DOI:
10.1039/C6RA14457H
(Paper)
RSC Adv., 2016,
6, 70913-70924
Plasma modification of the electronic and magnetic properties of vertically aligned bi-/tri-layered graphene nanoflakes†
Received
3rd June 2016
, Accepted 20th July 2016
First published on 21st July 2016
Abstract
Saturation magnetization (Ms) of pristine bi-/tri-layered graphene (denoted as – FLG) is enhanced by over four (4) and thirty-four (34) times to 13.94 × 10−4 and 118.62 × 10−4 emu g−1, respectively, as compared to pristine FLGs (Ms of 3.47 × 10−4 emu g−1), via plasma-based-hydrogenation (known as graphone) and nitrogenation (known as N-graphene) reactions, respectively. However, upon organo-silane treatment on FLG (known as siliphene), the saturation magnetization is reduced by over thirty (30) times to 0.11 × 10−4 emu g−1, as compared to pristine FLG. Synchrotron based X-ray absorption near edge structure spectroscopy measurements have been carried out to investigate the electronic structure and the underlying mechanism responsible for the variation of magnetic properties. For graphone, the free spin available via the conversion of the sp2 → sp3 hybridized structure and the possibility of unpaired electrons from induced defects are the likely mechanism for ferromagnetic ordering. During nitrogenation, the Fermi level of FLGs is shifted upwards due to the formation of a graphitic like extra π-electron that makes the structure electron-rich, thereby, enhancing the magnetic coupling between magnetic moments. On the other hand, during the formation of siliphene, substitution of the C-atom in FLG by a Si-atom occurs and relaxes out the graphene plane to form Si–C tetrahedral sp3-bonding with a non-magnetic atomic arrangement showing no spin polarization phenomena and thereby reducing the magnetization. Thus, plasma functionalization offers a simple yet facile route to control the magnetic properties of the graphene systems and has potential implications for spintronic applications.
Introduction
Graphene is a single atomic layer of sp2 hybridized carbon atoms covalently bonded to three other atoms arranged in a honeycomb lattice with a thickness of only 0.34 nm.1–7 Its unique structural, mechanical and electrical properties with high carrier mobility make it one of the most important topics in advanced materials science of the present era.8–14 Due to its unique properties, a variety of applications have been proposed and indeed reported in the literature including chemical sensors,15,16 nano-electronics devices,17 hydrogen storage systems18 and polymer nano-composites.19 Especially, graphene-based spintronics and magnetic materials are envisaged to be highly promising due to their extraordinary carrier mobility and may provide an easy way to integrate spin and molecular electronics.1 The long spin diffusion length of graphene makes it highly attractive for spintronic devices and has triggered a quest for integrating the charge and spin degrees of freedom.1 However, graphene is intrinsically non-magnetic and lacks localized magnetic moment due to the delocalized π-bonding network, which limits its applications in spintronic devices.20 Therefore, synthesis of ferromagnetic graphene or its derivatives with high magnetization is necessary for spintronics applications. For the synthesis of ferromagnetic graphene, dopants like hydrogen, nitrogen, boron, silicon, sulphur, selenium and/or different transitions metals have been explored with varying degrees of success.
One important development since the discovery of graphene has been the discovery of the so-called graphone and graphane, which are partially/fully hydrogenated forms of graphene and were first theoretically reported by Zhou et al.21 and Lu et al.,22 respectively. In graphone/graphane form, the carbon atoms are sp3 hybridized making them particularly suitable for spintronic applications. The predicted graphone/graphane structures were later confirmed experimentally by Ray et al.23 and Elias et al.24 by exposing graphene to hydrogen plasma (ranging from a few minutes to several hours) and it was observed that the magnetic moment of plasma functionalised graphene is enhanced dramatically. Graphane is predicted to have a stable structure with a band gap of 3.5 eV, consisting of a graphene layer in which each of the C atom is sp3-bonded to one H atom above and below in an alternating manner;25 whereas graphone (one sided hydrogenation of graphene) has sp3 bonding of C with H on only one side of the graphene and is useful in electrical isolation for graphene-based circuits.26 The nitrogen-doping and/or N-functionalization of graphene has also been shown as an effective route to obtain high magnetization values owing to its comparable atomic size to carbon and the presence of five valence bonds with carbon atoms,27 which can introduce magnetic moment into graphene.28 Theoretical studies too have confirmed that the N-doping is an effective method to introduce magnetic moments into graphene.28–32 These localized magnetic moments can be induced by different arrangement of N atoms with the pyridine and pyrrole like N-doping defects breaking the degeneracy of the spin polarisation of graphene.28–32 Indeed, Liu et al.33 have observed high values of magnetization in ferromagnetic graphene oxide (GO), when doped with nitrogen. Post N-doping, the Fermi level shifts upwards due to presence of extra π-electrons which makes graphene electron-rich34 thereby enhancing the coupling via the reduction of the magnetic moment distance.33 Zhang et al.31 proposed that the combination of vacancy defects and N atoms may provide a unique way for enhancing the magnetic moment of graphene. The silicon atoms, which are introduced into the graphene structure as heteroatoms, can also tune the magnetic and electronic properties of graphene.35 Needless to say, the implementation of Si-doping in graphene requires a clear understanding of the effect of silicon atoms on graphene for the fabrication of various electronic and magnetic devices. It was experimentally observed by Pao et al.35 that threefold-coordinated Si in graphene results in sp3 hybridization. Besides, a single Si-impurity in monolayer graphene and Si-doped bilayer-graphene have also been investigated, which shows the existence of covalent bonding between the Si atoms of different layers.36 This stable linked structure strongly suggests the importance of interaction between Si-impurity atoms in graphene. However, unlike the hydrogen and nitrogen doping of graphene, there are only a handful of experimental studies on the electronic and magnetic properties of Si-doped/functionalized graphene. Particularly, there are no reported studies on the magnetic properties of Si-doped graphene, thereby necessitating exploration of this graphene derived material (Si-doped graphene) for practical applications as a diluted magnetic semiconductor (DMSs) in spintronics.37
In our earlier work, we had reported on the synthesis of hydrogen-functionalized graphene (referred as graphone/graphane) on graphene layer (referred as graphene supported graphone/graphane bi-layer nano-structure materials) and found that the magnetic moment is enhanced significantly with potential implications for their use in spintronic applications.23 We also reported that the GO shows higher ferromagnetism than reduced graphene oxide (rGO), and the origin of ferromagnetism was identified as the C-2p(σ*)-derived states that involve defects/vacancies rather than C-2p(π*) states that are bound with oxygen-containing and hydroxyl groups on GO-sheets; which could also be potentially used in spintronic applications.38 In the present study, we have further investigated the significant enhancement of magnetic properties of FLGs upon doping by nitrogen plasma (referred as N-graphene). We have also studied the magnetic effect of organo-silane-treated (Si-doped/functionalized) graphene (referred as siliphene) and compared with graphone and N-graphene for the possibility of use in spintronic applications. The magnetic properties were studied by a superconducting quantum interference device (SQUID)-type magnetometer and magnetic force microscopy (MFM) measurements. The electronic structure and microstructural properties were correlated with magnetic properties using a variety of techniques including X-ray Absorption Near Edge Structure (XANES), X-ray Photoelectron Spectroscopy (XPS), Raman spectroscopy and field emission analysis among others.
