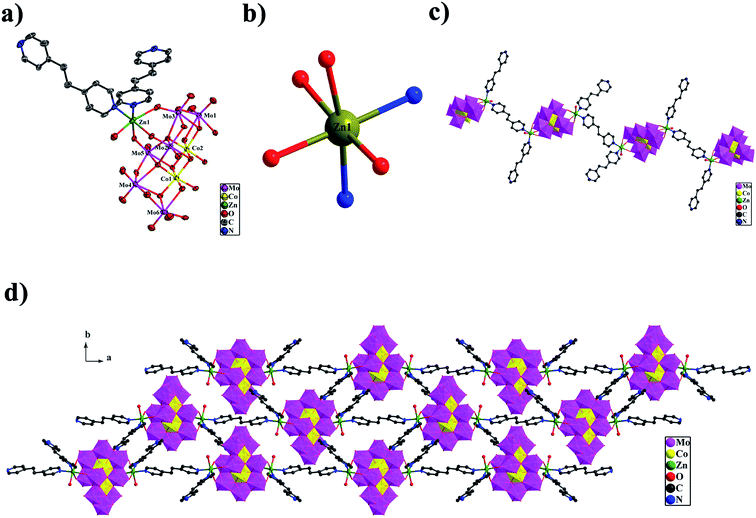 |
| Fig. 2 (a) ORTEP drawing of 2 with thermal ellipsoids at 50% probability. Free water molecules are omitted for clarity. (b) The coordination modes of Zn2+ cations in 2. (c) A view of the 1D chain in 2. (d) View of the 3D supramolecular framework of 2 along the c axis, (color code: Co, yellow; Mo, pink; Zn, green; O, red; N, blue; C, dark green; hydrogen bond, red). Free water molecules are omitted for clarity. | |
Crystal structure of 3
X-ray crystal structure analysis reveals that compound 3 crystallizes in the monoclinic space group P21/n. The asymmetric unit of compound 3 contains one zinc(II) ion, one [Co2Mo10H4O38]6− unit, two protonated H2bpp molecules together with four coordinated water molecules (Fig. 3a). There are six classes of Mo–O bond lengths: Mo–Ot 1.697(4)–1.715(4) Å, Mo–Ot′ 1.716(4)–1.725(4) Å, Mo–Ob 1.874(4)–1.967(4) Å, Mo–Oc 2.237(3)–2.304(3) Å, Mo–Od 1.964(4)–2.331(3) Å and Mo–Oq 2.291(4)–2.371(3) Å in 3. The central Co–O distances are 1.862(3)–1.953(3) Å, and the O–Co–O angles are in the range of 83.90(14)–176.57(15)°. The Zn atom is located in the [Zn(Co2Mo10)2] dimer and bridges two [Co2Mo10] units through two μ3-O atoms [O(11), O(14)] to generate a 1D chain and Zn–O bond lengths are ranging from 2.129(4) Å to 2.171(4) Å (Fig. 3c). The Zn atom exhibits a distorted octahedral ZnO6 coordination geometry, which is coordinated with two oxygen atoms from two different [Co2Mo10H4O38]6− units and four oxygen atoms [O(1W), O(2W), O(3W) and O(4W)] from coordinated water molecules with Zn–O distances of 2.099, 2.092, 2.061 and 2.049 Å, respectively. In the structure 3, the adjacent 1D chains are parallel with each other and connected by the bpp molecules via hydrogen-bonding interactions between the nitrogen atoms in the bpp and the oxygen atoms from [Co2Mo10H4O38]6− units (N4⋯O13 2.815 Å, N3⋯O33 2.794 Å) to form a 2D supramolecular layer. The adjacent layers are further linked together by strong hydrogen-bonding interaction between oxygen atoms of water molecules and surface O atoms of polyoxoanions to form a 3D supramolecular framework (O1W⋯O19 2.772 Å, O4W⋯O16 2.730 Å in 3) (Fig. 3d).
