DOI:
10.1039/C6RA14625B
(Paper)
RSC Adv., 2016,
6, 93956-93962
Obtaining a high value branched bio-alkane from biomass-derived levulinic acid using RANEY® as hydrodeoxygenation catalyst†
Received
6th June 2016
, Accepted 18th September 2016
First published on 19th September 2016
1. Introduction
Biomass represents an abundant carbon-neutral renewable resource for the production of bioenergy and biomaterials, which is the sole renewable carbon source of liquid fuel.1 Cellulose accounts for 75% of the biomass resources in nature, constituting the main fraction of biomass resources. Sugar derivatives from cellulose degradation can be used to synthesize high performance fuels and a variety of high value green chemicals,2 which is of great significance and has a profound impact for academia and industry, especially for the sustainable development of society. Traditional thermal cracking combined with a hydrodeoxygenation (HDO) process only yields low carbon number components (≤C6), limiting the product value due to the low energy density and causing a great waste of biomass resources.3 Therefore, exploring new reaction paths to obtain hydrocarbon compounds with a high carbon number, high energy density and high value from cellulose sources are the critical challenges in biofuel research.4
5-Hydroxymethyfurfural (5-HMF) derived from the dehydration of a six carbon sugar has been envisaged as an important platform compound for biofuel production in biorefinery processes.5 C–C bond coupling can be achieved by aldol condensation, due to the coupling reaction between the aldehyde functional group with ketones, alcohols and other functional groups.6 Furthermore, hydrocarbons with a high number of carbon atoms (≥C9) could be obtained by an HDO process.7 Compared with 5-HMF, levulinic acid is a more promising platform compound. Relying on its rich intramolecular functional groups, carbonyl and carboxyl functional groups, levulinic acid can eliminate one oxygen atom by a unique intramolecular esterification reaction to form angelica lactone, which needs no hydrogen consumption. Therefore, the C
:
O ratio (2.5) of angelica lactone (C5H6O2) is higher than the ratio (2.0) of 5-HMF (C6H6O3), which is very beneficial to the subsequent HDO step due to a lower hydrogen cost. There is another hydrogenation route where levulinic acid could also be used to produce GVL.8 On the other hand, the important C
C bond in the molecular structure of angelica lactone will lead to the C–C coupling and the formation of the self-aggregation dimer (C10). Angelica lactone is a platform compound that has more potential than 5-HMF based on decreasing hydrogen costs for the production of high carbon number alkanes from biomass sources.
As for the HDO step, the first studies on the use of supported nickel for the hydrogenation/hydrogenolysis of sugars and polyols dates back to the 1930s.2b As a consequence of the recent interest in biofuels, many HDO catalysis systems of biomass-derived intermediates have been explored.9 Supported-noble metals and nickel have been used as HDO catalysts.10 Mark Mascal has adopted Cu–ZnO, Pt–ReOx, ReOx and Ir-catalysts for the HDO of cyclic esters and obtained branched alkanes.4c Zhang's result shows that Pd/C is effective for oxygen removal, and his co-workers also used a silica-alumina supported nickel catalyst for the HDO of an angelica lactone dimer and trimer.4b,11 Dumesic discovered a heterogeneous catalyst based on Ni, Sn and Al, which is active and selective for the production by an aqueous-phase reforming of ethylene glycol, glycerol, and sorbitol.12 Serrano and co-workers studied the catalytic conversion of rapeseed oil into raw chemicals and fuels over Ni- and Mo-modified nanocrystalline ZSM-5 zeolite, and also studied the effects of metal and support properties on the bio-oil model compound anisole HDO over supported Ni and Co catalysts.13 RANEY®, a spongy bulky metal nickel catalyst, exhibits a particular catalytic performance and has often been used for several hydrogenation or hydrogenolytic cleavage reactions. It has higher activity due to its more active sites per volume or weight and needs milder conditions for the hydrogenation process compared with some noble metal catalysts. In particular, its surface defect sites could act as special active sites, leading to its exceptional catalytic performance. For example, RANEY® offers high efficiency and selectivity in molecular hydrogen activation.14 It has been used for the hydrogenation of D-glucose, and the kinetics of the hydrogenation of xylose over RANEY® has also been reported.15 However, the HDO of a large C–C bond coupling molecule (C10) from a biomass-derived platform compound has never been reported.