Experimental
Preparation of few layer graphene (FLG), graphone, N-graphene and siliphene
The synthesis of FLG was carried out in a SEKI microwave plasma enhanced chemical vapor deposition system, equipped with a 1.5 kW, 2.45 GHz microwave source. The substrates used were bare n-type heavily doped Si wafers (resistivity < 0.005 Ω cm−1) (10 mm × 10 mm). Prior to growth, the substrates were pre-treated with N2 plasma at 650 W at 40 Torr while the substrate temperature was maintained at 900 °C. Synthesis was then carried out using CH4/N2 (gas flow ratio = 1
:
4) plasma at 800 W for a duration of 60 s. The FLG were allowed to cool under a constant N2 flow. The conditions used were similar to the ones reported in our previous publications.48,49,88–90 The hydrogen microwave plasma treatment of the FLG was carried out at substrate temperatures of 50 °C (referred as graphone) at a chamber pressure of ∼2 Torr with a treatment time of 90 s and microwave power of 150 W.23 Nitrogen doping/functionalization of FLG (referred as N-graphene) was carried out using an Electron Cyclotron Resonance (ECR) nitrogen plasma treatment for 5 min;48 whereas Si is doped/functionalized (referred as siliphene) using RF-plasma glow discharge process with specific Ar/tetra-methyl-silane [TMS, Si(CH3)4] ratio at room temperature for 5 min.35
Material characterization
Raman spectroscopy was performed using an ISA LabRam system equipped with a 632.8 nm He–Ne laser with a spot size of approximately 2–3 mm, yielding a spectral resolution of better than 2 cm−1. Due care was given to minimize sample heating by using a low laser power below 2 mW. The XPS spectrum was measured on a Kratos Axis Supra DLD employing an Al Kα radiation (1486.6 eV). The X-ray absorption near edge structure (XANES) spectra was obtained using the high-energy spherical grating monochromator 20A-beamline at the National Synchrotron Radiation Research Center (NSRRC), Hsinchu, Taiwan. The magnetic properties of these FLGs were characterized by a SQUID-type magnetometer with sensitivity better than 5 × 10−8 emu. The topographical and magnetic force microscopy (MFM) measurements were carried out using a Veeco Dimension 3100 AFM connected to a Nanoscope IIIa controller in the tapping mode configuration. To detect magnetic domains in the prepared samples, low moment magnetic probes with Co/Cr coatings were used. In addition, the electron field emission (EFE) was measured using a Keithley source meter.
Results and discussion
The scanning electron microscopy (SEM) images of all the FLG samples are shown in Fig. 1(a–d) respectively. It is evident that the as-synthesized, pristine FLGs are vertically aligned to the underlying Si substrate and are randomly intercalated with each other to form a porous mesh-like network.23 The post-growth H2 and N2 plasma treatment processes does not disturb the vertically aligned nature of the graphene platelets; however, the plasma treatment does lead to an increase in the number of sharp graphene edges throughout the graphone and N-graphene samples. It is also found that the apparent thickness of the edges of graphene platelets is reduced due to plasma etching effects as reported in our previous work.23 However, for organo-silane-treated FLGs (siliphene), a significant increase in the thickness of FLG via the formation of round-shaped, rod- and tube-like structures was observed in the SEM image (Fig. 1(d)). Fig. 1(e) shows the plot for EFE current density (J) as a function of the applied electric field (EA) for graphene, graphone, N-graphene and siliphene. The figure shows the existence of a classical threshold electric field at which the current density, J increases significantly from a zero value. As compared to pristine FLGs, the threshold electric field is reduced for N-graphene whereas for graphone and siliphene, the threshold value increases, which suggests that the H and Si treatment increases the activation energy whereas the N treatment reduces the activation energy. The Fowler–Nordheim (F–N) plots shown in Fig. 1(f) clearly show the existence of threshold electric field or turn-on electric field (ETOE) which was measured by linear fitting of the curve in the high electric field region. For pristine FLGs, the ETOE value were 26.5 V μm−1, which was further increased to 29.3 (36.5) V μm−1 for graphone (siliphene) by the increase in the sp3-hybridized bonds in graphone/siliphine.23,35,39 In the case of N-graphene, the ETOE decreases to 20.0 V μm−1 due to the increase in the number of defect sites, lowering of the Fermi level and the possible enhancement of the sp2 bonding, as observed by Chiou et al.40 in nitrogen functionalized graphene nanoflakes. In the case of H and Si functionalised FLGs, the strong preference for sp3 bonding has been argued to be related to the outward relaxation of the H and Si atoms.23,35,39 The sp2 hybridised bonds as well as the upwards movement of the Fermi level in the N-graphene network are responsible for the enhancement of electron field emission current whereas the H, Si doping induced sp3 bonding configuration leads to the reduction of field emission current due to the reduction in the metallic character. Therefore, reduction of the EFE current in graphone/siliphene is due to the decrease of the number of sp2 hybridized bonds in the network which are responsible for the metallic EFE current.41,42 These results clearly indicate that H- and Si-doping promote the three-dimensional sp3 bonding configuration; whereas N-doping promotes sp2 bonding configuration.41,42
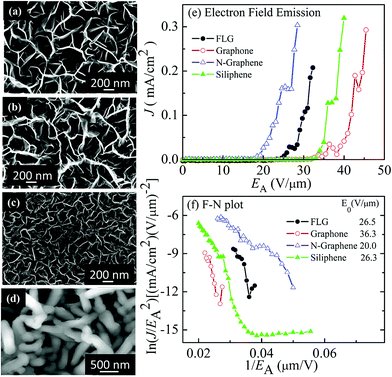 |
| Fig. 1 SEM images of (a) pristine FLGs, (b) graphone, (c) N-graphene and (d) siliphene. The (e) electron field emission behaviour showing current density (J) as a function of applied electric field (EA) and (f) Fowler–Nordheim plot obtained from EFE measurements. (a and b) Reproduced from S. C. Ray et al., Sci. Rep., 2014, 4, 3862. | |
Raman spectroscopy
Fig. 2 shows the deconvoluted Raman spectra of pristine FLGs along with graphone, N-graphene and siliphene in the range of 1200–3000 cm−1, obtained using a laser excitation of wavelength 632.8 nm. The main Raman spectra features of carbon-based materials are the G and D bands that lie around 1580 and 1350 cm−1, respectively. While, the G band corresponds to optical E2g phonons at the Brillouin zone centre; the D peak arises due to the breathing-like modes (corresponding to TO phonons close to the K point) and requires a defect for its activation via an inter-valley double resonance Raman process. Moreover, the intensity of the D-peak provides a simple measure of the amount of disorder in graphene and graphene based structures. The overtone, 2D band appears at around 2680 cm−1, being the sum of two phonons with opposite momentum, acts as a fingerprint for monolayer graphene and is present even in the absence of any defects.43–47 The D′ peak observed at ∼1620 cm−1, occurs via an intra-valley double resonance process in the presence of defects. Since the D band requires a defect to be Raman active, it is generally not used to characterize doping. However, both the G and 2D bands are both strongly influenced by the carrier concentration and they have been extensively studied for doping characterization.43–47 Detailed analysis of band shape analysis of the Raman bands was carried out by undertaking analysis of the band shape and full width at half-maximum (FWHM) parameters by fitting the first and second order Raman spectra using Lorentzian peak shapes for the D, G, and G′(2D) bands, and Gaussian peak for the D′ band. This is in accordance with our previous studies carried out on few layered graphene systems.23,48,49 As compared to the peak position (1580.5 cm−1) and the FWHM (23.4 cm−1) of the G band in pristine FLGs; upon nitrogen doping, the G band upshifted slightly to 1581.5 cm−1 with a corresponding increase in FWHM to 24.5 cm−1. It should be noted that although the G band peak shifts are more prominent in electrostatically gated monolayered graphene, nevertheless, similar stiffening of the G band along with FWHM enhancement has been previously observed in chemically doped graphene.48,50,51 This blue shift of the G band (E2g mode at Γ) along with the associated broadening of FWHM has been attributed to the nonadiabatic removal of the Kohn anomaly from the Brillouin zone center, Γ.48,50,51 The electron-cyclotron resonance plasma treatment induces the substitution of electron-donating nitrogen atoms into the graphene lattice, with the overall effect of a rise in the Fermi level that is then observed in the blue shift of the G band in Raman spectra.48 The incorporation of N into the FLG structure may generate C–N and N–N bonds at the expense of the C–C bonds. However, the C–N vibration modes, which lie between the G and D bands, cannot be identified owing to the insensitivity of Raman excitation to distinguish between the cross sections of C and N atoms. In electric field gated graphene, by applying suitable bias, both electron and hole conduction mechanisms are accessible by shifting the Fermi level and can be observed in the upshift of the G peak in the Raman spectra for both the cases.51 Similarly, the G′(2D) band shows an upshift from 2661.4 cm−1 to 2663.1 cm−1, with a slight increase in the FWHM from 43.7 cm−1 to 44.3 cm−1. This blue shift of the 2D band has also been observed by Yan et al., and has been attributed to the increased disorder and reduction in the crystallinity in N-doped graphene and is consistent with those observed in electrostatically gated graphene.51,52 An I2D/IG ratio of >1 is indicative of the formation of