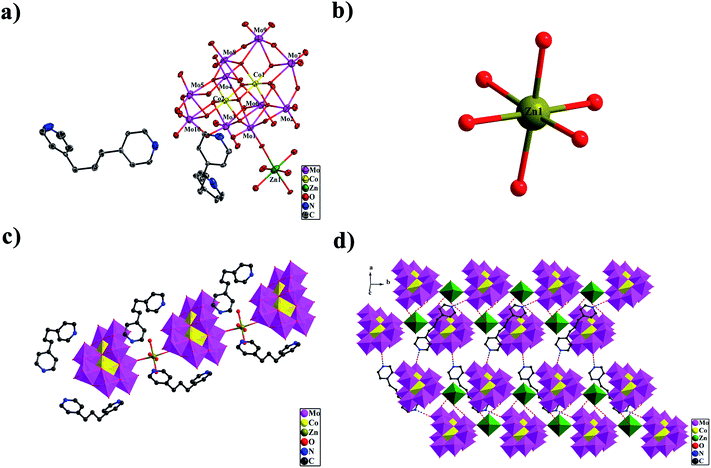 |
| Fig. 3 (a) ORTEP drawing of 3 with thermal ellipsoids at 50% probability. Free water molecules are omitted for clarity. (b) The coordination modes of Zn2+ cations in 3. (c) A view of the 1D chain in 3. (d) View of the 3D supramolecular framework of 3 along the c axis, (color code: Co, yellow; Mo, pink; Zn, green; O, red; N, blue; C, dark green; hydrogen bond, red). Free water molecules are omitted for clarity. | |
Crystal structure of 4
As shown in Fig. 4a, the asymmetric unit of compound 4 is composed of one [Co2Mo10H4O38]6− unit as anion, one trinuclear arrangement of [Zn3(Trz)6(H2O)6]6+ as cation to balance the whole charges, and twelve solvent water molecules in the crystal lattice. The trinuclear zinc fragment each with +6 charges is arranged in a linear geometry containing six triazole ligands and six coordinated water molecules within a unit. The three zinc ions link to each other by two pairs of three bridged triazole molecules as bidentate ligands via 1- and 2-positioned N atoms. All of the above zinc ions are six coordinated: the center zinc ion is surrounded by six N atoms to form disordered ZnN6 octahedron, while each terminal Zn(II) completes its octahedral geometry by three water molecules and three N atoms from triazole ligands. The Zn–N and Zn–O bond lengths are in normal ranges from 2.107(11) to 2.197(10) and 2.106(10) to 2.159(9) Å, respectively. The Mo–O bond lengths fall into five classes: Mo–Ot 1.691(9)–1.707(9) Å, Mo–Ob 1.918(9)–1.995(9) Å, Mo–Oc 2.250(9)–2.299(8) Å, Mo–Od 1.947(9)–2.365(9) Å and Mo–Oq 2.278(9)–2.353(8) Å in 4. The central Co–O distances are 1.874(8)–1.944(9) Å, and the O–Co–O angles are in the range of 84.1(4)–176.8(3)°. All bond lengths and bond angles are within the normal ranges.46,47
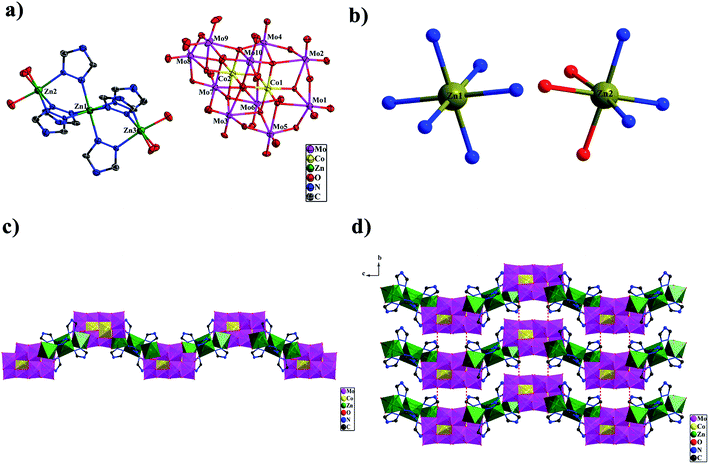 |
| Fig. 4 (a) ORTEP drawing of 4 with thermal ellipsoids at 50% probability. Free water molecules are omitted for clarity. (b) The coordination modes of Zn2+ cations in 4. (c) Polyhedron and ball-stick view of the 1D supramolecular chiral chain in 4 through the hydrogen-bonding interactions between the terminal oxygen atoms of polyoxoanions and coordinating water molecules. (d) View of the 3D supramolecular framework of 4 along the c axis, (color code: Co, yellow; Mo, pink; Zn, green; O, red; N, blue; C, dark green; hydrogen bond, red). Free water molecules are omitted for clarity. | |
