DOI:
10.1039/C6RA14010F
(Paper)
RSC Adv., 2016,
6, 78322-78334
Electronic structure and optical property of metal-doped Ga2O3: a first principles study
Received
30th May 2016
, Accepted 29th July 2016
First published on 8th August 2016
Abstract
The difficulty in fabricating p-type Ga2O3 is a crucial issue which restricts its applications in practical devices. In the present work, we have performed first principles studies on formation energies, electronic structures and optical properties for a series of metal doped β-Ga2O3, involving a long list of main group and transition metals, even some lanthanides, to search for potential p-type dopants. Calculations have shown that Li and Be, both with small atomic radius, prefer interstitial doping rather than substitutional doping of Ga, resulting eventually in an n-type character to the doped system. In addition, an O-rich atmosphere is more favorable for p-type substitutional dopings by comparison with the Ga-rich condition. A number of metal dopants show potential in achieving p-type β-Ga2O3, for example, Na, Mg, Ca, Cu, Ag, Zn, Cd, which are all worth a further emphasis study in experiment, although a satisfying holes concentration may only be possible to achieve by simultaneously committing to both the elimination of n-type background carriers and the activation mechanism of dopants. Absorption spectra have shown that all the above-mentioned potential p-type dopants are suitable for deep UV applications. The major peaks of the absorption spectra are red-shifted in most cases, due to the introduction of new states to the forbidden gap by dopants, which have been discussed in detail by inspecting into the partial density of states. Ga2O3 doped by some transition metals show potential as magnetic devices.
1 Introduction
Gallium oxide (Ga2O3) is the representative fourth-generation wide-bandgap semiconductor, which consists of five different crystalline structures, defined as α, β, γ, δ, ε-phases.1 Both theoretical studies2,3 and experiment measurements4,5 have shown that the monoclinic β-Ga2O3 is the most stable phase thermodynamically with a band gap of 4.9 eV.6,7 β-Ga2O3 has attracted significant attention due to its unique electric properties and good performance in high brightness LEDs, deep ultraviolet optoelectronics, ultra-high voltage power devices, and so on.8–12 The absorption edge of β-Ga2O3 is around 250 nm, making Ga2O3 itself already meet the needs of deep UV devices by avoiding the alloying strategies. In addition, β-Ga2O3-based power devices have majority advantages including high breakdown voltage, low loss, and high power capacity compared with those based on silicon technologies.11 Moreover, large-size, high-quality and low-cost single Ga2O3 crystals can be grown by traditional techniques, which are more effective and mature than many other wide-bandgap semiconductors.13–15
However, there are still “bottleneck problems” need to be addressed in the application of this material. It is hard to improve the quality of the bulk β-Ga2O3 owing to the existence of the cleavage plane. Moreover, the growth of high quality p-type β-Ga2O3 with high carrier concentration is difficult, due to the high self-compensation effect by n-type background carriers, and the low solubility and activation rate of dopants.16–18 Up so far, there are a few reports on successful p-type doping on β-Ga2O3 nanowire,19,20 however, to the best of our knowledge, there is still no public reports of p-type doping on both bulk and film structures up so far, which largely restricts the manufacture of the p–n junction semiconductor devices. In purpose of obtaining high quality p-type β-Ga2O3, researchers attempted a wide range of dopants, including metal21–35 or non-metal elements.36–38 Guo et al. have performed systematic first principles studies on electronic structures and optical properties of non-metal doped β-Ga2O3.39 Theoretical studies have also examined some metal dopants, such as Cr, Mn, Zn, Cu, Ni, W.40–45 However, continuous efforts need to be made in searching for more efficient p-type dopants, as well as in eliminating self-compensation effect and improving the activation rate for dopants.
Based on above-mentioned issues, we will perform successive and systematic studies on effective p-type doping strategies of β-Ga2O3. In present paper, we put our emphasis on searching of potential p-type dopants, by performing a thorough analysis on metal dopants, involving a long list of main group and transition metals in the periodic table, even some lanthanides. We consider not only the substitutional doping, but also the interstitial doping for some atoms with smaller radius than Ga. Moreover, for some potential elements, we further display the absorption spectra, which may provide useful information for applications, especially in ultraviolet optoelectronic devices.
Models and computational details will be present in Section 2. In Section 3, results and discussion will be shown. Conclusive remarks will be collected in Section 4.
2 Computational details
All calculations are based on density functional theory (DFT) with Perdew–Burke–Ernzerhof (PBE) of the generalized gradient approximation (GGA)46 functional for the exchange correlation function, implemented in Vienna Ab Initio Simulation Package (VASP).47,48 We employ the projector augmented-wave (PAW)49 method to describe the interaction of the ions and electrons. The kinetic energy cut-off is set to 340 eV. A 1 × 2 × 1 supercell with 40 atoms is used after checking convergence. We choose a Monkhorst–Pack set of 4 × 6 × 4 for the full optimization of the cell parameters for the bulk and 8 × 12 × 8 for other property calculations. The calculated lattice parameters of the supercell are a = 11.981 Å, b = 6.042 Å, c = 5.778 Å, β = 103.850 °C, which are in good agreement with the experimental data, with errors less than 2.08%.50 Geometries are considered to be converged while the force on each atom is no more than 0.01 eV Å−1.
GGA is well-known for its underestimation on band gaps, however, it is computationally cheap and well-preferred in especially studies involving large number of calculations. In present work, we will examine a long list of dopants, which makes GGA a good choice for series of calculations required. In order to prove the reliability of the results obtained by GGA functional, we have also calculated the formation energies and the electronic structures of some p-type doped cases (Mg, Cu, and Zn) with both GGA+U formalism51,52 and Heyd–Scuseria–Ernzerhof (HSE) hybrid functional,53,54 which will be collected and discussed in Sec. 3.5. In accordance with the computational procedure in ref. 55, we have determined the optimized parameter for our computation after series of test calculations. The Coulomb and exchange parameters of GGA+U method are set to U = 9 eV, J = 1 eV. For the HSE calculation, the screening parameter is set to 0.2 Å−1 and the amount of exact exchange is set to 0.37. The other computational parameters are similar to those used in GGA method. Band gaps obtained with these parameters are in good agreement with the experimental value.