bi-layer/tri-layer graphene, whereas an I2D/IG ratio of <1 represents tri- or multi-layered graphene.53,54 It is also observed that the G-band shifts on hydrogenation and organo-silane treatment too which is caused by a change in charge density55 and moves the Fermi level of FLG. Correspondingly, upon hydrogenation, the G band downshifted to 1579.1 cm−1 with a lowering of FWHM to 21.5 cm−1. When hydrogenated, the graphone samples are expected to be electron doped as carbon is slightly more electronegative than hydrogen and is corroborated by the red shift observed in the G band position.55 The G′(2D) spectra of graphone samples shows a significant downshift from 2661.4 cm−1 to 2657.9 cm−1 with a slight broadening of the line width from 43.7 cm−1 to 44.4 cm−1. This is accompanied by the change in the ID/IG (I2D/IG ratio) ratio to 0.32 (1.03) from 0.83 (1.02) for pristine FLGs, and as mentioned earlier signifies the formation of bi/tri-layered graphene. A similar reduction in the graphene layers upon plasma hydrogenation process has been observed previously as well and is corroborated by both the values of I2D/IG and the FWHM of the 2D band.56 In the recent study carried out by Eckmann et al., the ratio of D and D′ bands was used to comment upon the nature of defects induced in graphene through fluorination and Ar+ bombardment.57 In their study it was observed that the ID/ID′ ratio was maximum (∼13) for sp3 type defects and reaches a minimum (∼3.5) for boundaries in graphite.57 In our study, the ID/ID′ ratio varied from 3.03 for pristine FLGs vs. 3.23 for FLG:N and 5.5 for the FLG:H samples. The rather high value of ID/ID′ for the pristine samples can be attributed to the finite crystallite size and hence the presence of boundaries, the combination of which leads to the defect induced D peak as well. While both the N-graphene and graphone samples were prepared using plasma modification, the differences in the working pressures as well as the power leads to significant differences in the formation of defects. For graphone samples, the increase in the ID/ID′ ratio can be attributed to the formation of sp3 bonds as well as the vacancy-like defects produced due to H+ bombardment in the microwave plasma.23 While the N+ ion bombardment too will produce substitutional and vacancy-like defects in the graphene lattice, the electron-cyclotron resonance conditions used for functionalisation limits the amount of defects produced in the graphene lattice due to the lower ion energy of the species.48 Similarly, upon organo-silane treatment, the ID/ID′ ratio increased to 3.30 from 3.03 for pristine FLGs which again can be attributed to the formation of sp3 type defects and structures for FLG:Si samples. From the Raman spectrum of siliphene, it can be observed that there are no significant SiC segregations or clusters in siliphene. As compared to pristine FLGs, both the D and G bands for siliphene are upshifted to 1333.0 and 1581.3 cm−1, respectively accompanied by the broadening of D (45.1 cm−1 from 30.7 cm−1 for pristine FLG) and G bands (24.4 cm−1 from 23.4 cm−1 for pristine FLG). This broadening of the bands can be attributed to the formation of large number of sp3-hybridized atoms in siliphene after the weakening of the C–C bond caused due to bonding with the Si-atom. The consequential reduction of the ID/IG ratio (a sp2/sp3 signature) upon functionalization with Si [FLG:0.80 → siliphene:0.39] also indicates the formation of large number of sp3-hybridized atoms via a partial conversion of the sp2 bonding, which is a mixture of single and double C–C bonds, to the sp3 bonding with only single C–C bonds. The G′(2D) band for siliphene samples does not show any appreciable movement, however a significant increase in the FWHM of the 2D band is observed wherein it increases from 43.7 cm−1 to nearly 51.6 cm−1 accompanied by a reduction in the I2D/IG ratio to 0.49 from 1.03 for pristine FLGs. From the SEM image of Fig. 1(d), it can be clearly observed that the organo-silane treatment clearly leads to an increase in the edge thickness of the flakes which thereby leads to the formation of multi-layer graphene like structures. Since the FLG are vertically aligned on a Si-substrate, it is expected that only
the top-most surfaces of the FLG would be predominantly accessible to atomic hydrogen/nitrogen/silicon during the functionalization procedure.
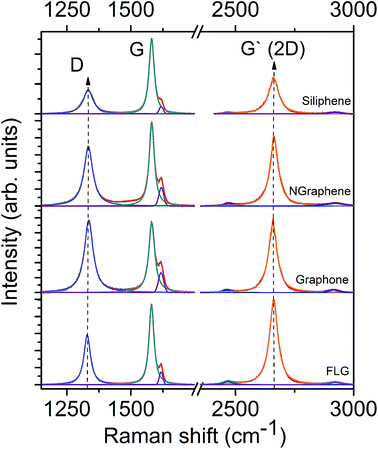 |
| Fig. 2 Raman spectra of pristine and functionalised FLG samples showing the changes occuring in the peak positions of the D and G′ bands. | |
XANES and XPS analysis
For graphitic materials in general, the X-ray absorption near edge structure (XANES) spectra can be divided into three regions characterized by specific resonance energies.58 The first region of π* resonance appears around 285 ± 1 eV, the C–H* resonance around 288 ± 1 eV, and a broad region between 290 and 315 eV corresponds to σ* resonance. The presence of the π* and C–H* resonances serve as a fingerprint for the existence of sp2 hybridized C–C bonds and C–H bonds, respectively. Fig. 3 shows the C K-edge XANES spectra of the FLGs with spectral features at ∼285.1 (±1) eV, ∼292.6 (±1) eV and ∼291.6 (±1) eV; which are attributed to the unoccupied 1s → π*, 1s → σ* and excitonic states transitions, respectively.58 The peak positions for graphone are similar to that of pristine FLG, but the absorption edges of N-graphene/siliphene are shifted towards higher/lower energy levels that can be seen clearly from the first order derivatives of all the C K-edge XANES spectra shown in S1 (ESI†). This change in the absorption edge is attributed to the change in the band gap of N-graphene/siliphene due to the structural rearrangement via carbon bonding with nitrogen and silicon, respectively.59,60 Apart from the π* and σ* resonance peaks, two other peaks at ∼287.4 (±1) eV and ∼288.5 (±1) eV are observed and are ascribed as signatures of C–H bonds and interlayer graphite states, respectively (also shown inset below in Fig. 3). When compared to the pristine FLG spectra, the graphone spectra show an increased intensity of the C–H peak accompanied by a reduction in the interlayer graphite peak intensity. This increase in the C–H peak intensity confirms the formation of sp3-rich structures having a higher content of C–H bonds. In the case of N-graphene, the C K-edge XANES spectrum shows a strong peak at 0.2 eV below the interlayer graphite peak (marked with a line bar), which may arise from the contribution of N- and O-related bonds in N-graphene. The Si-functionalized C K-edge XANES spectra show that both the π* and σ* peaks are wider and show a downward energy shift which is different from graphone/N-graphene. A similar downward energy shift has also been observed in Si-treated diamond-like carbon thin films59 indicating the change of band gap of the material. The wide π* region may be double features due to the possibility of the formation of two different kinds of bonds, namely, Si–C(:H)/Si–C(:O) and sp2 C–C bonds, which were also observed by Terekhov et al. in their XANES study of a-Si0.4C0.6:H composite materials.60
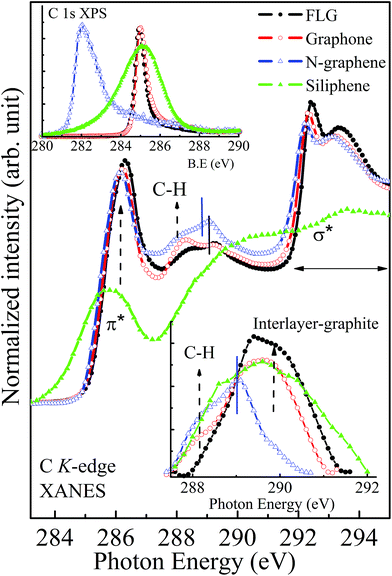 |
| Fig. 3 C K-edge XANES spectra of the pristine and plasma functionalised FLGs with the lower inset showing the signatures of C–H bonds and interlayer graphite states. The inset above shows the changes in the C 1s core level XPS spectra. | |
The general line shape of the C K-edge XANES spectrum of SiC/β-SiC60,61 is clearly very different from that of siliphene, which indicates the absence of SiC segregations in siliphene. The π* feature becomes wider for siliphene and similar broadening has also been observed in CVD diamond (see (ref. 5), Fig. 6). On comparison with the spectral features of the CVD diamond,60,61 the siliphene can be concluded to contain an increased number of sp3-hybridized atoms with diamond-like character. Again, the interlayer graphitic peak is not observed in the case of siliphene like graphone/N-graphene, thus, there is a possibility of the formation of the Si–C(:O) bonding in addition to the Si–C(:H) bonding. Inset above in Fig. 3 shows the C 1s core-level XPS spectra. The peak observed at ∼285.0 eV for both FLGs and graphone can be assigned as the C–C bond, which is shifted to the higher energy at ∼285.2 eV and became broader for siliphene. Pedio et al.62 studied the dependence of the formation of C60 on silicon surfaces on the annealing temperature using C 1s XPS measurements and found that the C–C bond and C–Si bond features are located at 284.2 and 282.6 eV, respectively. In the present case, the spectral features are different and apparently do not have the signature of SiC segregations in C 1s core-level XPS spectra, which further confirms enhanced sp3 bonding (i.e., diamond and/or diamond-like carbon) rather than sp2 bonding (like graphite and/or CNTs) and/or C–Si bonding. This result is consistent with those obtained from Raman and XANES measurements. The enhancement of Si–C tetrahedral bonding (sp3 bonding) and reduction of sp2 bonding improves the reactivity of siliphene, as the surface atoms with sp3 bonding have dangling bonds. In the case of N-graphene, the C 1s peak position is shifted to a lower energy level at ∼282.1 eV indicating the formation of a more graphitic structure and probable with the formation of nitrogen bonding in N-graphene. Moreover for detailed analysis about the presence of nitrogen-bonding and Si-bonding with C in N-graphene/siliphene, we have also studied the N K-edge (Si L-edge) XANES and N 1s (Si-2p–2s) XPS spectra, the results of which are discussed below.