It is notable that because of the high degree of hydration and abundant oxygen atoms of polyoxometalates, extensive hydrogen-bonding interactions exist in this structure. Each [Co2Mo10H4O38]6− unit is linked to the adjacent two trinuclear zinc arrangement ({Zn3}) and two other [Co2Mo10H4O38]6− units, through two N–H⋯O and two O–H⋯O bonds in a zigzag manner to generate a 2D supramolecular layer (Fig. 4c). The O⋯O and N⋯O distances in this framework vary from 2.633(6) to 2.945(6) Å and 2.605(7) to 2.847(7) Å, respectively. Furthermore, these 2D layers are joined together via hydrogen bonds (O9⋯O1W 2.761 Å) among oxygen atoms of coordination water molecules and terminal oxygen atoms to form a 3D supramolecular framework (Fig. 4d).
FT-IR spectra
IR spectra of compounds 1–4 display similar characteristic patterns of the Evans–Showell type structure [Co2Mo10H4O38]6− (Fig. S1a–d†), in agreement with those reported in literatures except for slight changes in peak position, perhaps due to coordination.46 In the spectra, the bands between 900 and 950 cm−1 are assignable to the Mo–Ot units; while between 500 and 750 cm−1 are for the Mo–Ob groups. Below 500 cm−1, the spectrum is rather difficult to analyze, the bending of the Mo–Ot and Mo–Ob bonds can be mixed with the Mo–Oc stretching vibrations. The bands at 1637, 1607, 1507 and 1206 cm−1 for 1 is corresponding to the characteristic absorptions of 4,4′-bipy ligand, while at 1617, 1554, 1417 and 1221 cm−1 for 2 is ascribed to the characteristic absorptions of bpe ligand. For 3, the characteristic absorptions of bpp ligand are located at 1616, 1556, 1506, 1431, 1069 and 1026 cm−1, and the characteristic absorption of triazole ligand in compound 4 are at 1623, 1528, 1301, 1148 and 1058 cm−1. In comparison with the uncoordinated POMs such as compounds 3–4, the Mo–O stretching vibrations of compounds 1–2 are shifted to low frequency due to the interaction of organonitrogen species, indicating the grafting of organic ligands onto the surface of POMs, which are in good agreement with the results of single-crystal X-ray structural analyses.
TG analysis
The TG curve of 1 exhibits a multi-step continuous weight loss process and gives a total weight loss of 45.9% in the range of 30–800 °C, which agrees with the calculated value of 46.7%. The weight loss of 7.5% at 70–266 °C corresponds to the loss of all lattice and composition water molecules (calc. 7.3%). The weight loss of 38.4% at 270–800 °C arises from the decomposition of bipy molecules and oxygen molecules from the residual metal oxides (calc. 39.3%).
TG curve of 2 exhibits two-step continuous weight loss process and gives a total weight loss of 51.3% in the range of 28–800 °C (calc. 50.2%). The weight loss of 10.9% from 28 to 350 °C corresponds to the loss of lattice water, composition water molecules and partial bpe molecules. The weight loss of 40.4% from 360 to 730 °C is due to the loss of bpe molecules and oxygen molecules from the residual metal oxides.