In this study, a 3-step preparation process starting from levulinic acid to obtain high value C10 branched bio-alkanes is presented (Scheme 1). Commercial RANEY® is used as the catalyst for the HDO step.
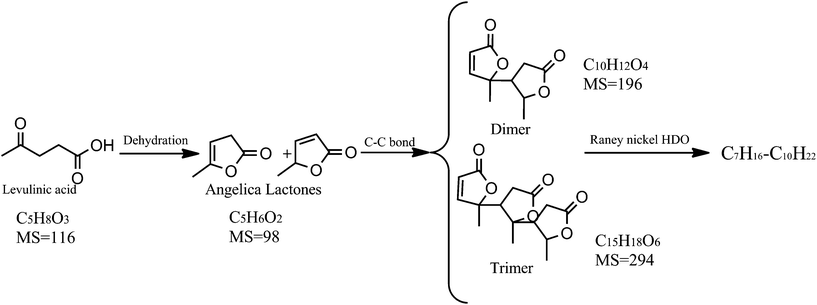 |
| Scheme 1 Reaction pathways for the conversion of levulinic acid into bio-alkanes. | |
2. Experimental
Levulinic acid (99 wt%) was purchased from Langfang Haoke Technology Co., Ltd. Alpha angelica lactone 97% (GC grade) was purchased from Sahn Chemical Technology Co., Ltd. (Shanghai, China). Anhydrous potassium carbonate, anhydrous ethanol, cyclohexane, decahydronaphthalene, petroleum ether, ethyl acetate and phosphate acid were purchased from Tianjin Kemiou Chemical Reagent Co, Ltd.
RANEY® catalyst characterization and evaluation
RANEY® was provided by Hangzhou Fangsheng Chemical Co., Ltd. The XRD patterns of the catalyst was recorded with a Philips PW 3040/00 X'Pert MPD/MRD diffractometer using Cu Kα radiation operated at 45 kV and 40 mA. N2 adsorption–desorption isotherms were determined at 77 K on a Micromeritics TriStar 3000 instrument. The catalyst was first degassed under vacuum at 353 K for 8 h. Scanning electron microscopy (SEM) images were obtained on a JSM-7001F microscope. The TEM (transmission electron microscopy) images were obtained on a JEM-2100F microscope. Temperature programmed reduction (H2-TPR) was carried out in an automatic equipment (Chemisorb 2750, Micromeritics). The sample (0.25 g) was submitted to a heat treatment (from room temperature to 900 °C with 10 °C min−1) in a gas flow (20 mL min−1) of the mixture N2
:
H2 (volume ratio = 95
:
5). XPS was performed under ultrahigh vacuum on a Kratos AXIS ULTRA DLD spectrometer with Al K radiation and a multichannel detector.
3. Results and discussion
Levulinic acid dehydration
Levulinic acid intramolecular dehydration can generate angelica lactone, whereas, angelica lactone will hydrolyze back into levulinic acid. Levulinic acid and angelica lactone are in a state of equilibrium (as shown in Scheme 2(a)).16 If angelica lactone can be separated from the reaction system, the equilibrium will be beneficial for the formation of angelica lactone and the reaction conversion can be considerably improved. Because the boiling point difference between angelica lactone and levulinic acid is large enough (levulinic acid b.p. = 518 K; angelica lactone b.p. = 440 K), reduced pressure distillation method can be employed to separate angelica lactone from the equilibrium reaction system. In the present study, an in situ separation setup was built using packed column distillation for the separation of angelica lactone from the equilibrium system where levulinic acid was refluxed into the reaction system, and angelica lactone was condensed through the cold pillars for collection. This process was designed to separate the generated angelica lactone from the reaction system in time, avoiding further side-reactions of angelica lactone during the reaction process and favoring the equilibrium towards angelica lactone. We carried out the levulinic acid dehydration reaction at different pressures by employing different concentrations of H3PO4, as the catalyst, under different temperatures. The results are summarized in Table 1. The optimal reaction conditions were 9 kPa, 453 K, and 3 wt% H3PO4. Under these conditions, the yield of angelica lactone could reach 60%. The process could yield 120 g of angelica lactone from 200 g of levulinic acid.
