DOI:
10.1039/C6RA14733J
(Paper)
RSC Adv., 2016,
6, 93303-93308
A facile method for the synthesis of CuInS2–ZnS quantum dots with tunable photoluminescent properties
Received
7th June 2016
, Accepted 9th September 2016
First published on 13th September 2016
Abstract
CuInS2 quantum dots were synthesized via a solvothermal approach by heating up a mixture of the corresponding metal precursors and dodecanethiol in octadecene. By coating ZnS shells around the CuInS2 quantum dot cores directly in a pristine reaction solution, we successfully synthesized CuInS2–ZnS quantum dots with good monodispersion, spherical morphology and a diameter of 7 nm on average. The absorption and PL emission spectra of the CuInS2 QDs were controllable from 600 nm to 550 nm and from 670 nm to 600 nm, respectively. With the growth of ZnS, the percentage of internal defect recombination increased, which lead to the enhancement of PL intensity of CuInS2–ZnS QDs. The PL intensity and peak position of CuInS2 and CuInS2–ZnS QDs could also be tuned by varying the excitation wavelength. Besides, the reaction temperature and time play important roles on the photoluminescent properties of the CuInS2–ZnS quantum dots. This line of research finds feasible approaches to prepare CuInS2–ZnS quantum dots with great controllable photoluminescent properties. The CuInS2–ZnS quantum dots could greatly contribute to the development of quantum dot light-emitting diode (QLEDs) devices and other potential applications due to their non-toxicity and excellent optical properties.
Introduction
Over the past two decades, colloidal luminescent semiconductor nanocrystals, which are also called quantum dots (QDs), have been exclusively studied due to their unique optical properties and wide applications in photovoltaics,1–7 light emitting diodes,8–14 biomedical labelling15,16 and so on. Binary II–VI compounds, mainly cadmium chalcogenide-based QDs, have been studied intensively to tune their photoluminescence (PL) in the visible spectrum by changing their sizes and compositions.17–22 Unfortunately, the intrinsic toxicity of cadmium impedes the practical application of these QDs, so it is urgent to develop cadmium-free QDs to reach non-toxic luminescence, especially in the fields of biological and medical applications.
In recent years, I–III–VI compounds-based QDs, especially CuInS2 QDs, have gained increasing attention due to their nontoxicity, which makes the CuInS2 QDs promising candidates for energy23–25 and healthcare fields.26 However when it comes to the practical application, there are still lots of problems need to be solved, such as large-scale production of CuInS2 QDs and precise control of their optical properties. Up to now, much work has been done to prepare CuInS2 QDs, tune PL behaviour and enhance fluorescence quantum yield (QY). For example, Zhong et al. synthesized spheric, fluorescence-tunable CuInS2 QDs by using thermolysis of a mixed solution of Cu(OAc), In(OAc)3 and dodecanethiol (DDT) in noncoordinating solvent 1-octadecene (ODE) in gram-scale.27 Li et al. used a “core–shell” structure to increase the QY of the CuInS2 QDs up to 10-fold with a blue shift of PL peak position by using ZnS as the shell material.28 Zhang et al. prepared Cu–Zn–In–S alloyed QDs and widened the range of PL spectrum from UV-Vis to NIR through changing the Cu
:
Zn ratios from 1
:
20 to 2
:
1.29 Zhang et al. developed a green method for the one-pot direct synthesis of hydrophilic CuInS2 and CuInS2–ZnS QDs by employing N,N-dimethylformamide (DMF) as a solvent.30
Herein, we successfully synthesized CuInS2 quantum dots with good monodispersion by heating up a mixture of the corresponding metal precursors and dodecanethiol in octadecene. Subsequently, CuInS2–ZnS QDs were obtained by coating the as-prepared CuInS2 quantum dots with the ZnS shell. Such CuInS2–ZnS QDs show a remarkable blue shift of the PL emission and UV-Vis absorption and a great enhancement of the luminescent intensity. On this basis, we further studied the PL behaviour of the obtained QDs under different excitation wavelengths and the influence of experimental parameters, such as reaction temperature and time, on the optical properties of the core–shell CuInS2–ZnS QDs. This work leads us to feasible approaches to tune the optical properties of CuInS2–ZnS QDs. Due to their excellent properties and non-toxicity, CuInS2–ZnS QDs are one of great candidates for the applications in such optical devices as QLEDs.