TG curve of 3 possesses a multi-step continuous weight loss process and gives a total loss of 49.6% in the range of 30–800 °C (calc. 48.5%). The weight loss of 3.2% from 30 to 140 °C arises from the loss of all lattice water molecules (calc. 2.9%). The weight loss of 3.6% at 190–225 °C corresponds to the loss of all coordinated and composition water molecules (calc. 3.9%). The continuous weight loss of 41.8% from 230 to 800 °C is due to the loss of bpp molecules and oxygen molecules from the residual metal oxides.
The TG curve of 4 also exhibits a multi-step continuous weight loss process and gives a total loss of 42.1% in the range of 30–900 °C (calc. 43.3%). The three-step continuous weight loss of 7.2% from 40 to 100 °C arises from the loss of all lattice, coordinated and composition water molecules (calc. 8.3%). The continuous weight loss of 34.9% from 100 to 800 °C is due to the loss of Trz molecules and oxygen molecules from the residual metal oxides.
Powder X-ray diffraction characterization
The PXRD patterns for 1–4 are presented in Fig. S3a–d.† The very good correspondence between the calculated and the experimental patterns suggest the high purities of these compounds. These conclusions are in agreement with the results of the single crystal X-ray analysis.
UV-vis diffuse reflective spectroscopy
The diffuse reflective spectra of compounds 1–4 are displayed in Fig. 5. On the UV region (200–400 nm), the plots display two intense absorption bands at 223 and 302 nm for 1, 220, 298 nm for 2, 227, 295 nm for 3, and 228, 296 nm for 4, which are corresponding to the characteristic of O → Mo charge transfer for POMs. On the visible region (400–800 nm), there are also two absorption bands at 442, 607 nm for 1, 445, 605 nm for 2, 441, 604 nm for 3 and 442, 603 nm for 4, which are respectively attributed to the 1A1g → 1T2g and 1A1g → 1T1g transition of a regular octahedral configuration low-spin Co3+ in the [Co2Mo10H4O38]6− anion.51
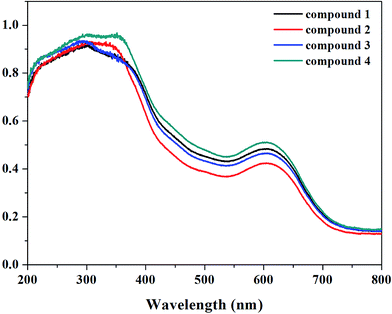 |
| Fig. 5 UV-vis diffuse reflectivity spectra of K–M functions vs. energy (eV) of compounds 1–4. | |
Cyanosilylation study
Cyanosilylation reaction provides a convenient route to cyanohydrins, which are key derivatives in the synthesis of fine chemicals and pharmaceuticals. In order to obtain cyanohydrin molecules, the use of a catalyst with Lewis acid or base which could necessarily activate both the substrate and the cyanide precursor.52 In this sense, the starting substrates commonly used are aldehydes or ketones with trimethylsilyl cyanide (TMSCN) as the nucleophilic agent. The catalytic properties of compounds 1–4 as heterogeneous catalysts in the aldehyde cyanosilylation reaction have been investigated under solvent-free condition at room temperature.