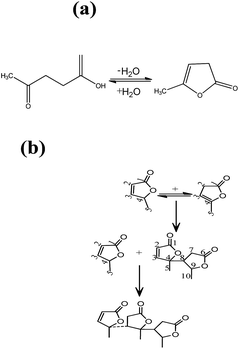 |
| Scheme 2 (a) The equilibrium of angelic lactone and levulinic acid (b) the mechanism of angelic lactone self-aggregation. | |
Table 1 The temperature and catalyst influence on the yield of angelica lactone LA produced into AL under 9 kPa by 1 wt%, 3 wt% and 5 wt% H3PO4 as catalyst at 443 K; LA produced into AL under 9 kPa by 3 wt% H3PO4 as catalyst at 443 K, 453 K and 463 K. LA produced into AL by 3 wt% H3PO4 as catalyst at 453 K under 6, 9, and 12 kPa
No. |
H3PO4 (wt%) |
T [K] |
Yield [%] |
1 |
1 |
443 |
22 |
2 |
3 |
443 |
53 |
3 |
5 |
443 |
45 |
4 |
3 |
443 |
48 |
5 |
3 |
453 |
60 |
6 |
3 |
463 |
58 |
7 |
3 |
453 |
59 |
8 |
3 |
453 |
60 |
9 |
3 |
453 |
49 |
Angelica lactone self-aggregation
It is well known that angelica lactone has two isomers, α-angelica lactone and β-angelica lactone. α-Angelica lactone further isomerizes to produce β-angelica lactone in the presence of catalysts at a high temperature. The two isomers also are in a state of equilibrium (Scheme 2(b)). Under a different catalysis system, the C
C bonds present in the molecular structure of both α-angelica lactone and β-angelica lactone could combine, leading to self-aggregation intermediates (Scheme 2(b)). Zhang used anhydrous K2CO3 as the catalyst and the yield reached up to 90%, and the distribution of di/tri/tetramers ratio was 6
:
3
:
1.4b In our study, the optimal reaction conditions were determined to be a reaction temperature of 353 K and 10 wt% K2CO3. Under these conditions, both the conversion and the yield to the dimer/trimer for the angelica lactone self-aggregation reached nearly 100%. Particularly, the dimer product was found to dominate (75%) (Fig. S1†). In this process, we could obtain nearly 7.5 g of the angelica lactone dimer from 10 g of angelica lactone. The ESI Fig. 2† shows a typical mass spectrum of products in the negative ion mode by time-of-flight mass spectrometry (MADLI/TOF-MS). Besides the peaks originating from the matrix, two peaks were observed at m/z 196 and 294, corresponding to the angelica lactone self-aggregated products namely the dimer and trimer, respectively. Furthermore, 13C NMR spectra were collected to confirm the chemical structures of products, which are shown in compounds are found to be (Fig. S4†). The tertiary and quaternary carbons would be distinguished as compared to DEPT135-13C NMR (Fig. S5†). Herein, we proposed a mechanism where the ratio of α-angelica lactone and β-angelica lactone is almost 6
:
4 before the reaction. Under the presence of anhydrous K2CO3, α-angelica lactone is isomerized to β-angelica lactone because of an alkaline catalysis effect. At the same time, C–C bond splitting took place for both α-angelica lactone and β-angelica lactone where the C3–H of α-angelica lactone broke and the C4–H of β-angelica lactone broke and then, the dimer was produced. When there is a substituent group in the C4 of β-angelica lactone, the C3–H split also occurred for β-angelica lactone. In the same way, for the dimer C3–H, a split took place as well. Therefore, the dimer and β-angelica lactone could combine into a trimer by C–C coupling (Scheme 2(b)).