Experimental section
Materials
Zinc stearate (ZnSt2, 86–87.5%), 1-octadecene (ODE, 90%), 1-dodecanethiol (DDT, 98%) were purchased from Alfa Aesar, cuprous acetate (Cu(OAc), 99%) from Strem Chemicals, indium acetate (In(OAc)3, 99.99%) and oleylamine (OAm, 80–90%) from Acros Organics, and oleic acid (OA, AR) from Xilong Chemical Co. Ltd., China. All the above chemicals were used as received.
Preparation of stock solution
The zinc solution for the ZnS shell growth was prepared by dissolving 1.27 g (2 mmol) of ZnSt2 in a mixture of 8 mL ODE and 2 mL OAm at 120 °C under flowing argon. The obtained zinc stock solution was stored for the following use.
Synthesis of CuInS2 and CuInS2–ZnS QDs
In a typical procedure, Cu(OAc) (0.245 g, 2 mmol), In(OAc)3 (0.584 g, 2 mmol) together with 10 mL DDT and 50 mL ODE were loaded in a 250 mL flask at room temperature. The mixture was then heated up under argon flow, in which 2 mL OA was dropped in fast at 90 °C. It was further heated to 220 °C and kept at this temperature for 1 hour allowing for CuInS2 QDs to grow. The as-prepared QDs were precipitated by adding hexane and excessive acetone into the sample and purified by repeating centrifugation and decantation cycles.
Growth of the ZnS shell around the CuInS2 core template was carried out in pristine Cu–In–S reaction mixture. The reaction system was cooled down to 120 °C first, where the zinc stock solution was injected subsequently. After that, the temperature of this mixture was raised to 240 °C for CuInS2–ZnS QDs growth. Aliquots of the sample were taken out at different time intervals. Purification of CuInS2–ZnS QDs was similar to that of CuInS2 QDs as described above.
Characterization
PL spectra were recorded on a Hitachi F-7000 fluorescence spectrometer. UV-Vis spectra were achieved on a Shimadzu UV-Vis-NIR spectrophotometer. PL dynamics were measured on Edinburgh FLS920. Transmission electron microscopy (TEM) images were acquired using a Tecnai F30 S-TWIN transmission electron microscope. The TEM samples were obtained by depositing a drop of QDs dispersion in hexane onto molybdenum grids. Powder X-ray diffraction (XRD) was performed using wide-angle X-ray diffraction on a Bruker D8 Advance X-ray powder diffractometer. XRD samples were prepared by depositing QDs powder on a piece of Si (100) wafer. X-ray photoelectron spectroscopy (XPS) was carried out on an Escalab 250Xi X-ray photoelectron spectrometer.
Results and discussion
Morphology and structure of CuInS2 and CuInS2–ZnS QDs
The CuInS2 QDs were prepared by heating up a mixture of corresponding metal precursors and dodecanethiol in octadecene media. This facile method shows high reproducibility and scale-up capability.27,28 Here, DDT is served as not only the anionic precursor, but also the ligand and solvent, which can eliminate the surface trap-states,31 allow for nearly complete consumption of cationic precursors, and minimize the waste of reagents. The CuInS2 QDs were synthesized to demonstrate this facile approach in preparing high-quality CuInS2 QDs, and the detailed procedures were described in the Experimental section. Morphology and structure characterization of CuInS2 and CuInS2–ZnS QDs were performed using XRD and TEM (Fig. 1). The wide-angle XRD patterns of the obtained QDs showed the characteristic peaks of the tetragonal chalcopyrite structure. After being coated by ZnS shell, the QDs maintained in the tetragonal structure but the diffraction peaks shifted to larger angles, which is consistent with the smaller lattice constant for ZnS compared to CuInS2. The TEM images demonstrate that the as-prepared QDs are of near-spherical shape and have a narrow size distribution. Fig. 1b and c show that the average diameter of CuInS2 and CuInS2–ZnS QDs is nearly 3.0 ± 0.3 nm and 7.0 ± 0.2 nm, respectively.