3 Results and discussion
3.1 Bulk undoped β-Ga2O3
The optimized model of bulk Ga2O3 is displayed in Fig. 1(a), with a 1 × 2 × 1 supercell containing 16 gallium (Ga) atoms and 24 oxygen (O) atoms. There are two nonequivalent Ga sites and three nonequivalent O sites among these atoms. Only the two different sites of Ga need to be considered in our calculations on metal dopants. As we can see from the model, Ga1 is located in a tetrahedron formed by four O atoms, while Ga2 forms an octahedron with six O anions. The bond lengths of Ga1–O1, Ga2–O1, Ga1–O2, Ga2–O2, Ga1–O3, Ga2–O3 are respectively 1.848 Å, 1.915 Å, 1.842 Å, 1.952 Å, 1.863 Å, 2.006 Å, which are in good agreement with both experiment and previous calculations.39,50
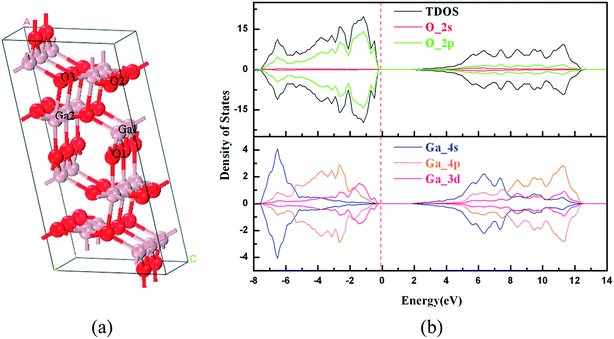 |
| Fig. 1 (a) Optimized structure of a 1 × 2 × 1 supercell of bulk undoped β-Ga2O3. Red spheres represent O atoms and pink spheres represent Ga atoms. (b) The total and partial density of states of bulk undoped β-Ga2O3. The red vertical dashed line is the Fermi level which has been set to zero. | |
Fig. 1(b) gives the information on the total density of states (TDOS) and partial density of states (PDOS) of bulk undoped Ga2O3. As we can see from the TDOS, the band gap predicted by GGA is 1.87 eV, which is much smaller than the experimental value (4.9 eV), due to the well-known intrinsic error of GGA in underestimating the band gap. However, since the major purpose of present work is mainly focusing on searching for potential p-type metal dopants, therefore, a precise prediction on band gap is not mandatory. In Sec. 3.5 we have given a detailed comparison on different computational methods, and the reliability of GGA results has been well proved by both GGA+U and HSE predictions. Therefore, a corrective error of 3.03 eV will be artificially added directly in following discussions. After an analysis of PDOS, we can see that the valence band maximum (VBM) is mainly contributed by O 2p states together with a little contribution of Ga 4p and 3d states, whereas the conduction band minimum (CBM) is contributed by Ga 4s states. The extensive overlap among Ga 3d, Ga 4p and O 2p states indicates the strong coupling between Ga and O atoms.
3.2 Formation energies
The formation energy (Ef) in charge state q = 0 is defined as following: |
Ef = Edoped − Eundoped + μGa − μM
| (1) |
where Edoped is the total energy of the metal doped β-Ga2O3 and Eundoped represents the energy of the bulk without doping. μGa and μM indicate the chemical potential for Ga and dopant metal, respectively. Due to the influence of the natural conditions during synthesizing the Ga2O3 material, the chemical potential for Ga is divided into two contrary cases. The chemical potential of Ga and O in β-Ga2O3 must satisfy the relationship:where, the quantity μbulk is the chemical potential of the bulk Ga2O3. Under Ga-rich condition, μGa is directly calculated by the Ga atoms in the bulk crystal. Under O-rich condition, μGa is determined by eqn (2), where the chemical potential of oxygen μO is obtained from the energy of oxygen in a cubic crystal with parameter of 10 Å. The chemical potential of dopants μM is calculated from the energy of the most stable bulk crystal of the doped metal atoms. Two nonequivalent Ga sites will be considered in our calculations. We summarize formation energies in Table 1, which are determined to examine the stabilities of the metal doped systems. The symbol X_1 and X_2 in the table represent the substitutional doping of metal element X on Ga1 and Ga2 sites, respectively.
Table 1 Formation energies of metal substitutional doped β-Ga2O3 under Ga-rich and O-rich conditions, respectively
Ef (eV) |
Ga-rich |
O-rich |
Ef (eV) |
Ga-rich |
O-rich |
Li_1 |
4.38 |
−0.70 |
Na_1 |
6.07 |
1.00 |
Li_2 |
4.54 |
−0.53 |
Na_2 |
5.68 |
0.60 |
K_1 |
7.60 |
2.53 |
Be_1 |
−0.27 |
−5.34 |
K_2 |
6.72 |
1.65 |
Be_2 |
0.77 |
−4.30 |
Mg_1 |
1.02 |
−4.05 |
Ca_1 |
2.24 |
−2.84 |
Mg_2 |
0.70 |
−4.37 |
Ca_2 |
1.63 |
−3.45 |
In_1 |
2.32 |
−2.75 |
Tl_1 |
5.75 |
0.68 |
In_2 |
1.73 |
−3.35 |
Tl_2 |
5.50 |
0.43 |
Pb_1 |
5.33 |
0.26 |
Sn_1 |
3.47 |
−1.60 |
Pb_2 |
5.22 |
0.15 |
Sn_2 |
3.00 |
−2.07 |
Ti_1 |
−1.05 |
−6.12 |
Pd_1 |
6.94 |
1.87 |
Ti_2 |
−1.41 |
−6.48 |
Pd_2 |
5.38 |
0.31 |
Mn_1 |
0.42 |
−4.65 |
Co_1 |
3.34 |
−1.73 |
Mn_2 |
0.06 |
−5.02 |
Co_2 |
2.87 |
−2.20 |
Ni_1 |
4.07 |
−1.00 |
Au_1 |
8.15 |
3.08 |
Ni_2 |
3.74 |
−1.33 |
Au_2 |
7.48 |
2.41 |
Cu_1 |
5.11 |
0.04 |
Ag_1 |
7.75 |
2.68 |
Cu_2 |
4.68 |
−0.39 |
Ag_2 |
6.86 |
1.78 |
Hg_1 |
7.26 |
2.19 |
Zn_1 |
3.35 |
−1.71 |
Hg_2 |
7.18 |
2.11 |
Zn_2 |
3.43 |
−1.66 |
Cd_1 |
5.53 |
0.46 |
Dy_1 |
−1.42 |
−6.50 |
Cd_2 |
5.26 |
0.19 |
Dy_2 |
−2.09 |
−7.16 |
Nd_1 |
−0.29 |
−5.36 |
Eu_1 |
1.29 |
−3.79 |
Nd_2 |
−0.59 |
−5.66 |
Eu_2 |
0.81 |
−4.27 |
Gd_1 |
−1.19 |
−6.27 |
Tb_1 |
−1.28 |
−6.35 |
Gd_2 |
−1.67 |
−6.74 |
Tb_2 |
−1.90 |
−6.97 |
It is worth to mention that GGA-predicted formation energies of the metal doped crystals are slightly lower than those by applying GGA+U and HSE methods, due to the correlation error in the d orbital and especially f orbital.56,57 However, it will have little effect on our comparison studies on different dopants, sites, and conditions, see details in Sec. 3.5. By inspecting into these Ef values of metal doped β-Ga2O3 in Table 1, we can find out the preferred doping sites, conditions and elements. First of all, for all considered metal dopants, the doping is favored under O-rich condition by comparison with Ga-rich condition. Under O-rich condition, the Ga atoms are easy to move away from the bulk crystal and the dopant has more opportunities to combine with the O atoms. Second, except the Li and Be atoms, most metal atoms prefer to substitute Ga2 atoms. It is mainly because that the atomic radii of Li and Be are much shorter than Ga and the octahedron sites of Ga2 have more space than the tetrahedron sites of Ga1. Comparing with substituting Ga2, Ga1-substitution produces more distortion to the crystal structure. It is also worth to mention that for Zn-doping the formation energies of two sites are similar, due to similar radius of Zn with Ga. Third, for elements in the same group, the impurity doping becomes more and more difficult with the radius increasing, such as Li < Na < K, Be < Mg < Ca, Cu < Ag < Au, Zn < Cd < Hg, In < Tl, Sn < Pb. Moreover, the calculated formation energies of the lanthanide doped crystals are lower than those doped by other transition metals.