Hydrodeoxygenation
RANEY® has often been used for hydrogenation/hydrogenolysis reactions and has attracted much attention due to its low cost, high efficiency and milder application conditions, which is understandable from an economic point of view (e.g., the energy input, hydrogen cost and the catalyst lifetime). In the present study, nearly 100% conversion could be reached for the HDO of the angelica lactone self-aggregation intermediates over the RANEY® catalyst because the RANEY® catalyst could be dispersed in the liquid phase of decahydronaphthalene very well and the three dimensional free rotation of the catalyst particles in the liquid phase could be achieved. All of the active sites present over the bulky RANEY® surface could be accessible to the HDO activation, which can significantly improve the catalytic efficiency. Traditional supported catalysts are limited in activity due to the amount of active metals that can be deposited on the pore walls of a support. Bulky RANEY® could sidestep this constraint since it is predominantly a metal that contains porosity. A RANEY® catalyst could bear a higher density of active sites and a higher specific activity per unit volume. On the other hand, the HDO reaction over RANEY® is very sensitive to temperature and a lower temperature will result in a significant drop for the RANEY® catalytic performance. For example, at 463 K the HDO product conversion is 51%, decreasing by 40% as compared with the temperature at 483 K. In the present study, the RANEY® catalyst also displayed excellent reusability. After five cycles of the catalyst, a high conversion above 93% still remained.
All of the HDO products are saturated branched alkanes according to GC result (Fig. 1). RANEY® catalyst could yield a narrow product distribution of C7 (4.92%), C8 (9.16%), C9 (10.27%), and C10 (75.65%). The main product was a high value C10 branched alkane, 3-ethyl-4-methyl heptane, which has a high octane number (RON, 155) and low freezing point (FP, 190 K), and is an important component of high performance jet fuel. The excellent yield to this C10 product results from the mild reaction temperature (463 K). Under this temperature, RANEY® could selectively activate the C–O bond hydrogenolysis and effectively remove oxygen. Moreover the cracking side-reaction of the C–C bond could be suppressed and is not significant. For many hydrotreatment reactions, decahydronaphthalene was proven to be an efficient solvent that is capable of transferring hydrogen, enabling the conversion to be greatly enhanced.17 In our study, it was also found that the solvent effect is evident. If the cyclohexane acts as a solvent, the conversion will decrease by 30% under the same condition. It is clear that decahydronaphthalene is more advantageous in the HDO reaction for the RANEY® system due to the hydrogen-donating benefit. Michael Coleman found that the classical hydrogen-donating solvent also is an excellent high-temperature thermal stabilizer that could efficiently inhibit the thermal cracking of the side-reaction.18 In the present study, the thermal stabilization effect of the decahydronaphthalene solvent also contributes to the minor “cracking” products (less than 25%). Hanford used a RANEY® catalyst for the hydrogenolysis of monosaccharides into glycols and glycerol.19 In his study he claimed an improvement to the process and the possibility of running it under milder conditions by introducing the RANEY® catalyst. The C–C bond of the tertiary carbon structure will be susceptible to the cleavage. In our study, the decarboxylation freed from the tertiary carbon structure of angelica lactone dimers will lead to the C9 (C10–C9) products and the ethyl group cleavage will lead to the C8 (C10–C8) and C7 (C9–C7) products.4c