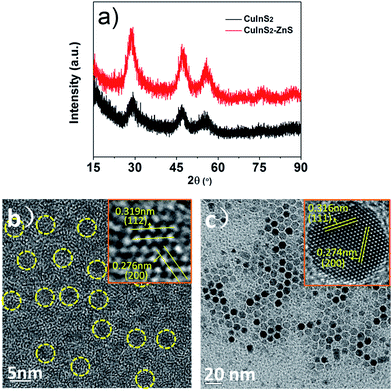 |
| Fig. 1 (a) XRD patterns of CuInS2 and CuInS2–ZnS QDs. (b) TEM and HRTEM images of CuInS2 QDs, (c) TEM and HRTEM images of CuInS2–ZnS QDs. | |
To investigate the compositions and valence states of the as-prepared QDs, EDS and XPS analysis were carried out (shown in Tables 1 and 2 and Fig. 2). The element composition of CuInS2 QDs is CuIn0.95S2.13, in which the larger S content is probably owing to the capping thiol ligands existed on the QD surface. After the growth of ZnS shell, the element composition is CuIn0.84Zn1.32S2.89. It is noted that compared to the nominal Cu/In/Zn ratio (1/1/1), the real value of Zn is relatively higher, which might be attributed to a partial cationic exchange.32 The binding energy of XPS narrow scans on Cu 2p, In 3d, Zn 2p and S 2p peaks of the as-prepared QDs demonstrated that the chemical states were Cu+, In3+, Zn2+ and S2−, respectively.
Table 1 EDX analysis of CuInS2 QDs
Element |
Weight/% |
Atomic/% |
Uncert./% |
Cu |
26.33 |
24.47 |
0.43 |
In |
45.30 |
23.29 |
1.13 |
S |
28.36 |
52.23 |
0.39 |
Table 2 EDX analysis of CuInS2–ZnS QDs
Element |
Weight/% |
Atomic/% |
Uncert./% |
Cu |
18.76 |
16.54 |
0.46 |
In |
28.43 |
13.87 |
1.26 |
Zn |
25.47 |
21.83 |
0.54 |
S |
27.31 |
47.74 |
0.45 |
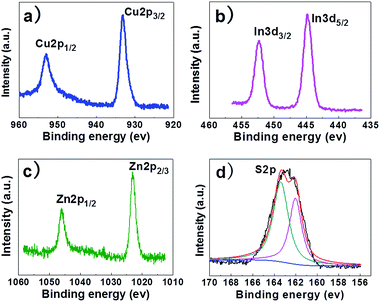 |
| Fig. 2 Electronic binding energy of XPS narrow scan spectra of (a) Cu 2p, (b) In 3d, (c) Zn 2p and (d) S 2p, respectively. | |
Influence of ZnS shell on the optical properties of CuInS2 QDs
The as prepared CuInS2 and CuInS2–ZnS QDs exhibit interesting optical properties. Fig. 3a shows the typical UV-Vis absorption spectra and PL spectra of CuInS2 QDs. The absorption spectra show a broad shoulder with a trail in the long-wavelength direction. Being different from the binary II–VI QDs, the absorption spectra of ternary CuInS2 QDs has no well-defined exciton absorption peaks. Two reasons why this phenomenon occurred were stated in previous publications.27 On the one hand, the size/shape inhomogeneity of the ensemble can be associated with the influence of quantum confinement. On the other hand, the irregular composition distribution among different nanocrystals could result in continuous distribution of the band width. Although there is no obvious absorption peak, the CuInS2 QDs still have an absorption edge at around 600 nm which is significantly blue-shifted from that of bulk CuInS2 (810 nm) and is consistent with the expected quantum confinement effect. The PL spectrum shown in Fig. 3a exhibits a typical emission peak at around 668 nm with a full width at half-maximum (fwhm) of ∼100 nm, and the apparent Stokes shift is ∼120 nm. The large Stokes shift decreases the self-absorption of QDs and ensures their practical application in QLEDs.