3.3 Optimized structures and electronic properties
In this section, information on the optimized doped structures, total and partial density of states and charge density differences are shown in several charts. In order to give a more ordered and clearer discussion, we have divided the doped metal atoms into group IA and IIA, transition metals and group IIIA and IVA.
3.3.1 Group IA and IIA. This group contains alkalis and alkalis-earth metals. Since the formation energy of K is much higher than others in this group, it predicts that the K doped β-Ga2O3 is unstable. According to the tendency of metal doped cases, the system with Rb doping will have even larger formation energy than that with K doping, it is reasonable to ignore higher elements. Moreover, interstitial doping cases which are possible happen by some atoms with small radius, such as Li, Na, Be, Mg, are also considered in our calculations.For interstitial doping, three most probably doping sites have been chosen (Fig. 2(a)) in the 1 × 2 × 1 supercell of β-Ga2O3. The fractional coordinates of site1, site2 and site3 are (0.75, 0.25, 0), (0.5, 0.25, 0.5) and (0.5, 0.25, 1), respectively. In the following discussion, these sites are renamed respectively X1, X2, X3 after doping, where X represents the doping metal. In this case, the equation to calculate the formation energy in charge state q = 0 will change as following:
|
Ef = Edoped − Eundoped − μM
| (3) |
with the same definition of each quantity as mentioned before. Formation energies of different dopants on different sites are listed in
Table 2. Calculations show that Li and Be atoms are very probably to form interstitial-doping crystals, due to their small atomic radii, therefore, these two interstitial-dopings will be considered for further calculations. By comparing the formation energies of three different interstitial sites, we can find that the most unstable location is site3, which exists a strong interaction with the nearby Ga atoms. Interstitial site1 and site2 show rather small difference for the interstitial doing of Li, while Be is much more preferred site 1 instead. These results provide useful information for β-Ga
2O
3 interstitial-doping researches.
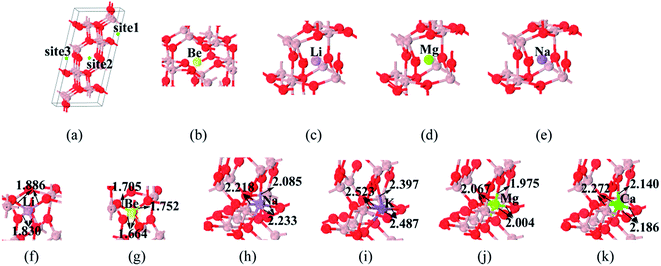 |
| Fig. 2 (a) The interstitial sites in the 1 × 2 × 1 supercell bulk β-Ga2O3. Optimized local structures for interstitial doped Ga2O3 by (b) Be, (c) Li, (d) Mg and (e) Na atoms. Optimized local structures of substitutional doped Ga2O3 by (f) Li, (g) Be, (h) Na, (i) K, (j) Mg and (k) Ca atoms. Bond lengths between several atoms are labeled in the figure. Red spheres represent O atoms and pink spheres represent Ga atoms. | |
Table 2 Formation energies of metal (Li, Be, Na, Mg) interstitial doped β-Ga2O3
Interstitial sites |
Ef (eV) |
Interstitial sites |
Ef (eV) |
Li_1 |
0.98 |
Be_1 |
1.53 |
Li_2 |
0.97 |
Be_2 |
2.64 |
Li_3 |
1.17 |
Be_3 |
2.67 |
Na_1 |
3.26 |
Mg_1 |
3.53 |
Na_2 |
3.14 |
Mg_2 |
3.47 |
Na_3 |
3.50 |
Mg_3 |
3.90 |
For interstitial-doping cases, the optimized structures are collected in Fig. 2(b)–(e), and optimized geometrical structures of substitutional doping cases are displayed in Fig. 2(f)–(k). Corresponding total and partial density of states are shown in Fig. 3. Average lengths between the doping metal atoms and the nearest O are 1.621 Å for Be–O, 2.012 Å for Li–O, 2.007 Å for Mg–O and 2.093 Å for Na–O, respectively. The optimized position for Be is site1 which is much closer to O atoms. Average lengths of Li–O and Mg–O are very close, which may attribute to similar ratios between their charge and atomic radius. From Fig. 3(a) and (b), we can see that the band gaps after interstitial doping are similar to the undoped crystal, but the positions of Fermi level increases significantly. The VBM after Li and Be interstitial doping show little changes with respect to the undoped crystal, whereas the CBM is consist of not only Ga 4s states but also the Li and Be 2s states, respectively. The interstitial atoms will donate their free electrons to the crystal, representing n-type characteristics.
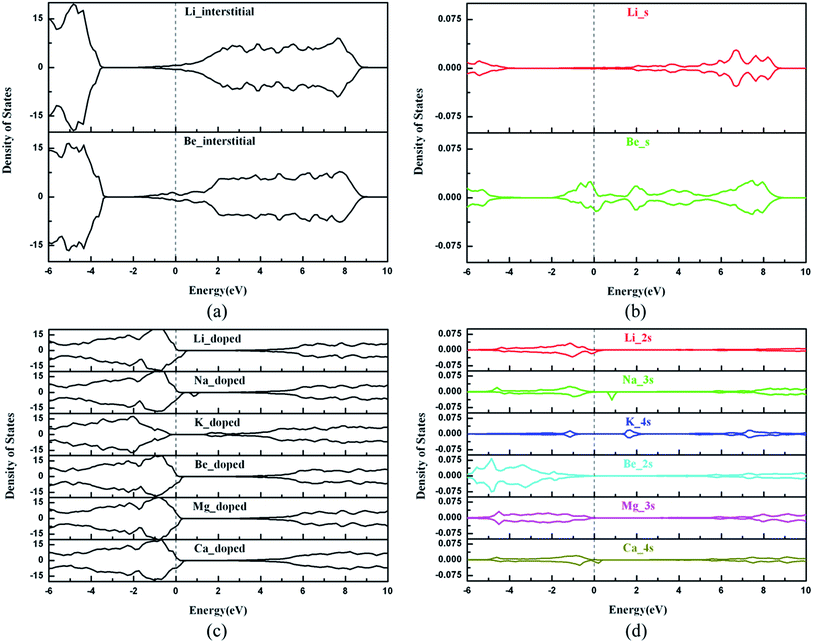 |
| Fig. 3 Total and partial density of states: (a) and (b) for interstitial doping of Li and Be; (c) and (d) for substitutional doping of Li, Na, K, Be, Mg and Ca. The vertical dashed line represents the Fermi level which has been set to zero. | |
For substitutional cases, the bond lengths between the metal and O atoms are labeled in Fig. 2(f)–(k). Optimized doping positions of Li and Be are the same, and the bond length of Li–O is slightly longer than Be–O, while shorter than the average length of Ga–O (1.904 Å), which is in accordance with the atomic radius relation (Ga > Li > Be). In addition, other metal atoms can easily substitute Ga2 position. The local structure of Mg doping has a bit deformation, while the K doped case deforms a lot (unfavorable by formation energies), also due to the radius. As we can see from Fig. 3(c) and (d), for substitutional cases, all alkalis and alkalis-earth metals act as acceptors, and the doped systems show p-type character. In general, the spin up and spin down contributions for most metal atoms are slightly asymmetric, therefore, doped semiconductors may have applications in magnetic fields. In particular, for Na doped β-Ga2O3, the Na 3s orbitals split into 2 different states, one of which generates new states at the forbidden gap near the VBM. For K doped β-Ga2O3, the K 4s orbitals mix well with the O 2p orbitals at the VBM but produce a new state at the forbidden gap near the CBM.