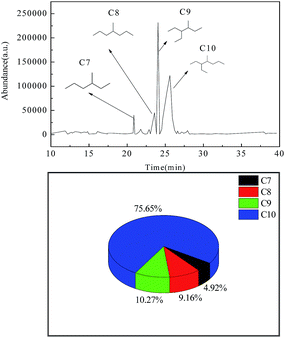 |
| Fig. 1 (a) GC chromatogram of hydrodeoxygenation of angelica lactone di/trimerization products, (b) product distribution of the HDO of angelica lactone di/trimers. | |
Physicochemical characterization of the catalysts
The X-ray diffraction patterns of RANEY® are displayed in Fig. 2(a). The XRD pattern of the commercial RANEY® is concordant with typical crystalline Ni, having diffraction peaks at 46.2 and 51.5°, corresponding to Ni (111) and Ni (200), respectively. The catalyst was produced by alkaline leaching of aluminum from a nickel–aluminum alloy, which consisted of Ni2Al3 and NiAl3 intermetallic phases and pure aluminum.20 Therefore, it is possible that the long time spent in the leaching process (about 8 h) promoted an almost completed dissolution of the other phases in the RANEY® catalyst. Fig. 2(b) shows the H2-TPR spectra from room temperature to 900 °C of the RANEY® catalyst. The sharp hydrogen consumption peaks, which are located at about 100 °C can be assigned to hydrogen adsorption of skeletal nickel. The H2-TPR result illustrates that RANEY® is present in several states with different reducibility. The reduction peaks around 300 °C can be assigned to the reduction of nickel clusters. According to the results of Fig. 3, hydrogen consumption peaks of nickel ranged from 500 °C to 700 °C and indicated that nickel can be generated even though the calcination temperature is merely 800 °C. Furthermore, it is remarkable that the sharp hydrogen consumption peaks before 170 °C can be assigned to hydrogen adsorption of skeletal nickel.
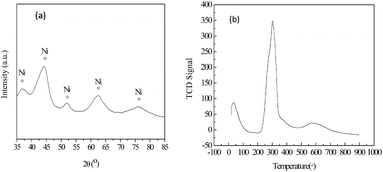 |
| Fig. 2 (a) XRD pattern of the RANEY® catalyst, (b) H2-TPR patterns of the RANEY® catalyst. | |
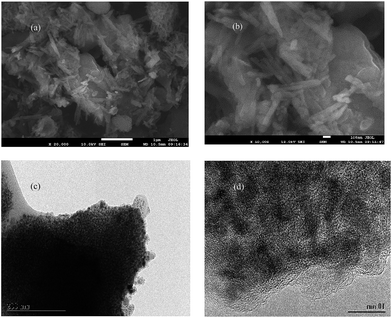 |
| Fig. 3 (a) and (b) SEM images of the RANEY® catalyst, (c) and (d) TEM images of the RANEY® catalyst. | |
Fig. 3(a) and (b) display the SEM images of the RANEY® catalyst. The SEM images show that the RANEY® has a relatively smooth surface and large particle size. There are some cracks on the surfaces of the catalysts, which are caused by the leaching of aluminum. The sample has abundant microstructures, modest dense agglomerates, and some grains. The sample is made up of several blocks with different-sized particles inserted on them. It is possible to observe the sponge-like morphology of the RANEY® catalyst, presenting pores <50 nm and some porous type slits. Details of the catalyst morphology could be better visualized by TEM. The TEMs of the RANEY® catalyst are given in Fig. 3(c) and (d). The figures show the spongy structure of the RANEY®, formed by small nickel domains with no defined shape.
The textural properties of the sample were obtained from the N2 adsorption isotherms (Fig. 4). The adsorption isotherms of the sample are the typical of mesoporous materials (diameters in the range of 5–20 nm). The BET surface area is about 37 m2 g−1. XPS can give information on the chemical compositions of the catalyst in the upper layers from the sample surface (Fig. 5). The catalyst still contained both the metallic and oxide states of nickel and aluminum. Furthermore, the nickel also exists in two states of metallic nickel (Ni 2p3/2 at 856.3 eV) and nickel oxide (Ni 2p3/2 at 861.0 eV). Aluminum oxide (Al 2p at 74.4 eV) could be detected on the RANEY® surface.