Fig. 4 shows the N K-edge (Si L-edge) XANES and N 1s (Si-2p–2s) XPS spectra of N-graphene/siliphene. The π* region of N K-edge XANES spectrum was deconvoluted into three components, while the N 1s XPS spectra was deconvoluted into two components using Gaussian lineshapes; which are shown in Fig. 4(a and c), respectively. The four peaks in the N K-edge XANES spectrum are located at ∼396.7, 397.7, 398.7 and 399.9 eV which can be attributed to nitrogen in nitride phase, pyridine-like bonding and pyrrole/substitutional graphite like bonding.63 Similar peak positions have been observed by Geng et al. in their study of nitrogen doping effects on the structure of graphene. The π* resonance peak at 397.7 eV is attributed to pyridine-like bonding and arises from transitions from the K-shell (N 1s) to the unoccupied π* orbital.64,65 The two peaks observed in the N 1s XPS spectrum are at ∼395.7 and 397.4 eV and can be ascribed to nitride-like species or aromatic N-imines and pyridine like species66 and is corroborated by N 1s XANES spectra. The peak position of the pyridine like species is however 1.0 eV lower to that reported by Liu et al. in the case of nitrogen doped graphene oxide.33 For siliphene, the Si L-edge spectrum is composed of four Gaussian peaks at 104.7 (I), 106.2 (II), 108.0 (III) and 108.5 eV (IV). These peaks are mainly Si 2p → 3s transition (peak I and II), split into L2,3-peak, and Si 2p → 3p/3d transitions (peak III and IV) as observed by Gaultois et al. in (TiO2)x(SiO2)1−x composite,67 Li et al. in quartz68 and Tsai et al. in SiO2 materials.69 The curve fitting of the Si 2p XPS was carried out using four Gaussian peaks at 101.6 (I), 103.0 (II), 104.2 (III) and 105.6 eV (IV), corresponding to Si–C, Si–C(:O)/Si–C(:H), Si–O and SiOx species respectively,35,70,71 although we have not observed any prominent Si–C peak in XANES spectrum. The results match very well with those observed for SiC thin films prepared using chemical vapor deposition method.71 While the Si incorporation occurs in the graphene lattice to form sp3-hybridized atoms or tetrahedral bonding with diamond-like character; the post functionalisation exposure of the samples to the ambient environment leads to the formation of Si–C(:O)/Si–C(:H) bonding. The Si 2s XPS decomposed into three peaks viz. 151.6 (I), 153.7 (II) and 156.4 (III) and ascribed as Si–Si (I) and Si–O (II & III).70
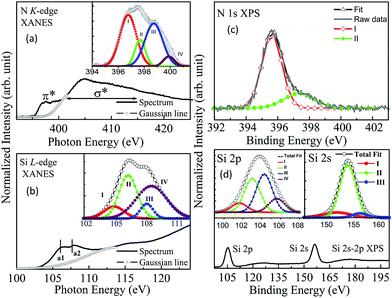 |
| Fig. 4 (a) N K-edge XANES spectra of N-graphene with its deconvolution in the inset, (b) Si L-edge XANES spectra of siliphene with its corresponding deconvolution in the inset, (c) N 1s XPS spectra of N-graphene with its deconvolution and (d) Si 2p–Si 2s XPS spectra of siliphene with their corresponding deconvolution in the inset. | |
Magnetic measurements
Now, the magnetic properties of the FLG and graphone/N-graphene/siliphene were measured within the range of −2 kOe < H < 2 kOe at temperatures of 300 K and 40 K, respectively. The measured magnetic hysteresis loops are shown in Fig. 5, with the N-graphene (Fig. 5(c)) showing the most expressed ferromagnetic behaviour with lowest field hysteretic features (Hr = 40 Oe) and highest saturation moment (Ms = 118.62 × 10−4 emu g−1), whereas the siliphene shows the highest hysteretic features (Hr = 120.03 Oe) and the lowest saturation moment (Ms = 0.11 × 10−4 emu g−1). As compared to the pristine FLGs (Ms = 3.47 × 10−4 emu g−1), the magnetic moment values of graphone (Fig. 5(b)) is higher (Ms = 13.94 × 10−4 emu g−1) due to the incorporation of hydrogen in the FLG as observed in C K-edge XANES spectrum, resulting in the formation of sp3 hybridized carbon structure through mono and possible di-hydrogen termination. Similar increase in the magnetic properties of graphene exposed to hydrogen has been reported recently by Herrero et al., wherein they deposited a single hydrogen atom on top of graphene and then utilised scanning tunnelling microscopy to detect magnetism on the sublattice lacking the deposited atom.72 In the case of siliphene, the spectral feature is entirely different than graphone/N-graphene and is considered as mixed dia- and ferro-magnetic in nature as shown in S2 (ESI†). Due to presence of mixed dia- and ferro-magnetic nature, we have subtracted the diamagnetic background revealing that the saturation magnetic moment is ∼0.11 × 10−4 emu g−1 only, significantly lower as compared to graphone/N-graphene and even lower than pristine FLGs (Ms = 3.47 emu g−1) as shown in Fig. 5(d). As the FLG are free from any catalyst remnants (ESI, Fig. S3†) and detectable foreign magnetic impurities, the observed magnetism can be attributed to the defects and vacancies created during synthesis, and the creation of sp3 hybridised structures.73–75 Previously, He et al., reported that the magnetisation of the 12C+ implanted highly oriented pyrolytic graphite (HOPG) was found to be closely correlated with the density of defects and an almost linear relationship between the ID/IG ratio and saturation magnetisation was observed.76 As the N-graphene shows the highest ID/IG ratio, it is expected and indeed observed that the N-graphene displays highest magnetization value, followed by the graphone.