For a further understanding on the chemical bonding between dopants and Ga2O3 crystal, we have also calculated the charge density differences for the most stable structures, as shown in Fig. 4. The data is obtained by the following equation:
|
Δρ = ρ(doped) − ρ(Ga2O3) − ρ(M)
| (4) |
where
ρ(doped) is the total charge density of the metal doped system,
ρ(Ga
2O
3) and
ρ(M) represent respectively the charge density of the undoped crystal and the charge density of the metal dopant. For interstitial dopings (
Fig. 4(a)), for Li, the electrons accumulate around the Li atoms along the Li–O bonds, which maybe caused by the strong attraction by the core. In Be doped crystal, the charge density is localized along both Be–Ga bonds and Be–O bonds. In Na and Mg doped cases, the electrons move from metal impurities to the O atoms, due to the reduction of the constraint on outermost electrons. For Li and Be substitutional doped β-Ga
2O
3, the optimized sites is similar and the electrons accumulate along the impurities and O atoms, which leads to the decrease of Li–O and Be–O bond lengths by comparison with Ga–O bonds. Na and Mg doped crystals show the depletion around the impurities, indicating the increase of the bond lengths. However, K and Ca doped cases (
Fig. 4(g) and (j)) show a different manner from Na and Mg, with the electrons locate between the impurities and the O atoms, which maybe caused by smaller electronegativities of K and Ca elements.
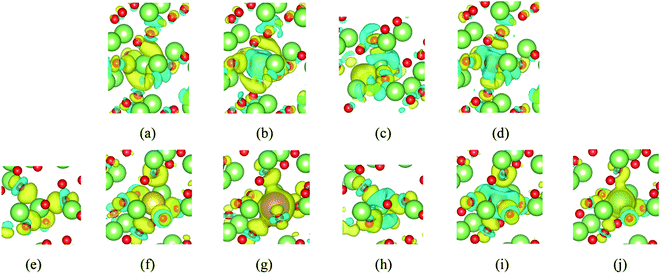 |
| Fig. 4 Charge density difference of interstitial doped β-Ga2O3 for (a) Li, (b) Na, (c) Be and (d) K, and of substitutional doping of β-Ga2O3 by (e) Li, (f) Na, (g) K, (h) Be, (i) Mg and (j) Ca. Red spheres represent O atoms and green spheres represent Ga atoms. Yellow and blue areas are the electronic accumulation and depletion regions, respectively. | |
3.3.2 Transition metals. We include Cu, Ag, Au, Zn, Cd, Hg, Ti, Mn, Co, Ni, Pd and part of lanthanides (Nd, Eu, Gd, Tb, Dy) in this group. The optimized local structures for each case are shown in Fig. 5. All optimized structures show different levels of inflation comparing with bulk β-Ga2O3, and bond lengths between O3 atoms and the impurities shown the largest extension than others. The bond lengths of the atoms with outermost 3d orbital are close to the undoped bulk β-Ga2O3, whereas the structures of lanthanides doped crystals produce large distortion. When comparing Ag doped with Au doped crystals (Fig. 5(g) and (h)), the average bond lengths between the impurities and the O atoms, 2.123 Å for Ag and 2.143 Å for Au, which show small difference, and the same rule holds for Cd and Hg doped case (Fig. 5(j) and (k)), which is mainly due to the effect of lanthanide contraction.
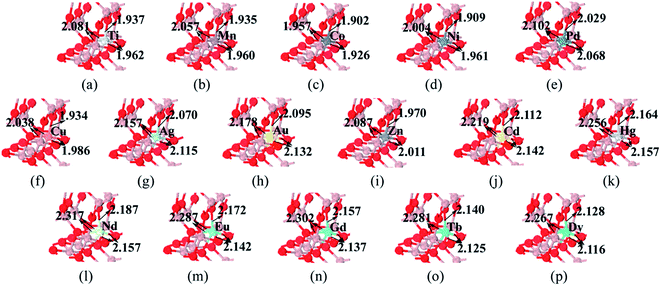 |
| Fig. 5 Optimized local structures of substitutional doping for (a) Ti, (b) Mn, (c) Co, (d) Ni, (e) Pd, (f) Cu, (g) Ag, (h) Au, (i) Zn, (j) Cd, (k) Hg, (l) Nd, (m) Eu, (n) Gd, (o) Tb and (p) Dy. Some bond lengths are labeled in the figure. Red spheres represent O atoms and pink spheres represent Ga atoms. | |
Fig. 6 shows the total and partial density of states of Ga2O3 doped by transition metals. First of all, band gaps of all transition metals doped Ga2O3 declines with respect to the undoped bulk crystal, due to the new states of metal atoms introduced into the forbidden gap. For most doped systems, the density of states is significantly asymmetric between spin-up and spin down cases, especially for Ti, Mn, Co, Ni and Pd doped cases, which may lead to applications in magnetic fields. The density of states are similar for dopings by elements in the same subgroup (see Fig. 6(c) and (d)), with Au 5d orbitals are easier to produce states in forbidden gap than Cu 3d and Ag 4d. Comparing doped crystals by different subgroup atoms (from Ti to Zn), the states introduced by the dopants shift from the CBM to VBM as the number of the outmost electrons increase. Therefore, the Ti and Nd doped β-Ga2O3 show obvious n-type characteristics, while Cu, Ag, Au, Zn, Cd and Hg (however, Au and Hg are unfavorable by formation energies) doped cases show obvious p-type characteristics. Moreover, as seen from Fig. 6(d), the density of states for Zn, Cd and Hg doped cases are similar and the partial density of states of the dopants show little contribution on the VBM. In addition, the total and partial density of states of Tb and Dy doped β-Ga2O3 are close to the undoped crystal, because the 4f orbitals of Tb and Dy show no contribution on the doped systems, and other orbitals (such as 3d orbitals shown in Fig. 6(f)) affect the deep-level. This is different from Nd, Eu and Gd, whose f orbitals all show contributions at the CBM and VBM.