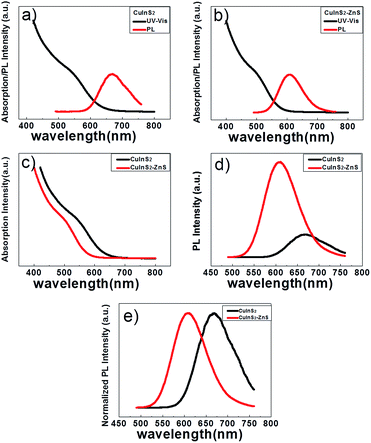 |
| Fig. 3 UV-Vis/PL spectra of (a) CuInS2 and (b) CuInS2–ZnS QDs, comparison of (c) UV/Vis and (d) PL of CuInS2 and CuInS2–ZnS QDs ZnS growth time is 20 min. (e) is normalized corresponding to (d). | |
Further injection of zinc precursor to the Cu–In–S reaction mixture led to the formation of a CuInS2–ZnS core–shell nanostructure. The typical UV-Vis absorption spectra and PL spectra are shown in Fig. 3b. After being coated with ZnS shell, the UV-Vis absorption spectra and PL spectra both show an evident blue-shift (shown in Fig. 3c–e) compared to the CuInS2 QDs. No obvious absorption peak can be found, and the absorption edge is at around 550 nm with a 50 nm shift compared to as prepared CuInS2 QDs. Meanwhile, the PL spectrum of the CuInS2–ZnS QDs shown in Fig. 3b exhibits a typical emission peak at around 605 nm which has blue-shift about 60 nm compared to CuInS2 QDs (shown in Fig. 3e) with a full width at half-maximum (fwhm) of ∼89 nm, and the apparent Stokes shift is nearly 110 nm. The decreased fwhm indicates a greater monochromaticity of QDs which makes them promising materials for QLEDs and displays. Besides, it can be found that the PL intensity enhanced greatly after the growth of ZnS shell (Fig. 3d). Recently, it has been reported that the core–shell structure can enhance the luminescent efficiency for some reasons.31 The type I band alignment makes both carriers (electrons and holes) localize in the core, which increases the rate of electron–hole recombination process. Furthermore, ZnS shell can eliminate surface trap-states of the CuInS2 core, which diminished the non-radiative electron–hole recombination process and improved the thermal and chemical stability of QDs. The blue-shift of the PL peak position after being coated with ZnS shell indicates that further incorporation of zinc into the core material can result in an increase of the band gap energy.33–35
The radiative lifetime of PL emission is another important optical property of semiconductor nanocrystals that needs to be further studied. Different radiative lifetimes may correspond to different electron–hole recombination mechanisms. To demonstrate the two recombination pathways,28 time-resolved laser technique was used to study the lifetime of PL emission. Fig. 4 shows the PL decay curves of the CuInS2 and CuInS2–ZnS QDs. The PL decay curves can be well fitted by a double-exponential function:
I(t) = A1 exp(−t/τ1) + A2 exp(−t/τ2). |
where
τ represents the decay time of the PL emission.
A represents the amplitude of the decay components at
t = 0, relative data are listed in
Table 3.
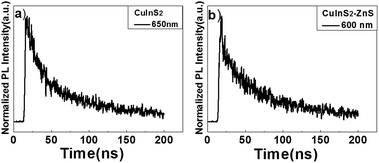 |
| Fig. 4 PL decay curves of the (a) CuInS2 and (b) CuInS2–ZnS QDs. | |
Table 3 Decay times and amplitude constant ratios of the PL emission for CuInS2 and CuInS2–ZnS QDs
Sample |
Wavelength/nm |
τ1/ns |
A1/% |
τ2/ns |
A2/% |
CuInS2 |
650 |
14.768 |
21.486 |
80.549 |
78.514 |
CuInS2–ZnS |
600 |
5.613 |
5.12 |
52.93 |
94.88 |
Influence of the excitation wavelength on the optical properties of CuInS2–ZnS QDs
It was also found that the optical properties of the prepared CuInS2 and CuInS2–ZnS QDs could be affected by the change of excitation wavelength, as shown in Fig. 5. The PL intensity increased first, and then decreased with increasing excitation wavelengths from 550 nm to 630 nm. The maximum of PL intensity was obtained at the excitation wavelength of 590 nm. This phenomenon could be attributed to the different absorption intensities at different excitation wavelengths. The UV-Vis absorption spectrum of CuInS2 QDs above tells us the exciton absorption peak is around 570 nm, which agrees well with the photoluminescent behaviour of the QDs. As the excitation wavelength red shifted, the PL peak also had a red-shift from about 665 nm to 685 nm. There are two recombination pathways observed in CuInS2 QDs that involve an electron in the quantized conduction band state and a hole trapped at either a surface defect which is the non-radiative recombination process or an internal defect which is the radiative recombination process.28 Since the internal defect state is fixed, the larger excitation wavelength, the lower quantized conduction band state electrons are in, which leads to release of photons with smaller energies when electron and hole recombine. That is why the PL emission peak red shifted along with the excitation wavelength. Meanwhile, similar phenomenon was found in CuInS2–ZnS QDs, the largest PL intensity was observed under the excitation of 530 nm which is also around the corresponding UV-Vis absorption band and the PL emission peak moved from about 604 nm to 630 nm when the excitation wavelength ranged from 510 nm to 590 nm.