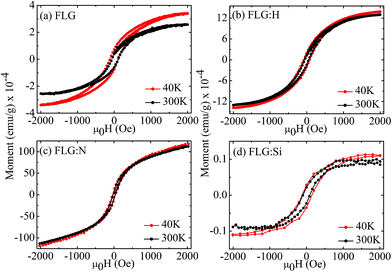 |
| Fig. 5 Magnetic hysteresis loops obtained for (a) FLG, (b) graphone, (c) N-graphene and (d) siliphene at 300 K and 40 K, respectively. | |
Even though the nitrogenation33 and hydrogenation23,77 may possibly occur on only the top-most surface layers of the FLG; thereby favouring the higher observed saturation magnetic moment compared to pure FLG; but their inherent mechanisms are different to each other. In the case of hydrogenation, the magnetism is due to the formation of sp3-rich hybridized structure thereby favouring ferromagnetism, as reported in our earlier work23 and similar to those reported earlier;78 whereas, for nitrogenation the magnetism is due to the extra π-electron from nitrogen making the structure electron-rich, thereby, enhancing the magnetic coupling between magnetic moments.33 In their recent work, Miao et al. observed enhanced ferromagnetism in N-doped graphene with an increase in the saturation magnetisation and coercive field with an increase in the nitrogen content, especially pyrrolic groups of the samples which can induce a net magnetic moment of 0.95 μB/N.79 Based on different hydrogen attachment configurations on graphene, Yazyev et al. predicted80 that the ortho-dimers and para-dimers are nonmagnetic, while single hydrogen attachment (monomer) are magnetic in nature.80 This may explain why graphone is more magnetic than FLG. Wang et al.81 proposed long-range coupling of spin units (defects) to be responsible for ferromagnetism in graphone whereas Xie et al.,82 for partially hydrogenated graphene, have invoked the formation of unpaired electrons in graphene which together with the remnant delocalised π bonding network introduces the observe ferromagnetism. In the case of N-graphene, the Fermi level shifts upwards due to the extra π electron that makes graphene electron-rich.34 The shift of C K-edge and formation of different carbon–nitrogen bonds observed in the N K-edge XANES spectra clearly indicated the formation of π electron and Fermi level shift. The high value of ID/IG ratio of N-graphene also implies defect rich N-graphene. This result suggested that the magnetic properties of the super lattices were entirely determined by the graphitic region due to the π character of the spin density. Thus, N-doping enhances the direct type of magnetic coupling between the magnetic moments due to the decrease in the distance between the magnetic moments. Moreover, the presence of magnetic exchange or coupling between the localized magnetic moments is a necessary ingredient for the magnetic cooperative behaviour such as ferromagnetic ordering. It is noted that the specific contribution made by each specific type of two N types (pyridine-like and cyanide-like) is complicated, and it is difficult to clarify the specific contribution in N-graphene. Interestingly, the nitrogen doping (N-graphene) increases the magnetization by almost 34 times as compared to pristine FLGs. Similar enhancement of magnetisation (∼15 times higher) was also observed by Liu et al.33 in their study of nitrogen doped GO. So, it is very clear from this study that N-doping result in the increase of the magnetization of FLG and the generation of ferromagnetism. In the case of siliphene, it is observed from the C K-edge XANES spectra that the sample contains an increased number of sp3-hybridized atoms or tetrahedral bonding with diamond-like character with possibility of the formation of the Si–C(:O)/Si–C(:H) bonding. The Si–C/Si–O bonding is observed in the Si L-edge XANES as well as the Si 2p–2s XPS spectrum. As compared to pristine FLG, the band gap of siliphene is enhanced. As pure SiC is a direct band gap semiconductor material, the conduction band bottom and valence band maximum are located at Brillouin zone Γ-point and have no phenomena of spin polarization; thereby non-defective tetrahedral bonding SiC has no magnetism.81 Hence, the magnetization is reduced after formation of SiC and SiO in the siliphene structure.
MFM measurements
Further detection of the magnetic domains in the pristine and graphone/N-graphene/siliphene was carried out by magnetic force microscopy (MFM) measurements using low moment magnetic probes with Co/Cr coating. Fig. 6 and 7 show the topographic (height), amplitude and phase signals imaged simultaneously for both tapping mode AFM (TM-AFM) and magnetic force microscopy (MFM) mode to assess the correlation of surface features, identify and eliminate possible artifacts and to assess the effects of functionalization on magnetization. The magnetized Co/Cr coated probe interacts with magnetic field gradients generated by magnetic domains within the synthesised FLGs resulting in changes in the phase and amplitude of the oscillating cantilever. To assess the correlation of surface features and the effects of magnetization, the topographic (height), amplitude and phase signals were imaged simultaneously for both conventional topographical imaging and magnetic measurements. MFM data were acquired while maintaining a constant “lift scan height” of ∼10 nm above the topography (height) data to reduce coupling between van der Waals and magnetic forces and also to demonstrate the field strength generated by the magnetic domains. In the “lift-scan” mode, the topography is measured in the dynamic amplitude modulation mode after which the tip is then moved at a constant distance above the surface during which the magnetic component of the data is recorded.23 In theory, the topographic contributions should get eliminated in the second image. Comparing the AFM scans of the samples with the MFM scans, while no significant changes are observed in the topographic signal; significant differences are observed in the phase and amplitude scans. For the conventional AFM scans, the phase signal is essentially a map of how the phase of the cantilever oscillation is affected by its interaction with the sample surface and is affected highly by topography among other factors such as chemical nature, relative hardness/softness of the sample. Since the MFM signal represents the phase shift between the probe oscillation and the driving signal due to magnetic force acting on the tip, therefore by visualising/measuring the changes occurring in the amplitude and phase images, the existence of magnetic domains in the samples can be ascertained.
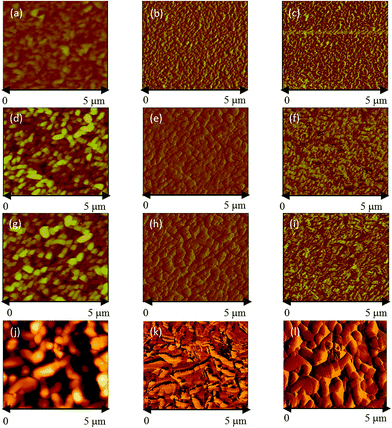 |
| Fig. 6 Atomic Force Microscopy (topography, phase, amplitude) images of pristine FLG (a–c), graphone (d–f), N-graphene (g–i) and siliphene (j–l). | |
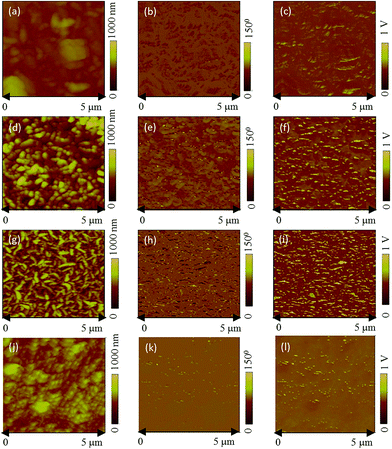 |
| Fig. 7 Magnetic Force Microscopy (topography, phase, amplitude) images of pristine FLG (a–c), graphone (d–f), N-graphene (g–i) and siliphene (j–l). | |
For a true quantitative interpretation of the MFM images, it is necessary to have precise knowledge of the geometry, magnetic properties of the tip and the tip-sample interactions in order to express the force acting on the tip, which has only been achieved in special cases.83,84 Nevertheless, a qualitative analysis can be carried out by considering the phase and amplitude changes observed in the MFM images (Fig. 7). It should be noted that an attractive interaction between the between the tip and the sample leads to a negative phase shift (dark contrast), while a repulsive interaction will lead to a positive phase shift (bright contrast). In fact, the MFM phase and amplitude images show a very good correlation in the formation of the magnetic domains. For all the FLG samples, the magnetic domains appear as dark and bright-localized regions in the phase and amplitude images respectively. Considering Fig. 7(c, f, i and l) representing amplitude images of pristine, graphone, N-graphene and siliphene, it can be clearly observed that the magnetic domains in the graphone/N-graphene are more localized than in the case of pristine FLG and indeed siliphene. Especially, in the case of siliphene, the lack of contrast in phase and amplitude images with respect to pristine FLGs showed a reduction in the presence of magnetic domains which essentially leads to the lowering of the magnetic response from the sample. A simple visual scaling of MFM phase data suggests that the siliphene has the weakest magnetization, whereas the N-graphene/graphone the strongest magnetization effect, which is consistent with the M–H magnetization results, described above.
Effect of functionalisation
The application of graphene is limited in spintronics devices due to its semi-metallic behaviour. Hence, opening of the zero bandgap of graphene to semiconducting region is highly necessary with the fabrication of p-type and n-type semiconductors. For any type of spintronics device application, the materials should have semiconducting and enhanced magnetic properties. Hence, it is necessary to choose a particular element to functionalize the graphene to induce semi-metallic behaviour to semiconducting behaviour without compromising beneficial magnetic properties so that the materials can withstand the applications as observed in diluted magnetic semiconductor (DMSs). It is well known that graphene possesses very profitable magnetic behaviour due to its sp2 hybridization bonding between carbons. The magnetic hysteresis of the samples show a form of ferromagnetic field response i.e. induced ferromagnetism behaviour both at low temperature and room temperature in all pristine and functionalized systems [Fig. 5(a–d)] wherein an increase in the saturation magnetization is observed; which is a natural consequence of quenching of thermal energy from the magnetic spin system upon lowering the temperature. However, the magnetic moment at low temperature is higher than the room temperature. This indicates the defective structure associated in the graphene is due to the disruption of the sp2 hybridization of the carbon atoms. It is noteworthy that graphene usually shows p-type conduction due to the presence of adsorbed oxygen or water molecules on the graphene surface. Hence, graphene functionalized with H, N and Si is taken in consideration to form n-type semiconductor and to observe how magnetic behavior changes with respect to the functionalized elements.