Firstly, the cyanosilylation of benzaldehyde acts as a model reaction to investigate the catalytic nature of compounds 1–4. The reaction was carried out with 2 mol% of catalysts under the conditions described in Table 3. All our compounds effectively catalyzed the cyanosilylation to afford the corresponding cyanohydrin trimethylsilyl ether with yield of up to about 90%. In a comparison of the activity of our catalysts with that of some previously reported POM-based compounds ([Cu2(bpy)(H2O)5.5]2[H2W11O38]18 and TBA8H2[(SiYW10O36)2]),40 it was found to be higher than the first one (benzaldehyde, 24 h, and yield = 98.1%), which uses solvent conditions, and less than the second one, which is a homogeneous catalyst and also uses solvent conditions (benzaldehyde, 15 min, and yield = 94%). Besides, compared with other catalysts used to promote the cyanosilylation reactions, such as Lewis acids,53 nonionic bases,54 N-heterocyclic carbenes55 and organic/inorganic salts,56 these compounds can be modified to contact with organic solvents due to coordination bonds between metallic clusters and organic ligands, as a consequence, the use of solvent-free conditions during reactions contribute to preserve structure, prevent the presence of heterogeneous active sites in the reaction. In addition, solvent-free meets concept which people advocate green synthesis and environmentally favorable. Most importantly, the yields in our experiments are higher than those of above mentioned compounds, and the reactions spend less time. Control experiments were also carried out, which suggested that the absence of the catalysts could hardly trigger the cyanosilylation of benzaldehyde to target product. When using the [Co2Mo10H4O38]6− unit or Zn mixed with ligands (bpe) to replace the catalysts 1–4, only low yields of product (56% or 42%) could be detected under the same reaction conditions.
Subsequently, the cyanosilylation reaction of various kinds of aromatic aldehyde substrates in the presence of catalyst 2 was further studied. With a substrate like 2-hydroxybenzaldehyde, 4-nitrobenzaldehyde, or 4-methylbenzaldehyde, the catalyst shows efficient activity to catalyze these cyanosilylation reactions with the high yields (Table 3). It seems that the nature of the substituent on the aromatic ring had no dramatic effect on the reaction yield. However, when using the large molecule 1-napthaldehyde as the substrate, the yield greatly decreases due to the steric hindrance and electron effects, it is suggested that the transition-state geometry of aldehyde here is not suitable for driving the reaction to the product according to the Lewis acid effect of transition metals.57
To probe the heterogeneity of the catalysts, after the catalytic reactions, the catalysts were recovered from their reaction media. Solid of 2 could be easily isolated from the reaction suspension by a simple filtration and reused at least three times without an appreciable loss of its high catalytic performance (from 99% to 93% yield see Table S1†). The PXRD patterns of the retrieved catalyst 2 were identical with those of the fresh catalyst (shown in Fig. 6). The IR spectrum of the recovered compound 2 was also identical to that of the freshly prepared sample (Fig. S4†). These observations suggested that 2 is a true heterogeneous catalyst for the cyanosilylation reaction. In addition, infrared spectroscopy of the catalyst 2 impregnated with benzaldehyde exhibited one broad C–O stretch at 1691 cm−1. The ν(C–O) stretch had a red shift of 11 cm−1 from 1702 cm−1 of the free benzaldehyde (Fig. S5†). This experiment unambiguously demonstrated the possible activation of the substrate by the Zn2+ cation as Lewis acid site in 2.
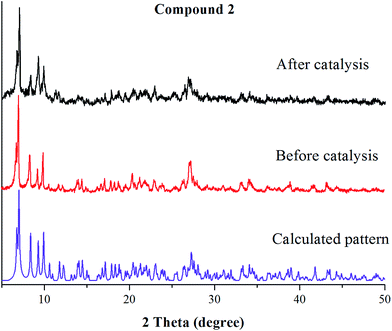 |
| Fig. 6 Powder X-ray diffraction (PXRD) patterns of 3: calculated pattern from crystal data (blue line); experimental pattern before catalysis (red line); recovered catalyst 3 after 3 catalytic runs of the cyanosilylation of benzaldehyde (black line). | |
Conclusions
In summary, four novel hybrids based on Evans–Showell-type POMs and zinc–organic units have been synthesized and characterized, which represent the second examples of organic–inorganic hybrid species constructed from Evans–Showell POMs and transition metal complexes. Compounds 1–2 represent the first extended hybrid structure based on Evans–Showell-type POMs. In compounds 1–4, hydrogen bonds and π⋯π interactions play important roles in constructing 3D crystal supramolecular frameworks. These new POM materials are efficient heterogeneous Lewis acid catalysts for cyanosilylation of various aldehyde compounds under solvent-free conditions. The successful isolation of these species certainly provokes researchers' interest to develop new heterogeneous catalytic materials containing Evans–Showell POMs and transition metal complexes.