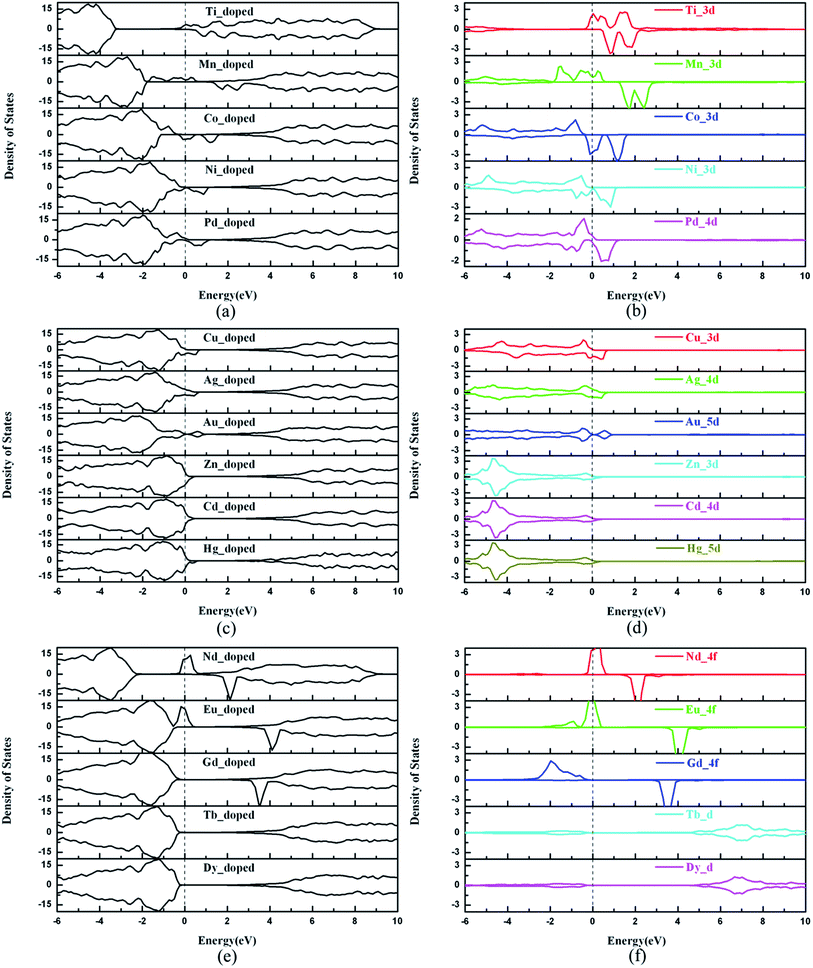 |
| Fig. 6 Total and partial density of states: (a) and (b) for Ti, Mn, Co, Ni, Pd; (c) and (d) for Cu, Ag, Au, Zn, Gd, Hg; (e) and (f) for lanthanides, respectively. The vertical dashed line represents the Fermi level which has been set to zero. | |
Fig. 7 gives the information on the charge density differences of the transition metal doped β-Ga2O3. In general, the electrons deplete along the impurities and the O atoms, especially in Cu, Ag, Au, Zn, Cd and Hg (see Fig. 7(f)–(k)), indicating the increase of the bond lengths. Conditions for the lanthanides doping are much more complicated, due to the effect from f-orbital electrons. The electrons of the lanthanides doped cases accumulate between the impurities and the O atoms but deplete between the impurities and the nearest Ga atoms.
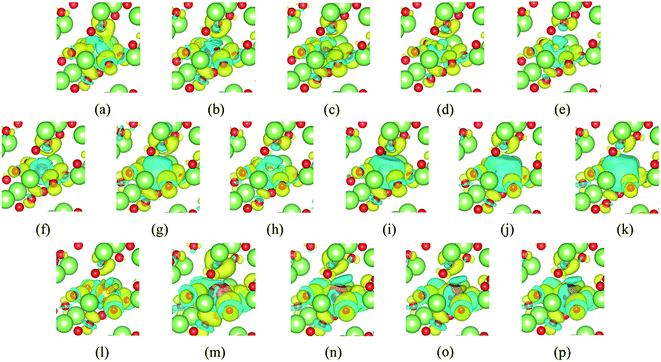 |
| Fig. 7 Charge density difference of substitutional doped β-Ga2O3 for (a) Ti, (b) Mn, (c) Co, (d) Ni, (e) Pd, (f) Cu, (g) Ag, (h) Au, (i) Zn, (j) Cd, (k) Hg, (l) Nd, (m) Eu, (n) Gd, (o) Tb and (p) Dy. Red spheres represent O atoms and green spheres represent Ga atoms. Yellow and blue areas are the electron accumulation and depletion regions, respectively. | |
3.3.3 Group IIIA and IVA. We consider In, Tl, Sn, Pb in this group, with Fig. 8 and 9 show the optimized local structures and the total and partial density of states, respectively. For doped cases by the same group elements, such as In and Tl (Fig. 8(a) and (b)), larger radii of In (1.67 Å) and Tl (1.71 Å) compared to Ga (1.53 Å) lead them a slight movement out of the octahedral. As we can see from Fig. 8, the bond lengths of In–O (2.175 Å, 2.085 Å and 2.098 Å), Tl–O (2.296 Å, 2.192 Å and 2.176 Å), Sn–O (2.104 Å, 2.023 Å and 2.032 Å) and Pb–O (2.289 Å, 2.192 Å and 2.176 Å) are all lager than the Ga–O bonds (2.069 Å, 1.950 Å and 1.970 Å). Therefore, we conclude that the distortion of the doped structures is related with the dopant radii, which is similar with above discussions.
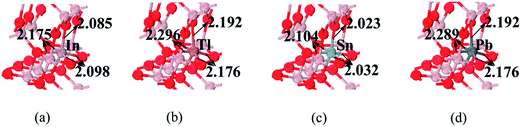 |
| Fig. 8 Optimized local structures of substitutional doping for (a) In, (b) Tl, (c) Sn and (d) Pb. Some bond lengths are labeled in the figure. Red spheres represent O atoms and pink spheres represent Ga atoms. | |
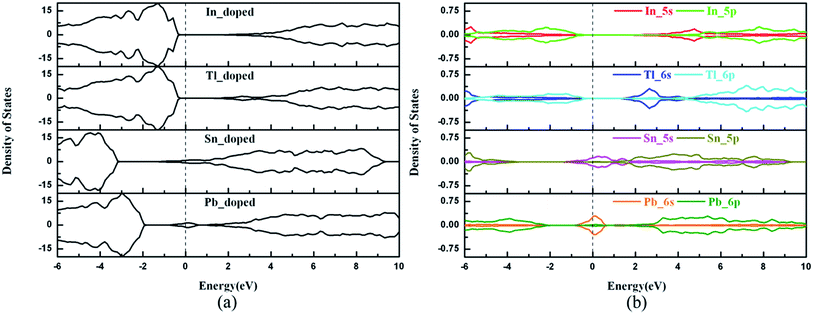 |
| Fig. 9 Total (a) and partial (b) density of states for substitutional doping of In, Tl, Sn and Pb, respectively. The vertical dashed line represents the Fermi level which has been set to zero. | |
In Fig. 9, the outermost electrons of these two group elements are filled in both s and p orbitals, and both have contributions to the density of states of the doped systems. For In doped case, the orbitals of the dopant mix well with the bulk β-Ga2O3 orbitals at the VBM and CBM, therefore In atom is a deep-level dopant. For Tl, Sn and Pb doped crystals, the s orbitals of the dopants produce new states in the forbidden gap near the CBM. Sn and Pb act as donors, which show n-type characteristics, whereas In and Tl give p-type potentials. The VBM of In and Tl doped cases remains almost unchanged with respect to the undoped crystal, whereas the CBM of them are shifted downwards, mainly due to the contribution of s orbitals. Due to the much higher energy of Tl 6s compared to Ga 3s orbitals, the highest occupied level of Tl doped cases moves downwards significantly by 0.60 eV (0.12 eV for In doped case). Sn and Pb doped cases show the same conditions. As a result, band gaps of In, Tl, Sn and Pb doped β-Ga2O3 are respectively 1.75 eV, 1.27 eV, 1.51 eV and 1.03 eV (4.78 eV, 4.30 eV, 4.54 eV and 4.06 eV after artificial bandgap correction). Fig. 10 shows the charge density difference diagrams for In, Tl, Sn and Pb doped crystals, which reveal the strong depletion of electron density between the impurities and the O atoms, leading to the distortion of the doped systems.