Hydrogen incorporation of graphene increases the sp3 hybridization but bonding with carbon reduces the defect present in the pristine graphene sheet. In this case, there is not much variation in the saturation magnetization value at low and room temperature as shown in Fig. 5(b). The saturation magnetization value is increased to four times the saturation value of pristine graphene. Even the magnetic behaviour is enhanced by the functionalization of H, but it is not considered suitable materials to use in field effect transistor, memory storage devices and other spintronics devices due to small opening of the bandgap (i.e. 0.27 eV).85 However, nitrogen doped/functionalized graphene sheet (N-graphene) shows significant enhancement of magnetism as observed and shown in Fig. 5(c). It was reported earlier by the authors and many other groups33 that nitrogen has a strong affinity towards carbon and forms sp2 like hybridized bonds. Not only does the sp2 hybridization bonding increases but as well it increases the percentage of unpaired electrons due to its electronic structure of 1s22s22p3. As nitrogen's p orbital is half filled, its bonding with carbon is always left with one unpaired electrons. This unpaired electron is the mechanism of enhancing the saturation magnetization in a large value i.e. 34 times higher than the saturation magnetization value of graphene. It is reported that the bandgap of N-functionalized graphene is almost 1.23 eV 85 and the nature of semiconductor is of n-type. However, the maximum opening of bandgap can occur up to 2.01 eV depending upon the percentage of Si in graphene86 and the structure is popularly known as siliphene. The M–H loop measurements for Si functionalized graphene are plotted in Fig. 5(d). The as measured data demonstrates magnification values that are extremely small, viz. more than one order of magnitude smaller than the other materials studied in this report, hence a diamagnetic signal was detected especially at higher fields in the form dM/dH < 0. It is observed that the saturation magnetisation was decreased by 30 times in comparison to the pristine graphene.
This reduction in the magnetic behaviour suggests that Si incorporation in graphene forms a strong interaction between Si–C which are tetrahedral bonded at the cost of sp2 hybridization present in graphene. However, during synthesis and subsequent exposure to the ambient environment, the contamination of oxygen occurs with Si and forms a bond Si–O and as a consequence, it reacts with carbon contain graphene and forms clusters like Si–O–C (defect structure). This is the mechanism behind the observation of ferromagnetic behaviour. Here, the saturation magnetization does not vary a lot at 40 K and 300 K, which represents that there is a structural ordering between Si and C. It is reported in literature that the incorporation of Si in graphene transforms the semi-metallic magnetic graphene to semiconducting magnetic graphene which has a large area of application field emission transistors and in spintronics devices. In this case, Si is a good candidate to provide both semiconducting as well as magnetic properties in graphene if Si can be doped in a controlled way. As in our study, Si incorporation reduces the magnetization value severely but on the other hand opens a new path for the application in spintronics devices.
To have a comparative view on saturation magnetization, remnant magnetization and coercivity of H, N and Si functionalized graphene, the analysis has been presented in Table 1. It seems that the coercivity values of siliphene are highest and the corresponding saturation magnetisation are lowest compared to graphone/N-graphene. Similar behaviour has been observed in graphene oxide and reduced graphene oxides and has been attributed to an increase in the structural disorder leading to an increase in the anisotropy energy density which leads to an increase in the coercivity.87 The siliphene is amenable to high coercivity ferromagnetic materials and are known as magnetically hard materials; whereas the N-graphene shows low coercivity and are termed to be magnetically soft materials. However, the coercivity values of graphone/N-graphene/siliphene are almost similar at 40 K and 300 K respectively, indicating that all the elements are homogenously distributed throughout the graphene matrix and have no thermal evolution of the magnetic species or the coupling between them. This homogeneous arrangement of functionalized elements in graphene represents the synthesis technique adopted is a unique one for the fabrication of junction devices and field effect transistors as desired for semiconductor applications.
Table 1 Magnetic properties of the pristine and plasma treated graphene samples at 40 K and 300 K
Sample/temperature |
Hc (coercivity) (Oe) |
Ms (saturation magnetisation) (emu g−1) |
Mr (remnant magnetisation) (emu g−1) |
Pristine FLG |
40 K |
112.37 |
3.47 × 10−4 |
0.52 × 10−4 |
300 K |
62.98 |
2.60 × 10−4 |
0.42 × 10−4 |
![[thin space (1/6-em)]](https://www.rsc.org/images/entities/char_2009.gif) |
Graphone |
40 K |
76.19 |
13.94 × 10−4 |
1.91 × 10−4 |
300 K |
52.88 |
12.91 × 10−4 |
1.28 × 10−4 |
![[thin space (1/6-em)]](https://www.rsc.org/images/entities/char_2009.gif) |
N-graphene |
40 K |
40.00 |
118.62 × 10−4 |
9.74 × 10−4 |
300 K |
25.42 |
111.91 × 10−4 |
6.04 × 10−4 |
![[thin space (1/6-em)]](https://www.rsc.org/images/entities/char_2009.gif) |
Siliphene |
40 K |
120.03 |
0.11 × 10−4 |
0.03 × 10−4 |
300 K |
94.75 |
0.09 × 10−4 |
0.02 × 10−4 |
Conclusions
In summary, we have observed the room-temperature ferromagnetism of different FLG materials functionalized with hydrogen/nitrogen/silicon species. The ferromagnetism may originate in the long-range coupling of spin units existing as defects in graphene sheets, which are generated during functionalization process. But more direct evidence is needed for a quantitative assessment. The intrinsic room-temperature ferromagnetic character of the materials, but without the constraint of spontaneous magnetization, combined with its semi-conductivity and functionalization capability, should have wide-reaching implications in material science, and these collective properties could make graphene-based materials a competent choice for many important device applications, including spintronics, magneto-resistance, magnetic memory devices, and so on. Our results may also prompt more thorough and quantitative assessment of room-temperature ferromagnetism of pure FLG, including graphone/N-graphene/siliphene. Moreover, we believe that the non-uniform distribution of the hydrogen, nitrogen and silicon atoms over the FLG plane may also produce ferromagnetic clustering or spin-glass dynamics with attributes that would also deserve further in-depth studies beyond the present state of magnetization.
Acknowledgements
The author S.C.R. acknowledges to National Research Foundation, South Africa for financial support. AMS thanks the SA-NRF (93549), the FRC and URC of UJ for financial assistance. D. Britz is thanked for his assistance with the magnetic measurements.
Notes and references
- K. S. Novoselov, A. K. Geim, S. V. Morozov, D. Jiang, Y. Zhang, S. A. Dubonos, I. V. Grigorieva and A. A. Firsov, Science, 2004, 306, 666–669 CrossRef CAS PubMed.
- A. K. Geim and K. S. Novoselov, Nat. Mater., 2007, 6, 183–191 CrossRef CAS PubMed.
- R. K. Layek and A. K. Nandi, Polymer, 2013, 54, 5087–5103 CrossRef CAS.
- E. W. Hill, A. Vijayaragahvan and K. S. Novoselov, IEEE Sens. J., 2011, 11(12), 3161–3170 CrossRef CAS.
- W. Choi, I. Lahiri, R. Seelaboyina and Y. S. Kang, Crit. Rev. Solid State Mater. Sci., 2010, 35, 52–71 CrossRef CAS.
- Y. Si and E. T. Samulski, Nano Lett., 2008, 8(6), 1679–1682 CrossRef CAS PubMed.
- V. Singh, D. Joung, L. Zhai, S. Das, S. I. Khondaker and S. Seal, Prog. Mater. Sci., 2011, 56(8), 1178–1271 CrossRef CAS.
- N. A. H. Castro, F. Guinea, N. M. R. Peres, K. S. Novoselov and A. K. Geim, Rev. Mod. Phys., 2009, 81, 109 CrossRef.
- S. Basu and P. Bhattacharyya, Sens. Actuators, B, 2012, 173, 1–21 CrossRef CAS.
- D. A. L. Gomez, Y. Zhang, C. W. Schlenker, K. Ryu, M. E. Thompson and C. Zhou, ACS Nano, 2010, 4(5), 2865–2873 CrossRef PubMed.