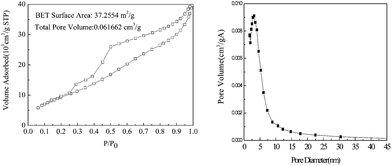 |
| Fig. 4 N2 adsorption–desorption isotherms of the RANEY® catalyst. | |
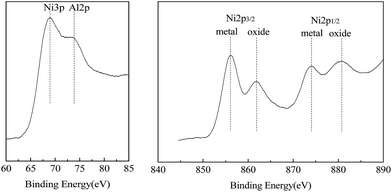 |
| Fig. 5 XPS spectra of Ni 2p, Ni 3p, Al 2p. | |
4. Conclusions
In this study, biomass-derived levulinic acid was used as the only starting raw material that could be transformed into a high value C10 branched bio-alkane via 3 steps. As compared to 5-HMF, angelica lactone is a more potential platform for C–C bond coupling. A RANEY® catalyst dispersed in the decahydronaphthalene displayed a very high activity at mild temperature, significantly suppressing the C–C bond breaking side-reaction.
Acknowledgements
This work received financial support from the Natural Science Foundation of China (21403273), the National Key Basic Research Program of China (973 Program) (2012CB215305), the Shanxi Scholarship Council of China (2015-122) and the Department of Human Resource and Social Security of Shanxi Province (Y6SW9613B1).
Notes and references
-
(a) J. R. Arthur, K. W. Charlotte, H. D. Brian, B. George, C. John, A. E. Charles, J. F. William Jr, P. H. Jason, J. L. David, L. L. Charles, R. M. Jonathan, M. Richard, T. Richard and T. Tschaplinski, The Path Forward for Biofuels, Science, 2006, 311, 484–489 CrossRef PubMed;
(b) D. D. Laskar, M. P. Tucker, X. Chen, G. L. Helms and B. Yang, Noble-metal catalyzed hydrodeoxygenation of biomass-derived lignin to aromatic hydrocarbons, Green Chem., 2014, 16(2), 897 RSC.
-
(a) J. C. Serrano-Ruiz, R. Luque and A. Sepulveda-Escribano, Transformations of biomass-derived platform molecules: from high added-value chemicals to fuels via aqueous-phase processing, Chem. Soc. Rev., 2011, 40(11), 5266–5281 RSC;
(b) A. M. Ruppert, K. Weinberg and R. Palkovits, Hydrogenolysis goes bio: from carbohydrates and sugar alcohols to platform chemicals, Angew. Chem., 2012, 51(11), 2564–2601 CrossRef CAS PubMed.
- J. J. Bozell and G. R. Petersen, Technology development for the production of biobased products from biorefinery carbohydrates—the US Department of Energy's “Top 10” revisited, Green Chem., 2010, 12(4), 539 RSC.
-
(a) A. Corma, O. de la Torre and M. Renz, High-quality diesel from hexose- and pentose-derived biomass platform molecules, ChemSusChem, 2011, 4(11), 1574–1577 CrossRef CAS PubMed;
(b) J. Xin, S. Zhang, D. Yan, O. Ayodele, X. Lu and J. Wang, Formation of C–C bonds for the production of bio-alkanes under mild conditions, Green Chem., 2014, 16(7), 3589 RSC;
(c) M. Mascal, S. Dutta and I. Gandarias, Hydrodeoxygenation of the angelica lactone dimer, a cellulose-based feedstock: simple, high-yield synthesis of branched C7–C10 gasoline-like hydrocarbons, Angew. Chem., 2014, 53(7), 1854–1857 CrossRef CAS PubMed.
-
(a) J. N. Chheda, Y. Roman-Leshkov and J. A. Dumesic, Production of 5-hydroxymethylfurfural and furfural by dehydration of biomass-derived mono- and poly-saccharides, Green Chem., 2007, 9(4), 342 RSC;
(b) J. P. Lange, E. van der Heide, J. van Buijtenen and R. Price, Furfural–a promising platform for lignocellulosic biofuels, ChemSusChem, 2012, 5(1), 150–166 CrossRef CAS PubMed;
(c) H. Wang, T. Deng, Y. Wang, X. Cui, Y. Qi, X. Mu, X. Hou and Y. Zhu, Graphene oxide as a facile acid catalyst for the one-pot conversion of carbohydrates into 5-ethoxymethylfurfural, Green Chem., 2013, 15(9), 2379 RSC.