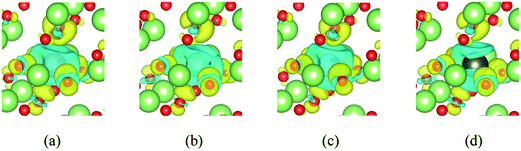 |
| Fig. 10 Charge density difference of substitutional doped β-Ga2O3 for (a) In, (b) Tl, (c) Sn and (d) Pb. Red spheres represent O atoms and green spheres represent Ga atoms. Yellow and blue areas are the electron accumulation and depletion regions, respectively. | |
3.4 Optical properties
For optical applications, for example as solar-blind ultraviolet photo-detector, absorption spectrum can always provide useful information. Fig. 11 shows the absorption spectra of wavelength from 0 nm to 400 nm for the metal doped crystals, and the absorption spectrum for undoped Ga2O3 is also shown for comparison. Specially, due to the underestimation of the band gap by using PBE functional computation, we artificially added the correction of the band gap in the spectrum.
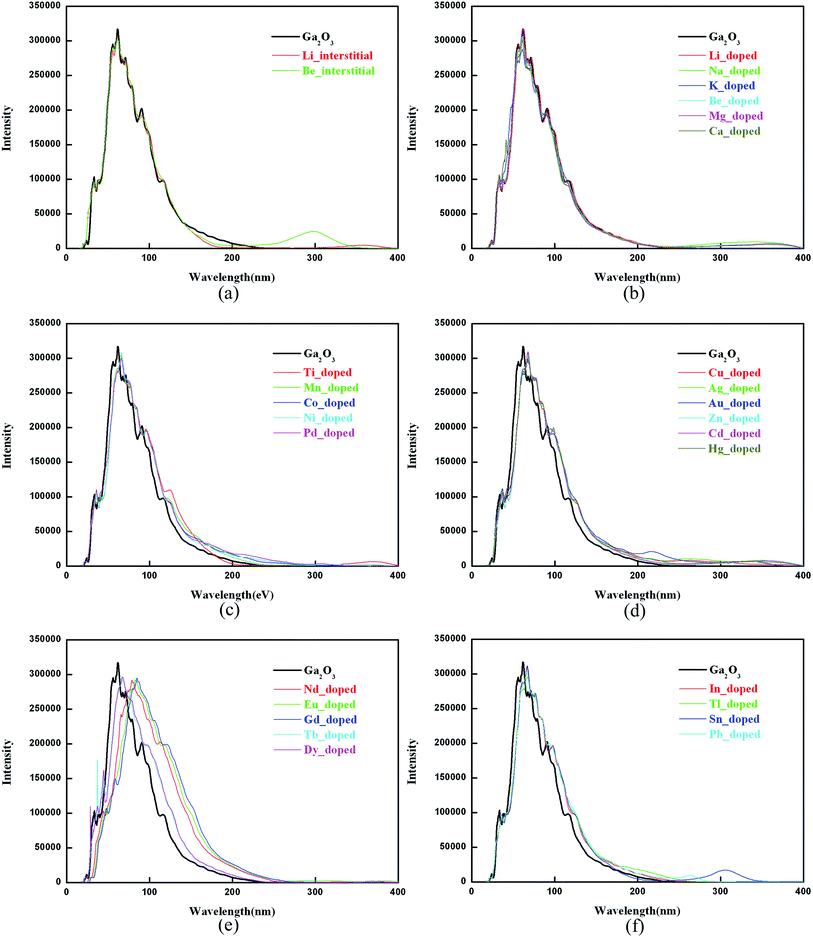 |
| Fig. 11 Absorption spectra of wavelength from 0 nm to 400 nm for (a) Li and Be atoms interstitial doped β-Ga2O3, (b) Li, Na, K, Be, Mg and Ca doped β-Ga2O3, (c) Ti, Mn, Co, Ni and Pd doped β-Ga2O3, (d) Cu, Ag, Au, Zn, Cd and Hg doped β-Ga2O3, (e) Nd, Eu, Gd, Tb and Dy doped β-Ga2O3, (f) In, Tl, Sn and Pb doped β-Ga2O3, respectively. The correction of the band gap has been added in the figure. | |
For alkali and alkaline earth metals doped cases, major peaks of the absorption spectra overlaps well with those of the undoped β-Ga2O3 for both interstitial and substitutional cases, indicating little influence of these dopants on major optical properties. Differences appear in the spectral range longer than 150 nm, where the absorption of Li interstitial doped crystal decreases to zero around 180 nm and the intensity of Be interstitial doping starts a steady increase after 220 nm, due to the more contribution of s orbitals than Li atom at the CBM. In Fig. 11(b), all substitutional conditions by these elements show absorptions above 250 nm. Major peaks of the absorption spectrum are red-shifted for transition metal doped cases, due to the narrowing of the band gap after doping. Nd, Eu and Gd doped systems show the largest shifts resulting from new states produced by f orbitals in the forbidden gap. All major peaks of the absorption spectra of doped β-Ga2O3 in Fig. 11(f) are also slightly red-shifted. As a result, major absorption peaks are red-shifted in most metal doped β-Ga2O3 but still located at the wavelength less than 200 nm, indicating potential applications in deep UV optoelectronic devices.
3.5 GGA+U and HSE result
In order to prove the reliability of the GGA results, we have calculated the formation energies of some p-type doped β-Ga2O3 by GGA+U and HSE methods and listed the results in Table 3. Overall, both GGA+U and HSE predict higher formation energies but the same trend with respect to the GGA results. According to Table 3, the O-rich condition is favorable for doping in comparison with the Ga-rich condition. Ga2 atoms are easier to be substituted by other metal dopants in most cases, except for Zn doped case which shows similar formation energies in the two sites. These results are well consistent with those predicted by GGA, indicating that different computational methods show little effect on our comparison study on different dopants, sites, and conditions.
Table 3 Formation energies of Mg, Cu, and Zn substitutional doped β-Ga2O3 under Ga-rich and O-rich conditions by GGA+U and HSE methods, respectively
Ef (eV) |
GGA+U |
HSE |
Ga-rich |
O-rich |
Ga-rich |
O-rich |
Mg_1 |
3.42 |
−1.66 |
3.24 |
−1.81 |
Mg_2 |
2.61 |
−2.46 |
2.94 |
−2.21 |
Cu_1 |
8.31 |
3.23 |
7.22 |
2.15 |
Cu_2 |
7.28 |
2.20 |
6.03 |
0.95 |
Zn_1 |
5.47 |
0.40 |
4.25 |
−0.82 |
Zn_2 |
5.59 |
0.52 |
4.83 |
−0.60 |
TDOS and PDOS of undoped β-Ga2O3 calculated by both GGA+U and HSE methods are listed in Fig. 12. The band gaps of undoped β-Ga2O3 have been predicted respectively to be 4.89 eV and 4.86 eV by GGA+U and HSE, which are in good agreement with the experimental value. As we can see from Fig. 12 and 1(b), the electronic structures obtained by different methods are similar. The VBM consists of O 2p, Ga 4p and Ga 4s states, whereas the CBM is contributed by Ga 4s states. For further comparison, the TDOS and PDOS of Mg, Cu and Zn doped crystal are also calculated by GGA+U and HSE and the results are listed in Fig. 13. From the figure, the states introduced by the dopants show slightly difference, especially in the forbidden gap. For HSE results, the Mg and Zn doped cases exhibit new states in forbidden gap, mainly caused by the dopants. However, in general, the calculated TDOS of these metal doped crystals also show the p-type conductivity. Therefore, calculations with different methods reach the same conclusion, indicating the reliability of our main discussions based on GGA.