- Y. Zhu, S. Murali, W. Cai, X. Li, J. W. Suk, J. R. Potts and R. S. Ruoff, Adv. Mater., 2010, 22(35), 3906–3924 CrossRef CAS PubMed.
- M. J. Allen, V. C. Tung, L. Gomez, Z. Xu, L. M. Chen, K. S. Nelson, C. Zhou, R. B. Kaner and Y. Yang, Adv. Mater., 2009, 21, 2098–2102 CrossRef CAS.
- R. V. Gorbachev, A. S. Mayorov, A. K. Savchenko, D. W. Horsell and F. Guinea, Nano Lett., 2008, 8(7), 1995–1999 CrossRef CAS PubMed.
- M. Dragoman and D. Dragoman, Prog. Quantum Electron., 2009, 33(6), 165–214 CrossRef CAS.
- Y. Zhao, X. G. Li, X. Zhou and Y. N. Zhang, Sens. Actuators, B, 2016, 231, 324–340 CrossRef CAS.
- R. K. Upadhyay, N. Soin and S. S. Roy, RSC Adv., 2014, 4(8), 3823–3851 RSC.
- F. Schwierz, Nat. Nanotechnol., 2010, 5, 487–496 CrossRef CAS PubMed.
- D. W. Boukhvalov, M. I. Katsnelson and A. I. Lichtenstein, Phys. Rev. B: Condens. Matter Mater. Phys., 2008, 77, 035427 CrossRef.
- G. Mittal, V. Dhand, K. Y. Rhee, S. J. Park and W. R. Lee, J. Ind. Eng. Chem., 2015, 21, 11–25 CrossRef CAS.
- L. Li, R. Qin, H. Li, L. Yu, Q. Liu, G. Luo, Z. Gao and J. Lu, ACS Nano, 2011, 5(4), 2601–2610 CrossRef CAS PubMed.
- J. Zhou, Q. Wang, Q. Sun, X. S. Chen, Y. Kawazoe and P. Jena, Nano Lett., 2009, 9(11), 3867–3870 CrossRef CAS PubMed.
- N. Lu, Z. Li and J. Yang, J. Phys. Chem. C, 2009, 113(38), 16741–16746 CAS.
- S. C. Ray, N. Soin, T. Magkato, C. H. Chuang, W. F. Pong, S. S. Roy, S. K. Ghosh, A. M. Strydom and J. A. McLaughlin, Sci. Rep., 2014, 4, 3862 Search PubMed.
- D. C. Elias, R. R. Nair, T. M. G. Mohiuddin, S. V. Morozov, P. Blake, M. P. Halsall, A. C. Ferrari, D. W. Boukhvalov, M. I. Katsnelson, A. K. Geim and K. S. Novoselov, Science, 2009, 323, 610–613 CrossRef CAS PubMed.
- X. D. Wen, T. Yang, R. Hoffman, N. W. Ashcroft, R. L Martin, S. P. Rudin and J. X. Zhu, ACS Nano, 2012, 6(8), 7142–7150 CrossRef CAS PubMed.
- J. D. Jones, K. K. Mahajan, W. H. Williams, P. A. Ecton, Y. Mo and J. M. Perez, Carbon, 2010, 48, 2335–2340 CrossRef CAS.
- S. U. Lee, R. V. Belosludov, H. Mizuseki and Y. Kawazoe, Small, 2009, 5(15), 1769–1775 CrossRef CAS PubMed.
- Y. F. Li, Z. Zhou, P. W. Shen and Z. F. Chen, ACS Nano, 2009, 3(7), 1952–1958 CrossRef CAS PubMed.
- Y. C. Ma, A. S. Foster, A. V. Krasheninnikov and R. M. Nieminen, Phys. Rev. B: Condens. Matter Mater. Phys., 2005, 72, 205416 CrossRef.
- C. C. Ma, X. H. Shao and D. P. Cao, J. Mater. Chem., 2012, 22, 8911–8915 RSC.
- Y. Zhang, S. Talapatra, S. Kar, R. Vajtai, S. K. Nayak and P. M. Ajayan, Phys. Rev. Lett., 2007, 99, 107201 CrossRef CAS PubMed.
- J. Y. Dai and J. M. Yuan, Phys. Rev. B: Condens. Matter Mater. Phys., 2010, 81, 165414 CrossRef.
- Y. Liu, N. Tang, X. Wan, Q. Feng, M. Li, Q. Xu, F. Liu and Y. Du, Sci. Rep., 2013, 3, 2566 Search PubMed.
- S. Jalili and R. Vaziri, Mol. Phys., 2011, 109, 687–694 CrossRef CAS.
- C. W. Pao, S. C. Ray, H. M. Tsai, Y. S. Chen, H. C. Chen, I. N. Lin, W. F. Pong, J. W. Chiou, N. G. Shang and P. Papakonstantinou, J. Phys. Chem. C, 2010, 114(18), 8161–8166 CAS.
- P. A. Denis, Chem. Phys. Lett., 2010, 492, 251–257 CrossRef CAS.
- K. Ando, Science, 2006, 312, 1883–1885 CrossRef CAS PubMed.
- Y. F. Wang, S. B. Singh, M. V. Limaye, Y. C. Shao, S. H. Hsieh, L. Y. Chen, H. C. Hsueh, H. T. Wang, J. W. Chiou, Y. C. Yeh, C. W. Chen, C. H. Chen, S. C. Ray, J. Wang, W. F. Pong, Y. Takagi, T. Ohigashi, T. Yokoyama and N. Kosugi, Sci. Rep., 2015, 5, 15439 CrossRef CAS PubMed.
- S. B. Fagan, R. Mota, R. J. Baierle, A. J. R. daSilva and A. Fazzio, Diamond Relat. Mater., 2003, 12, 861–863 CrossRef CAS.
- J. W. Chiou, S. C. Ray, S. I. Peng, C. H. Chuang, B. Y. Wang, H. M. Tsai, C. W. Pao, H. J. Lin, Y. C. Shao, Y. F. Wang and S. C. Chen, J. Phys. Chem. C, 2012, 116(30), 16251–16258 CAS.
- S. C. Ray, U. Palnitkar, C. W. Pao, H. M. Tsai, W. F. Pong, I. N. Lin, P. Papakonstantinou, A. Ganguly, L. C. Chen and K. H. Chen, J. Appl. Phys., 2008, 104, 063710 CrossRef.
- S. C. Ray, W. Mbiombi and P. Papakonstantinou, Curr. Appl. Phys., 2014, 14, 1845–1848 CrossRef.
- A. C. Ferrari, J. C. Meyer, V. Scardaci, C. Casiraghi, M. Lazzeri, F. Mauri, S. Piscanec, D. Jiang, K. S. Novoselov, S. Roth and A. K. Geim, Phys. Rev. Lett., 2006, 97, 187401 CrossRef CAS PubMed.
- A. C. Ferrari, Solid State Commun., 2007, 143, 47–57 CrossRef CAS.
- F. Tuinstra and J. L. Koenig, J. Chem. Phys., 1970, 53, 1126 CrossRef CAS.
- A. C. Ferrari and J. Robertson, Phys. Rev. B: Condens. Matter Mater. Phys., 2000, 61, 14095 CrossRef CAS.
- W. Wu, Q. Yu, P. Peng, Z. Liu, J. Bao and S. S. Pei, Nanotechnology, 2012, 23, 035603 CrossRef PubMed.
- N. Soin, S. S. Roy, S. Roy, K. S. Hazra, D. S. Misra, T. H. Lim, C. J. D. Hetherington and J. A. McLaughlin, J. Phys. Chem. C, 2011, 115, 5366–5372 CAS.
- N. Soin, S. S. Roy, C. OKane, J. A. D. McLaughlin, T. H. Lim and C. J. D. Hetherington, CrystEngComm, 2011, 13, 312–318 RSC.
- K. S. Subrahmanyam, L. S. Panchakarla, A. Govindaraj and C. N. R. Rao, J. Phys. Chem. C, 2009, 113(11), 4257–4259 CAS.
- S. Pisana, M. Lazzeri, C. Casiraghi, K. S. Novoselov, A. K. Geim, A. C. Ferrari and F. Mauri, Nat. Mater., 2007, 6(3), 198–201 CrossRef CAS PubMed.
- J. Yan, Y. Zhang, P. Kim and A. Pinczuk, Phys. Rev. Lett., 2007, 98(16), 166802 CrossRef PubMed.