-
(a) M. Espinal Viguri, M. A. Huertos, J. Perez, L. Riera and I. Ara, Re-mediated C–C coupling of pyridines and imidazoles, J. Am. Chem. Soc., 2012, 134(50), 20326–20329 CrossRef CAS PubMed;
(b) M. J. Monreal and L. P. Diaconescu, Reversible C–C Coupling in a Uranium Biheterocyclic Complex, J. Am. Chem. Soc., 2010, 132, 7676–7683 CrossRef CAS PubMed.
- P. V. Tushar, Z. Huiyan, S. Aimaro, X. Rui and G. W. Huber, Renewable Chemical Commodity Feedstocks from Integrated Catalytic Processing of Pyrolysis Oils, Science, 2010, 330, 1222–1227 CrossRef PubMed.
-
(a) C. Xiao, T.-W. Goh, Z. Qi, S. Goes, K. Brashler, C. Perez and W. Huang, Conversion of Levulinic Acid to γ-Valerolactone over Few-Layer Graphene-Supported Ruthenium Catalysts, ACS Catal., 2016, 6(2), 593–599 CrossRef CAS;
(b) J. Tan, J. Cui, G. Ding, T. Deng, Y. Zhu and Y.-W. Li, Efficient aqueous hydrogenation of levulinic acid to γ-valerolactone over a highly active and stable ruthenium catalyst, Catal. Sci. Technol., 2016, 6(5), 1469–1475 RSC;
(c) S. G. Wettstein, J. Q. Bond, D. M. Alonso, H. N. Pham, A. K. Datye and J. A. Dumesic, RuSn bimetallic catalysts for selective hydrogenation of levulinic acid to γ-valerolactone, Appl. Catal., B, 2012, 117–118, 321–329 CrossRef CAS.
- E. Furimsky, Catalytic hydrodeoxygenation, Appl. Catal., A, 2000, 199, 147–190 CrossRef CAS.
-
(a) J. Lee, Y. T. Kim and G. W. Huber, Aqueous-phase hydrogenation and hydrodeoxygenation of biomass-derived oxygenates with bimetallic catalysts, Green Chem., 2014, 16(2), 708 RSC;
(b) P. Yuan, Z. Liu, W. Zhang, H. Sun and S. Liu, Cu–Zn/Al2O3 Catalyst for the Hydrogenation of Esters to Alcohols, Chin. J. Catal., 2010, 31(7), 769–775 CrossRef CAS;
(c) M. N. Jason, M. B. Lee, G. B. Robert and J. A. Ellman, Catalytic C–O Bond Cleavage of 2-Aryloxy-1-arylethanols and Its Application, J. Am. Chem. Soc., 2010, 132, 12554–12555 CrossRef PubMed;
(d) L. He, H. Cheng, G. Liang, Y. Yu and F. Zhao, Effect of structure of CuO/ZnO/Al2O3 composites on catalytic performance for hydrogenation of fatty acid ester, Appl. Catal., A, 2013, 452, 88–93 CrossRef CAS;
(e) R. V. Maligal-Ganesh, C. Xiao, T. W. Goh, L.-L. Wang, J. Gustafson, Y. Pei, Z. Qi, D. D. Johnson, S. Zhang, F. Tao and W. Huang, A Ship-in-a-Bottle Strategy To Synthesize Encapsulated Intermetallic Nanoparticle Catalysts: Exemplified for Furfural Hydrogenation, ACS Catal., 2016, 6(3), 1754–1763 CrossRef CAS.
- O. O. Ayodele, F. A. Dawodu, D. Yan, X. Lu, J. Xin and S. Zhang, Hydrodeoxygenation of angelica lactone dimers and trimers over silica-alumina supported nickel catalyst, Renewable Energy, 2016, 86, 943–948 CrossRef CAS.
-
(a) G. W. Huber, J. W. Shabaker and J. A. Dumesic, RANEY®–Sn Catalyst for H2 Production from Biomass-Derived Hydrocarbons, Science, 2003, 300, 2075–2077 CrossRef CAS PubMed;
(b) A. Perosa and P. Tundo, Selective Hydrogenolysis of Glycerol with RANEY®, Ind. Eng. Chem. Res., 2005, 44, 8535–8537 CrossRef CAS.