Acknowledgements
The authors thank the National Natural Science Foundation of China (21371027, 21274015, 20901013), Natural Science Foundation of Liaoning Province (2015020232) and Fundamental Research Funds for the Central Universities (DUT15LK02, DUT15LN18) for financial support.
References
- A. Müller, F. Peters, M. T. Pope and D. Gatteschi, Chem. Rev., 1998, 98, 239 CrossRef.
- S. Uchida, M. Hashimoto and N. Mizuno, Angew. Chem., Int. Ed., 2002, 42, 2814 CrossRef.
- D. L. Long, E. Burkholder and L. Cronin, Chem. Soc. Rev., 2007, 36, 105 RSC.
- M. Li, C. Xu, J. S. Ren, E. B. Wang and X. G. Qu, Chem. Commun., 2013, 49, 11394 RSC.
- J. Song, Z. Luo, D. K. Britt, H. Furukawa, O. M. Yaghi, K. L. Hardcastle and C. L. Hill, J. Am. Chem. Soc., 2011, 133, 16839 CrossRef CAS PubMed.
- Z. Y. Zhang, Q. P. Lin, D. Kurunthu, T. Wu, F. Zuo, S. T. Zheng, C. J. Bardeen, X. H. Bu and P. Y. Feng, J. Am. Chem. Soc., 2011, 133, 6934 CrossRef CAS PubMed.
- L. Chen, F. L. Jiang, Z. Z. Lin, Y. F. Zhou, C. Y. Yue and M. C. Hong, J. Am. Chem. Soc., 2005, 127, 8588 CrossRef CAS PubMed.
- A. Seliverstov and C. Streb, Chem. Commun., 2014, 50, 1827 RSC.
- C. L. Hill, Angew. Chem., Int. Ed., 2004, 43, 402 CrossRef CAS PubMed.
- Z. G. Han, X. Q. Chang, J. S. Yan, K. N. Gong, C. Zhao and X. L. Zhai, Inorg. Chem., 2014, 53, 670 CrossRef CAS PubMed.
- D. L. Long, E. Burkholder and L. Cronin, Chem. Soc. Rev., 2007, 36, 105 RSC.
- L. S. Felices, P. Vitoria, J. M. Gutiérrez-Zorrilla, S. Reinoso, P. Vitoria and L. Lezama, Chem.–Eur. J., 2004, 10, 5138 CrossRef PubMed.
- P. J. Hagrman, D. Hagrman and J. Zubieta, Angew. Chem., Int. Ed., 1999, 38, 3165 CrossRef.
- B. S. Bassil, M. H. Dickman, I. Romer, B. V. D. Kammer and U. Kortz, Angew. Chem., Int. Ed., 2007, 46, 6192 CrossRef CAS PubMed.
- H. H. Yu, X. B. Cui, J. W. Cui, L. Kong, W. J. Duan, J. Q. Xu and T. G. Wang, Dalton Trans., 2008, 195 RSC.
- D. Y. Du, J. S. Qin, S. L. Li, Z. M. Su and Y. Q. Lan, Chem. Soc. Rev., 2014, 43, 4615 RSC.
- A. Proust, R. Thouvenot and P. Gouzerh, Chem. Commun., 2008, 1837 RSC.
- P. Mialane, A. Dolbecq and F. Sécheresse, Chem. Commun., 2006, 3477 RSC.
- J. Y. Niu, P. T. Ma, H. Y. Niu, J. Li, J. W. Zhao, Y. Song and J. P. Wang, Chem.–Eur. J., 2007, 13, 8739 CrossRef CAS PubMed.
- Q. G. Zhai, X. Y. Wu, S. M. Chen, Z. G. Zhao and C. Z. Lu, Inorg. Chem., 2007, 46, 5046 CrossRef CAS PubMed.
- C. Ritchie, E. Burkholder, P. Kögerler and L. Cronin, Dalton Trans., 2006, 14, 1712 RSC.