- Y. Y. Wang, Z. H. Ni, T. Yu, Z. X. Shen, H. M. Wang, Y. H. Wu, W. Chen and A. T. S. Wee, J. Phys. Chem. C, 2008, 112(29), 10637–10640 CAS.
- D. Graf, F. Molitor, K. Ensslin, C. Stampfer, A. Jungen, C. Hierold and L. Wirtz, Nano Lett., 2007, 7(2), 238–242 CrossRef CAS PubMed.
- S. Ryu, M. Y. Han, J. Maultzsch, T. F. Heinz, P. Kim, M. L. Steigerwald and L. E. S. Brus, Nano Lett., 2008, 8(12), 4597–4602 CrossRef CAS PubMed.
- L. Xie, L. Jiao and H. Dai, J. Am. Chem. Soc., 2010, 132(42), 14751–14753 CrossRef CAS PubMed.
- A. Eckmann, A. Felten, A. Mishchenko, L. Britnell, R. Krupke, K. S. Novoselov and C. Casiraghi, Nano Lett., 2012, 12(8), 3925–3930 CrossRef CAS PubMed.
- S. C. Ray, J. W. Chiou, W. F. Pong and M. H. Tsai, Crit. Rev. Solid State Mater. Sci., 2006, 31(4), 91–110 CrossRef CAS.
- S. C. Ray, C. W. Bao, H. M. Tsai, J. W. Chiou, J. C. Jan, K. K. Kumar, W. F. Pong, M. H. Tsai, W. J. Wang, C. J. Hsu and T. I. T. Okpalugo, Appl. Phys. Lett., 2004, 85, 4022–4024 CrossRef CAS.
- V. A. Terekhov, E. I. Terukov, I. N. Trapeznikova, M. Kashkarov, O. V. Kurilo, S. Y. Turishchev, A. B. Golodenko and E. P. Domashevskaya, Semiconductors, 2005, 39(7), 830–834 CrossRef CAS.
- Y. K. Chang, H. H. Hsieh, W. F. Pong, M. H. Tsai, K. H. Lee, T. E. Dann, F. Z. Chien, P. K. Tseng, K. L. Tsang, W. K. Su and L. C. Chen, Phys. Rev. B: Condens. Matter Mater. Phys., 1998, 58, 9018 CrossRef CAS.
- M. Pedio, F. Borgatti, A. Giglia, N. Mahne, S. Nannarone, S. Giovannini, C. Cepek, E. Magnano, G. Bertoni, E. Spiller and M. Sancrotti, Phys. Scr., 2005, 115, 695–698 CrossRef.
- D. Geng, S. Yang, Y. Zhang, J. Yang, J. Liu, R. Li, T. K. Sham, X. Sun, S. Ye and S. Knights, Appl. Surf. Sci., 2011, 257(21), 9193–9198 CrossRef CAS.
- S. C. Ray, C. W. Pao, J. W. Chiou, H. M. Tsai, J. C. Jan, W. F. Pong, R. McCann, S. S. Roy, P. Papakonstantinou and J. A. McLaughlin, J. Appl. Phys., 2005, 98, 033708 CrossRef.
- R. Gago, I. Jiménez, J. Neidhardt, B. Abendroth, I. Caretti, L. Hultman and W. Möller, Phys. Rev. B: Condens. Matter Mater. Phys., 2005, 71, 125414 CrossRef.
- H. Dong, C. Liu, H. Ye, L. Hu, B. Fugetsu, W. Dai, Y. Cao, X. Qi, H. Lu and X. Zhang, Sci. Rep., 2015, 5, 17542 CrossRef CAS PubMed.
- M. W. Gaultois and A. P. Grosvenor, J. Mater. Chem., 2011, 21, 1829–1836 RSC.
- D. Li, G. M. Bancroft, M. Kasrai, M. E. Fleet, X. H. Feng, K. H. Tan and B. X. Yang, Solid State Commun., 1993, 87, 613 CrossRef CAS.
- H. M. Tsai, S. C. Ray, C. W. Pao, J. W. Chiou, C. L. Huang, C. H. Du, W. F. Pong, M. H. Tsai, A. Fukano and H. Oyanagi, J. Appl. Phys., 2008, 103, 013704 CrossRef.
- A. Avila, I. Montero, L. Galan, J. M. Ripalda and R. Levy, J. Appl. Phys., 2001, 89(1), 212–216 CrossRef CAS.
- H. Kim, M. Seo, M. H. Park and J. Cho, Angew. Chem., Int. Ed., 2010, 49(12), 2146–2149 CrossRef CAS PubMed.
- H. González-Herrero, J. M. Gómez-Rodríguez, P. Mallet, M. Moaied, J. J. Palacios, C. Salgado, M. M. Ugeda, J. Y. Veuillen, F. Yndurain and I. Brihuega, Science, 2016, 352(6284), 437–441 CrossRef PubMed.
- H. R. Matte, K. S. Subrahmanyam and C. N. R. Rao, J. Phys. Chem.
C, 2009, 113(23), 9982–9985 CAS.
- H. X. Yang, M. Chshiev, D. W. Boukhvalov, X. Waintal and S. Roche, Phys. Rev. B: Condens. Matter Mater. Phys., 2011, 84, 214404 CrossRef.
- C. S. Rout, A. Kumar, N. Kumar, A. Sundaresan and T. S. Fisher, Nanoscale, 2011, 3(3), 900–903 RSC.
- Z. He, X. Yang, H. Xia, X. Zhou, M. Zhao, Y. Song and T. Wang, Carbon, 2011, 49(6), 1931–1938 CrossRef CAS.
- J. S. Bunch, S. S. Verbridge, J. S. Alden, A. M. Van Der Zande, J. M. Parpia, H. G. Craighead and P. L. McEuen, Nano Lett., 2008, 8(8), 2458–2462 CrossRef CAS PubMed.
- G. Ning, C. Xu, L. Hao, O. Kazakova, Z. Fan, H. Wang, K. Wang, J. Gao, W. Qian and F. Wei, Carbon, 2013, 51, 390–396 CrossRef CAS.
- Q. Miao, L. Wang, Z. Liu, B. Wei, F. Xu and W. Fei, Sci. Rep., 2016, 6, 21832 CrossRef CAS PubMed.
- O. V. Yazyev and L. Helm, Phys. Rev. B: Condens. Matter Mater. Phys., 2007, 75, 25408 Search PubMed.
- Y. Wang, Y. Huang, Y. Song, X. Zhang, Y. Ma, J. Liang and Y. Chen, Nano Lett., 2009, 9, 220–224 CrossRef CAS PubMed.
- L. Xie, X. Wang, J. Lu, Z. Ni, Z. Luo, H. Mao, R. Wang, Y. Wang, H. Huang, D. Qi and R. Liu, Appl. Phys. Lett., 2011, 98, 193113 CrossRef.
- J. Červenka, M. I. Katsnelson and C. F. J. Flipse, Nat. Phys., 2009, 5(11), 840–844 CrossRef.
- W. K. Lee, K. E. Whitener, J. T. Robinson and P. E. Sheehan, Adv. Mater., 2015, 27(10), 1774–1778 CrossRef CAS PubMed.
- R. Lv and M. Terrones, Mater. Lett., 2012, 78, 209–218 CrossRef CAS.
- M. S. S. Azadeh, A. Kokabi, M. Hosseini and M. Fardmanesh, Micro Nano Lett., 2011, 6, 582–585 CAS.
- K. Bagani, A. Bhattacharya, J. Kaur, A. R. Chowdhury, B. Ghosh, M. Sardar and S. Banerjee, J. Appl. Phys., 2014, 115, 023902 CrossRef.
- N. Soin, S. S. Roy, S. K. Mitra, T. Thundat and J. A. McLaughlin, J. Mater. Chem., 2012, 22, 14944–14950 RSC.
- N. Soin, S. S. Roy, S. Sharma, T. Thundat and J. A. McLaughlin, J. Solid State Electrochem., 2013, 17, 2139–2149 CrossRef CAS.
- N. G. Shang, P. Papakonstantinou, M. McMullan, M. Chu, A. Stamboulis, A. Potenza, S. S. Dhesi and H. Marchetto, Adv. Funct. Mater., 2008, 18, 3506–3514 CrossRef CAS.
Footnote |
† Electronic supplementary information (ESI) available. See DOI: 10.1039/c6ra14457h |
|
This journal is © The Royal Society of Chemistry 2016 |