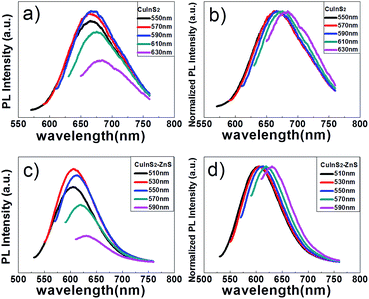 |
| Fig. 5 PL spectra of CuInS2 and CuInS2–ZnS QDs with different excitation wavelength, (b), (d) is normalized corresponding to (a), (c). | |
Influence of the reaction temperature and time on the optical properties of CuInS2–ZnS QDs
The PL peak position and intensity of the resulting CuInS2–ZnS QDs were also found to be dependent on the preparation parameters such as the reaction temperature and time. Therefore, we studied the influences of these two variables on the optical properties of the obtained QDs while maintaining all the other experimental variables as described in the Experimental section.
Reaction temperature plays a key role on the growth process, including surface adsorption, lattice incorporation, lattice diffusion, and etc.36,37 Fig. 6a and b show the PL spectra of the samples with different reaction temperatures. It could be noticed that with the reaction temperature increasing, the PL intensity increased initially and declined afterwards while the temperature exceeded 230 °C. The PL peak kept a blue-shift from about 616 nm to 580 nm as the reaction temperature increased from 210 °C to 240 °C. This blue-shift indicated a further incorporation of zinc into the core material, so it can be noted that increasing the reaction temperature could result in the precipitate during the growth of ZnS shell. The PL intensity enhancement could be ascribed to the core–shell structure mentioned above, for the ZnS shell will continue to grow with the increasing reaction temperature. Whereas the declination of PL intensity at higher reaction temperature might be attributed to the destruction of DDT ligands29 on the QDs surface.
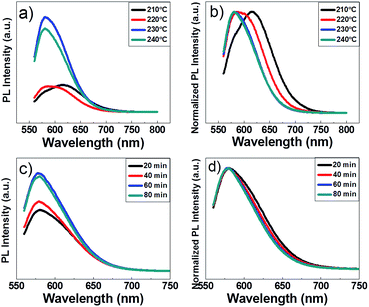 |
| Fig. 6 (a) PL spectra of CuInS2–ZnS QDs with different reaction temperature and a fixed reaction time of 20 min. (b) Normalized PL spectra corresponding to (a). (c) PL spectra of CuInS2–ZnS QDs with different reaction time and a fixed reaction temperature of 240 °C. (d) Normalized PL spectra corresponding to (c). | |
The influence of the reaction time on the PL spectra of the samples is shown in Fig. 6c and d. It can be noticed that, as the reaction time prolonged, the PL intensity increased firstly, and then declined. The largest PL intensity was obtained at the reaction time of 60 min. The whole process was accompanied by a light blue-shift of the PL emission peak position, which is similar to that of the reaction temperature.
Conclusions
High quality CuInS2 QDs with an average size of ∼3 nm were prepared using a solvothermal approach, and CuInS2–ZnS QDs with an average size of ∼7 nm were synthesized by the growth of ZnS shell on the CuInS2 core. The as-prepared CuInS2 and CuInS2–ZnS nanocrystals were in a tetragonal crystalline structure. The measurement results indicated that, the absorption and PL emission spectra of the CuInS2 QDs were controllable from 600 nm to 550 nm and from 670 nm to 600 nm, respectively. The PL decay curve of the CuInS2 nanoparticles had a bi-exponential characteristic which implied that surface defect recombination process and internal defect recombination process were both involved in the PL emission of CuInS2 QDs. With the growth of ZnS, the percentage of internal defect recombination increased which lead to the enhancement of PL intensity. The PL intensity and peak position of CuInS2 and CuInS2–ZnS QDs could also be tuned by varying the excitation wavelengths. The fluorescence of CuInS2 nanocrystals could be achieved from 665 nm to 685 nm when excitation wavelength changed from 550 nm to 630 nm. Meanwhile, for CuInS2–ZnS nanocrystals, the fluorescence could be achieved from 600 nm to 630 nm with the excitation wavelength changing from 510 nm to 590 nm. Furthermore, reaction temperature and time also played key roles on the optical properties of CuInS2–ZnS QDs. As reaction temperature increased, the PL peak position blue shifted from about 625 nm to 585 nm. And the PL intensity increased firstly, then decreased slightly. The reaction time had a similar effect trend on the PL spectra of the samples. Overall, the cadmium-free CuInS2 and CuInS2–ZnS QDs demonstrate great potential for practical applications in QLEDs and bio-labelling due to their non-toxicity and excellent optical properties.