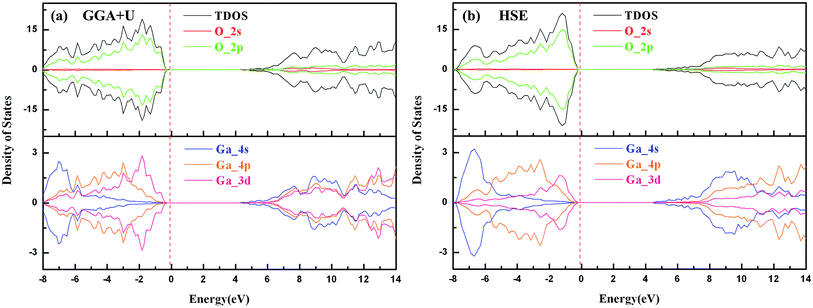 |
| Fig. 12 The total and partial density of states of bulk undoped β-Ga2O3 obtained by (a) GGA+U and (b) HSE methods, respectively. The red vertical dashed line is the Fermi level which has been set to zero. | |
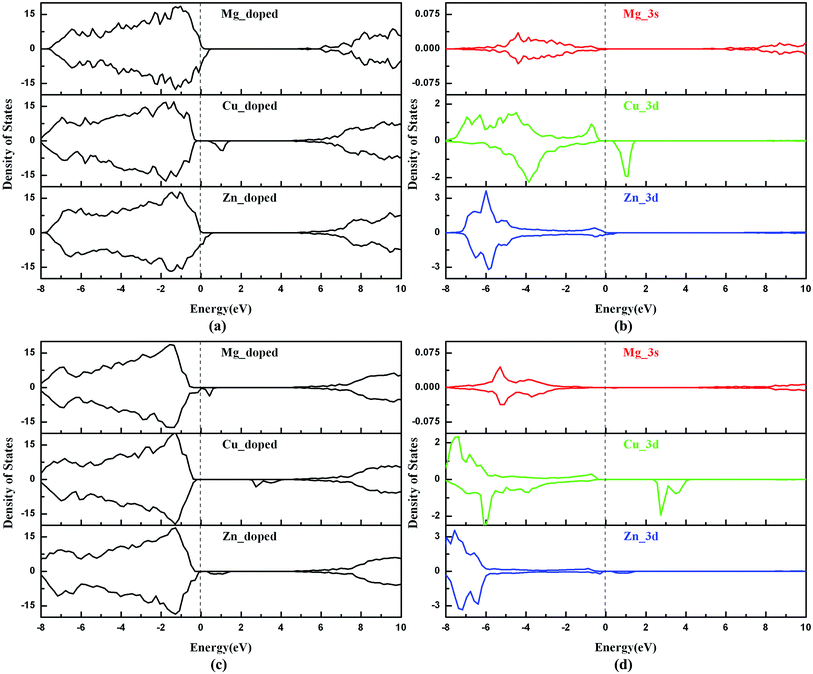 |
| Fig. 13 (a) Total and (b) partial density of states of Mg-, Cu-, Zn-doped β-Ga2O3 obtained by GGA+U method; (c) total and (d) partial density of states of Mg-, Cu-, Zn-doped β-Ga2O3 obtained by HSE method. The vertical dashed line is the Fermi level which has been set to zero. | |
4 Conclusions
In present paper, first principles studies have been performed on formation energies, electronic structures and optical properties of metal doped β-Ga2O3, in order to search for potential p-type dopants. A long list of metals have been well examined, including Li, Na, K, Be, Mg, Ca, Ti, Mn, Co, Ni, Pb, Cu, Ag, Au, Zn, Cd, Hg, Nd, Eu, Gd, Tb, Dy, In, Tl, Sn and Pb. The reliability of our discussions based on GGA predictions have been well addressed by detailed comparison with GGA+U and HSE methods. Calculations indicate that O-rich condition is more preferred for metal-doping. From the location of the Fermi level relative to VBM and CBM, one can easily determine the n- and p-type of the semiconductor. Results show that Li and Be prefer interstitial rather than substitutional doping due to their small radii, which will eventually give them n-type characteristics. This provides a possible explanation for the n-type nature of doped β-Ga2O3 by small radii atoms. Na, Mg, Ca, Cu, Ag, Zn, Cd doped β-Ga2O3 show a potential in the p-type crystal fabrication, which should be further examined in experiments. However, it is always not easy at all to get high carrier concentrations, due to high self-compensation effect and low activation rate of dopants. Studies on determination and elimination of n-type background carriers, and effective activation strategies are on-going in our group, both in theory and experiment. By the way, for some transition metal dopants, such as Mn, Co and some lanthanides, our calculation show asymmetric density of states for spin-up and spin-down states, which may lead potential applications as magnetic devices. Absorption spectra show that both undoped and doped β-Ga2O3 crystals are all suitable for deep UV optoelectronic applications, with most doped cases show red-shift with respect to the undoped crystal, due to introduction of new states in the forbidden gap by dopants.
Acknowledgements
We acknowledge the National Nature Science Foundation of China (Grant No. 21573129), the National Nature Science Foundation of Shandong Province (Grant No. ZR2015BQ001), and the General Financial Grant from the China Postdoctoral Science Foundation (Grant No. 2013M531595). Z. Jia wishes to thank the Young Scholars Program of Shandong University (No. 2015WLJH36), and State Key Laboratory of Optoelectronic Materials and Technologies (Sun Yat-sen University) for the financial support (No. OEMT-2015-KF-06). A generous grant of computer time from the National Supercomputer Center in Tianjin-TianHe-1(A) and the Norwegian Programme for Supercomputing are also well acknowledged.
References
- R. Roy, V. Hill and E. Osborn, J. Am. Chem. Soc., 1952, 74, 719 CrossRef CAS.
- H. He, R. Orlando, M. A. Blanco, R. Pandey, E. Amzallag, I. Baraille and M. Rerat, Phys. Rev. B: Condens. Matter Mater. Phys., 2006, 74, 195123 CrossRef.
- F. Litimein, D. Rached, R. Khenata and H. Baltache, J. Alloys Compd., 2009, 488, 148 CrossRef CAS.
- N. Ueda, H. Hosono, R. Waseda and H. Kawazoe, Appl. Phys. Lett., 1997, 71, 933 CrossRef CAS.
- H. Tippins, Phys. Rev., 1965, 140, A316 CrossRef.
- Y. Hou, L. Wu, X. Wang, Z. Ding, Z. Li and X. Fu, J. Catal., 2007, 250, 12 CrossRef CAS.
- M. Zinkevich and F. Aldinger, J. Am. Ceram. Soc., 2004, 87, 683 CrossRef CAS.