-
(a) J. A. Botas, D. P. Serrano, A. García, J. de Vicente and R. Ramos, Catalytic conversion of rapeseed oil into raw chemicals and fuels over Ni- and Mo-modified nanocrystalline ZSM-5 zeolite, Catal. Today, 2012, 195(1), 59–70 CrossRef CAS;
(b) T. M. Sankaranarayanan, A. Berenguer, C. Ochoa-Hernández, I. Moreno, P. Jana, J. M. Coronado, D. P. Serrano and P. Pizarro, Hydrodeoxygenation of anisole as bio-oil model compound over supported Ni and Co catalysts: Effect of metal and support properties, Catal. Today, 2015, 243, 163–172 CrossRef CAS.
- M. Pisarek, M. Lukaszewski, P. Winiarek, P. Kedzierzawski and M. Janikczachor, Influence of Cr addition to RANEY® catalyst on hydrogenation of isophorone, Catal. Commun., 2008, 10(2), 213–216 CrossRef CAS.
-
(a) A. Perrard, P. Gallezot, J.-P. Joly, R. Durand, C. Baljou, B. Coq and P. Trens, Highly efficient metal catalysts supported on activated carbon cloths: A catalytic application for the hydrogenation of D-glucose to D-sorbitol, Appl. Catal., A, 2007, 331, 100–104 CrossRef CAS;
(b) H. Hofmann, DipLChem and W. Bill, Geschwindigkeitsbestirnrnende Faktoren bei Reaktionen mit suspendiertem Kontakt, Chem. Ing. Tech., 1959, 31(2), 81–88 CrossRef CAS;
(c) J. Wlsnlak and P. Alfandary, Sperm Whale Oil Replacements from Halogenation of Jojoba Oil, Ind. Eng. Chem. Prod. Res. Dev., 1979, 18(4), 358–364 CrossRef.
- M. G. Al-Shaal, W. Ciptonugroho, F. J. Holzhäuser, J. B. Mensah, P. J. C. Hausoul and R. Palkovits, Catalytic upgrading of α-angelica lactone to levulinic acid esters under mild conditions over heterogeneous catalysts, Catal. Sci. Technol., 2015, 5(12), 5168–5173 CAS.
- T. J. Bruno, A. Wolk and A. Naydich, Stabilization of Biodiesel Fuel at Elevated Temperature with Hydrogen Donors: Evaluation with the Advanced Distillation Curve Method, Energy Fuels, 2009, 23, 1015–1023 CrossRef CAS.
-
(a) E. M. Yoon, L. Selvaraj, C. Song, J. B. Stallman and M. M. Coleman, High-Temperature Stabilizers for Jet Fuels and Similar Hydrocarbon Mixtures. 1. Comparative Studies of Hydrogen Donors, Energy Fuels, 1996, 10, 806–811 CrossRef CAS;
(b) E. M. Yoon, L. Selvaraj, S. Eser and M. M. Coleman, High-Temperature Stabilizers for Jet Fuels and Similar Hydrocarbon Mixtures. 2. Kinetic Studies, Energy Fuels, 1996, 10, 812–815 CrossRef CAS.
- W. E. Hanford, US 2,209,055, United States Patent Office, 1940.
-
(a) C. B. Rodella, G. Kellermann, M. S. P. Francisco, M. H. Jorda and D. Zanchet, Textural and Structural Analyses of Industrial RANEY® Catalyst, Ind. Eng. Chem. Res., 2008, 47, 8612–8618 CrossRef CAS;
(b) H. Lei, Z. Song, D. Tan and X. Bao, Preparation of novel RANEY® catalysts and characterization by XRD, SEM and XPS, Appl. Catal., A, 2001, 214, 69–76 CrossRef CAS.
Footnote |
† Electronic supplementary information (ESI) available. See DOI: 10.1039/c6ra14625b |
|
This journal is © The Royal Society of Chemistry 2016 |