Conflict of interest
The authors declare no competing financial interest.
Acknowledgements
H. W. acknowledges Prof. Quanlin Liu and Dr Zhen Song at USTB for PL spectra measurement. The authors are grateful for the financial support from the National Natural Science Foundation of China (51101013) and the Fundamental Research Funds for the Central Universities (FRF-TP-14-012A2, FRF-TP-15-007A3).
Notes and references
- D. V. Talapin, J. S. Lee, M. V. Kovalenko and E. V. Shevchenko, Chem. Rev., 2010, 110, 389–458 CrossRef CAS PubMed.
- J. Wang, I. Mora-Seró, Z. Pan, K. Zhao, H. Zhang, Y. Feng, G. Yang, X. Zhong and J. Bisquert, J. Am. Chem. Soc., 2013, 135, 15913–15922 CrossRef CAS PubMed.
- Z. Zhu, J. Ma, Z. Wang, C. Mu, Z. Fan, L. Du, Y. Bai, L. Fan, H. Yan, D. L. Phillips and S. Yang, J. Am. Chem. Soc., 2014, 136, 3760–3763 CrossRef CAS PubMed.
- Z. Pan, I. Mora-Seró, Q. Shen, H. Zhang, Y. Li, K. Zhao, J. Wang, X. Zhong and J. Bisquert, J. Am. Chem. Soc., 2014, 136, 9203–9210 CrossRef CAS PubMed.
- Z. Zhang, J. Yang, X. Wen, L. Yuan, S. Shrestha, J. A. Stride, G. J. Conibeer, R. J. Patterson and S. Huang, J. Phys. Chem. C, 2015, 119, 24149–24155 CAS.
- S. T. Cate, C. S. S. Sandeep, Y. Liu, M. Law, S. Kinge, A. J. Houtepen, J. M. Schins and L. D. A. Siebbeles, Acc. Chem. Res., 2015, 48, 174–181 CrossRef PubMed.
- A. H. Ip, A. Kiani, I. J. Kramer, O. Voznyy, H. F. Movahed, L. Levina, M. M. Adachi, S. Hoogland and E. H. Sargent, ACS Nano, 2015, 9, 8833–8842 CrossRef CAS PubMed.
- A. L. Rogach, N. Gaponik, J. M. Lupton, C. Bertoni, D. E. Gallardo, S. Dunn, N. L. Pira, M. Paderi, P. Repetto, S. G. Romanov, C. O'Dwyer, C. M. S. Torres and A. Eychmüller, Angew. Chem., Int. Ed., 2008, 47, 6538–6549 CrossRef CAS PubMed.
- Q. Sun, Y. A. Wang, L. S. Li, D. Wang, T. Zhu, J. Xu, C. Yang and Y. Li, Nat. Photonics, 2007, 1, 717–722 CrossRef CAS.
- E. Mutlugun, B. Guzelturk, A. P. Abiyasa, Y. Gao, X. Sun and H. V. Demir, J. Phys. Chem. Lett., 2014, 5, 2802–2807 CrossRef CAS PubMed.
- X. Yang, E. Mutlugun, C. Dang, K. Dev, Y. Gao, S. T. Tan, X. Sun and H. V. Demir, ACS Nano, 2014, 8, 8224–8231 CrossRef CAS PubMed.
- H. M. Kim, J. Kim, J. Lee and J. Jang, ACS Appl. Mater. Interfaces, 2015, 7, 24592–24600 CAS.
- J. H. Kim, C. Y. Han, K. H. Lee, K. S. An, W. Song, J. Kim, M. S. Oh, Y. R. Do and H. Yang, Chem. Mater., 2015, 27, 197–204 CrossRef CAS.
- J. Kwak, J. Lim, M. Park, S. Lee, K. Char and C. Lee, Nano Lett., 2015, 15, 3793–3799 CrossRef CAS PubMed.