- L. Lisnard, A. Dolbecq, P. Mialane, J. Marrot, E. Codjovi and F. Sécheresse, Dalton Trans., 2005, 24, 3913 RSC.
- H. Liu, C. Qin, Y. G. Wei, L. Xu, G. G. Gao, F. Y. Li and X. S. Qu, Inorg. Chem., 2008, 47, 4166 CrossRef CAS PubMed.
- X. S. Qu, L. Xu, G. G. Gao, F. Y. Li and Y. Y. Yang, Inorg. Chem., 2007, 46, 4775 CrossRef CAS PubMed.
- X. L. Wang, Y. F. Bi, B. K. Chen, H. Y. Lin and G. C. Liu, Inorg. Chem., 2008, 47, 2442 CrossRef CAS PubMed.
- Y. Q. Lan, S. L. Li, Z. M. Su, K. Z. Shao, J. F. Ma, X. L. Wang and E. B. Wang, Chem. Commun., 2008, 1, 58 RSC.
- C. D. Zhang, S. X. Liu, C. Y. Sun, F. J. Ma and Z. M. Su, Cryst. Growth Des., 2009, 8, 3655 Search PubMed.
- B. Liu, Z. T. Yu, J. Yang, W. Hua, Y. Y. Liu and J. F. Ma, Inorg. Chem., 2011, 50, 8967 CrossRef CAS PubMed.
- H. Y. Liu, J. Yang, Y. Y. Liu and J. F. Ma, Dalton Trans., 2012, 41, 9922 RSC.
- Y. Q. Lan, S. L. Li, X. L. Wang, K. Z. Shao, D. Y. Du, H. Y. Zang and Z. M. Su, Inorg. Chem., 2008, 47, 8179 CrossRef CAS PubMed.
- J. Q. Sha, J. Peng, Y. Q. Lan, Z. M. Su, H. J. Pang, X. A. Tian, P. P. Zhang and M. Zhu, Inorg. Chem., 2008, 47, 5145 CrossRef CAS PubMed.
- H. Y. An, L. Wang, Y. Hu, T. Q. Xu and Y. J. Hou, Inorg. Chem., 2016, 55, 144 CrossRef CAS PubMed.
- H. Y. An, Y. G. Li, D. X. Xiao, E. B. Wang and C. Y. Sun, Cryst. Growth Des., 2006, 6, 1107 CAS.
- H. Y. An, Y. G. Li, E. B. Wang, D. R. Xiao, C. Y. Sun and L. Xu, Inorg. Chem., 2005, 44, 6062 CrossRef CAS PubMed.
- P. Gouzerh and A. Proust, Chem. Rev., 1998, 98, 77 CrossRef CAS PubMed.
- H. Y. An, L. Wang, Y. Hu and F. Fei, CrystEngComm, 2015, 7, 1531 RSC.
-
(a) R. F. D'Vries, M. Iglesias, N. Snejko, E. Gutiérrez-Puebla and M. A. Monge, Inorg. Chem., 2012, 51, 11349 CrossRef PubMed;
(b) R. F. D'Vries, V. A. Peña-O'Shea, N. Snejko, M. Iglesias, E. Gutiérrez-Puebla and M. A. Monge, Cryst. Growth Des., 2012, 12, 5535 CrossRef.
- M. North, D. L. Usanov and C. Young, Chem. Rev., 2008, 108, 5146 CrossRef CAS PubMed.
- K. Suzuki, M. Sugawa, Y. Kikukawa, K. Kamata, K. Yamaguchi and N. Mizuno, Inorg. Chem., 2012, 51, 6953 CrossRef CAS PubMed.
- Q. X. Han, X. P. Sun, J. Li, P. T. Ma and J. Y. Niu, Inorg. Chem., 2014, 53, 6107 CrossRef CAS PubMed.
- Y. F. Qi, Y. G. Li, C. Qin, E. B. Wang, H. Jin, D. R. Xiao, X. L. Wang and S. Chang, Inorg. Chem., 2007, 46, 3217 CrossRef CAS PubMed.