- P. Zrazhevskiya, M. Senab and X. Gao, Chem. Soc. Rev., 2010, 39, 4326–4354 RSC.
- X. Sun, X. Huang, J. Guo, W. Zhu, Y. Ding, G. Niu, A. Wang, D. O. Kiesewetter, Z. Wang, S. Sun and X. Chen, J. Am. Chem. Soc., 2014, 136, 1706–1709 CrossRef CAS PubMed.
- C. B. Murray, D. J. Norris and M. G. Bawendi, J. Am. Chem. Soc., 1993, 115, 8706–8715 CrossRef CAS.
- L. Qu and X. Peng, J. Am. Chem. Soc., 2002, 124, 2049–2055 CrossRef CAS PubMed.
- X. Zhong, M. Han, Z. Dong, T. J. White and W. Knoll, J. Am. Chem. Soc., 2003, 125, 8589–8594 CrossRef CAS PubMed.
- R. E. Bailey and S. Nie, J. Am. Chem. Soc., 2003, 125, 7100–7106 CrossRef CAS PubMed.
- K. B. Wan, K. Char, H. Hur and S. Lee, Chem. Mater., 2008, 20, 531–539 CrossRef.
- M. D. Regulacio and M. Y. Han, Acc. Chem. Res., 2010, 43, 621–630 CrossRef CAS PubMed.
- M. Sun, D. Zhu, W. Ji, P. Jing, X. Wang, W. Xiang and J. Zhao, ACS Appl. Mater. Interfaces, 2013, 5, 12681–12688 CAS.
- S. H. Park, A. Hong, J. H. Kim, H. Yang, K. Lee and H. S. Jang, ACS Appl. Mater. Interfaces, 2015, 7, 6764–6771 CAS.
- J. Du, Z. Du, J. S. Hu, Z. Pan, Q. Shen, J. Sun, D. Long, H. Dong, L. Sun, X. Zhong and L. J. Wan, J. Am. Chem. Soc., 2016, 138, 4201–4209 CrossRef CAS PubMed.
- T. Pons, E. Pic, N. Lequeux, E. Cassette, L. Bezdetnaya, F. Guillemin, F. Marchal and B. Dubertret, ACS Nano, 2010, 4, 2531–2538 CrossRef CAS PubMed.
- H. Zhong, Y. Zhou, M. Ye, Y. He, J. Ye, C. He, C. Yang and Y. Li, Chem. Mater., 2008, 20, 6434–6443 CrossRef CAS.
- L. Li, A. Pandey, D. J. Werder, B. P. Khanal, J. M. Pietryga and V. I. Klimov, J. Am. Chem. Soc., 2011, 133, 1176–1179 CrossRef CAS PubMed.
- J. Zhang, R. Xie and W. Yang, Chem. Mater., 2011, 23, 3357–3361 CrossRef CAS.
- J. Zhang, W. Sun, L. Yin, X. Miao and D. Zhang, J. Mater. Chem. C, 2014, 2, 4812–4817 RSC.
- J. A. Hollingsworth, Chem. Mater., 2013, 25, 1318–1331 CrossRef CAS PubMed.
- Q. A. Akkerman, A. Genovese, C. George, M. Prato, I. Moreels, A. Casu, S. Marras, A. Curcio, A. Scarpellini, T. Pellegrino, L. Manna and V. Lesnyak, ACS Nano, 2015, 9, 521–531 CrossRef CAS PubMed.
- H. Nakamura, W. Kato, M. Uehara, K. Nose, T. Omata, S. O. Yao-Matsuo, M. Miyazaki and H. Maeda, Chem. Mater., 2006, 18, 3330–3335 CrossRef CAS.
- X. Tang, W. Cheng, E. S. Choo and J. Xue, Chem. Commun., 2011, 47, 5217–5219 RSC.
- W. Zhang and X. Zhong, Inorg. Chem., 2011, 50, 4065–4072 CrossRef CAS PubMed.
- D. Chen, R. Viswanatha, G. L. Ong, R. Xie, M. Balasubramaninan and X. Peng, J. Am. Chem. Soc., 2009, 131, 9333–9339 CrossRef CAS PubMed.
- W. Zhang, X. Zhou and X. Zhong, Inorg. Chem., 2012, 51, 3579–3587 CrossRef CAS PubMed.
Footnote |
† Y. H. Jia and H. C. Wang contributed this paper equally. |
|
This journal is © The Royal Society of Chemistry 2016 |