- J. Q. Sha, J. Peng, A. X. Tian, H. S. Liu, J. Chen, P. P. Zhang and Z. M. Su, Cryst. Growth Des., 2007, 7, 2535 CAS.
- W. T. Xu, F. L. Jiang, Y. F. Zhou, K. C. Xiong, L. Chen, M. Yang, R. Feng and M. C. Hong, Dalton Trans., 2012, 41, 7737 RSC.
- J. S. Yan, K. N. Gong, X. L. Xue, X. L. He, C. Zhao, Z. G. Han and H. T. Yu, Eur. J. Inorg. Chem., 2014, 5969 CrossRef CAS.
- R. J. H. Gregory, Chem. Rev., 1999, 99, 3649 CrossRef CAS PubMed.
-
(a) G. A. Tsigdinos, PhD thesis, Boston University, 1961 Search PubMed;
(b) A. L. Nolan, R. C. Burns and G. A. Lawrance, Dalton Trans., 1996, 2629 RSC;
(c) U. Lee and H. C. Joo, Acta Crystallogr., Sect. E: Struct. Rep. Online, 2010, 66, i10 CAS.
- H. T. Evans and J. S. Showell, J. Am. Chem. Soc., 1969, 91, 6881 CrossRef CAS.
-
(a) G. M. Sheldrick, SHELXL 97, Program for Crystal Structure Refinement, University of Gőttingen, Germany, 1997 Search PubMed;
(b) G. M. Sheldrick, SHELXL 97, Program for Crystal Structure Solution, University of Gőttingen, Germany, 1997 Search PubMed.
- Z. X. Zhang, M. Sadakane, T. Murayama, N. Sakaguchi and W. Ueda, Inorg. Chem., 2014, 53, 7309 CrossRef CAS PubMed.
- I. D. Brown and D. Altermatt, Acta Crystallogr., Sect. B: Struct. Sci., 1985, 41, 244 CrossRef.
- C. I. Cabello, F. M. Cabrerizo, A. Alvarez and H. J. Thomas, J. Mol. Catal. A: Chem., 2002, 186, 89 CrossRef CAS.
- R. F. D'Vries, M. Iglesias, N. Snejko, E. Gutiérrez-Puebla and M. A. Monge, Inorg. Chem., 2012, 51, 11349 CrossRef PubMed.
- M. North, D. L. Usanov and C. Young, Chem. Rev., 2008, 108, 5146 CrossRef CAS PubMed.
- Z. G. Wang, B. Fetterly and J. G. Verkade, J. Organomet. Chem., 2002, 646, 161 CrossRef CAS.
- J. J. Song, F. Gallou, J. T. Reeves, Z. L. Tan, N. K. Yee and C. H. Senanayake, J. Org. Chem., 2006, 71, 1273 CrossRef CAS PubMed.
- X. H. Liu, B. Qin, X. Zhou, B. He and X. M. Feng, J. Am. Chem. Soc., 2005, 127, 12224 CrossRef CAS PubMed.
-
(a) T. P. Hu, Y. Q. Zhao, K. Mei, S. J. Lin, X. P. Wang and D. Sun, CrystEngComm, 2015, 17, 5947 RSC;
(b) P. Phuengphai, S. Youngme, P. Gamez and J. Reedijk, Dalton Trans., 2010, 39, 7936 RSC;
(c) S. Neogi, M. K. Sharma and P. K. Bharadwaj, J. Mol. Catal. A: Chem., 2009, 299, 1 CrossRef CAS.
Footnote |
† Electronic supplementary information (ESI) available: IR, TG curves and PXRD patterns for 1–4, IR spectra for as-synthesized and recovered catalyst 2 are available. Study on recycling of catalyst 2 is listed in Table S1. CCDC 1449669 for 1, 1449670 for 2, 1449671 for 3, 1449672 for 4. For ESI and crystallographic data in CIF or other electronic format see DOI: 10.1039/c6ra14894h |
|
This journal is © The Royal Society of Chemistry 2016 |