- N. Ueda, H. Hosono, R. Waseda and H. Kawazoe, Appl. Phys. Lett., 1997, 70, 3561 CrossRef CAS.
- R. Suzuki, S. Nakagomi, Y. Kokubun, N. Arai and S. Ohira, Appl. Phys. Lett., 2009, 94, 222102 CrossRef.
- M. Higashiwaki, K. Sasaki, T. Kamimura, M. H. Wong, D. Krishnamurthy, A. Kuramata, T. Masui and S. Yamakoshi, Appl. Phys. Lett., 2013, 103, 123511 CrossRef.
- M. Higashiwaki, K. Sasaki, A. Kuramata, T. Masui and S. Yamakoshi, Phys. Status Solidi A, 2014, 211, 21 CrossRef CAS.
- M. Higashiwaki, K. Sasaki, H. Murakami, Y. Kumagai, A. Koukitu, A. Kuramata, T. Masui and S. Yamakoshi, Semicond. Sci. Technol., 2016, 31, 034001 CrossRef.
- Z. Galazka, K. Irmscher, R. Uecker, R. Bertram, M. Pietsch, A. Kwasniewski, M. Naumann, T. Schulz, R. Schewski, D. Klimm and M. Bickermann, J. Cryst. Growth, 2014, 404, 184 CrossRef CAS.
- Z. Galazka, R. Uecker, K. Irmscher, M. Albrecht, D. Klimm, M. Pietsch, M. Bruetzam, R. Bertram, S. Ganschow and R. Fornari, Cryst. Res. Technol., 2010, 45, 1229 CrossRef CAS.
- E. G. Villora, K. Shimamura, Y. Yoshikawa, K. Aoki and N. Ichinose, J. Cryst. Growth, 2004, 270, 420 CrossRef CAS.
- J. B. Varley, J. R. Weber, A. Janotti and C. G. Van de Walle, Appl. Phys. Lett., 2010, 97, 142106 CrossRef.
- X. Du, Z. Li, C. Luan, W. Wang, M. Wang, X. Feng, H. Xiao and J. Ma, J. Mater. Sci., 2015, 50, 3252 CrossRef CAS.
- T. C. Lovejoy, R. Chen, X. Zheng, E. G. Villora, K. Shimamura, H. Yoshikawa, Y. Yamashita, S. Ueda, K. Kobayashi, S. T. Dunham, F. S. Ohuchi and M. A. Olmstead, Appl. Phys. Lett., 2012, 100, 181602 CrossRef.
- P. C. Chang, Z. Y. Fan, W. Y. Tseng, A. Rajagopal and J. G. Lu, Appl. Phys. Lett., 2005, 87, 222105 CrossRef.
- L. L. Liu, M. K. Li, D. Q. Yu, J. Zhang, H. Zhang, C. Qian and Z. Yang, Appl. Phys. A: Mater. Sci. Process., 2010, 98, 831 CrossRef CAS.
- X. Feng, Z. Li, W. Mi and J. Ma, Vacuum, 2016, 124, 101 CrossRef CAS.
- Z. Wu, G. Bai, Q. Hu, D. Guo, C. Sun, L. Ji, M. Lei, L. Li, P. Li, J. Hao and W. Tang, Appl. Phys. Lett., 2015, 106, 171910 CrossRef.
- D. Wang, Y. Lou, R. Wang, P. Wang, X. Zheng, Y. Zhang and N. Jiang, Ceram. Int., 2015, 41, 14790 CrossRef CAS.
- R. Lorenzi, A. Paleari, N. V. Golubev, E. S. Ignat'eva, V. N. Sigaev, M. Niederberger and A. Lauria, J. Mater. Chem. C, 2015, 3, 41 RSC.
- Z. Chen, K. Saito, T. Tanaka, M. Nishio, M. Arita and Q. Guo, J. Cryst. Growth, 2015, 430, 28 CrossRef CAS.
- Y. Wang, K. Xu, D. Li, H. Zhao and Z. Hu, Opt. Mater., 2014, 36, 1798 CrossRef CAS.
- M. Bagheri, A. R. Mahjoub, A. A. Khodadadi and Y. Mortazavi, RSC Adv., 2014, 4, 33262 RSC.
- X. Wang, S. Shen, S. Jin, J. Yang, M. Li, X. Wang, H. Han and C. Li, Phys. Chem. Chem. Phys., 2013, 15, 19380 RSC.
- N. K. Shrestha, K. Lee, R. Kirchgeorg, R. Hahn and P. Schmuki, Electrochem. Commun., 2013, 35, 112 CrossRef CAS.
- B. K. Kang, S. R. Mang, D. H. Go and D. H. Yoon, Mater. Lett., 2013, 111, 67 CrossRef CAS.
- A. A. Dakhel, Solid State Sci., 2013, 20, 54 CrossRef CAS.
- Y. Tokida and S. Adachi, J. Appl. Phys., 2012, 112, 063522 CrossRef.
- J. Zhao, W. Zhang, E. Xie, Z. Ma, A. Zhao and Z. Liu, Appl. Surf. Sci., 2011, 257, 4968 CrossRef CAS.
- J. Zhao, W. Zhang, E. Xie, Z. Liu, J. Feng and Z. Liu, Mater. Sci. Eng., B, 2011, 176, 932 CrossRef CAS.
- A. A. Dakhel, J. Mater. Sci., 2011, 47, 3034 CrossRef.
- F. Zhang, K. Saito, T. Tanaka, M. Nishio and Q. Guo, J. Mater. Sci.: Mater. Electron., 2015, 26, 9624 CrossRef CAS.
- R. Sun, H.-Y. Zhang, G.-G. Wang, J.-C. Han, X.-Z. Wang, L. Cui, X.-P. Kuang, C. Zhu and L. Jin, Superlattices Microstruct., 2014, 65, 146 CrossRef CAS.
- Y. Zhang, J. Yan, Q. Li, C. Qu, L. Zhang and T. Li, Phys. B, 2011, 406, 3079 CrossRef CAS.
- W. Guo, Y. Guo, H. Dong and X. Zhou, Phys. Chem. Chem. Phys., 2015, 17, 5817 RSC.
- G. Pei, C. Xia, Y. Dong, B. Wu, T. Wang and J. Xu, Scr. Mater., 2008, 58, 943 CrossRef CAS.
- W.-Z. Xiao, L.-L. Wang, L. Xu, Q. Wan and B. S. Zou, Scr. Mater., 2009, 61, 477 CrossRef CAS.
- T. C. Lovejoy, R. Chen, E. N. Yitamben, V. Shutthanadan, S. M. Heald, E. G. Villora, K. Shimamura, S. Zheng, S. T. Dunham, F. S. Ohuchi and M. A. Olmstead, J. Appl. Phys., 2012, 111, 123716 CrossRef.
- Y. Guo, H. Yan, Q. Song, Y. Chen and S. Guo, Comput. Mater. Sci., 2014, 87, 198 CrossRef CAS.
- H. Yan, Y. Guo, Q. Song and Y. Chen, Phys. B, 2014, 434, 181 CrossRef CAS.
- S. W. Zheng, G. H. Fan, M. He and L. Z. Zhao, Acta Physica Sinica, 2014, 63, 196101 Search PubMed.
- J. P. Perdew, K. Burke and M. Ernzerhof, Phys. Rev. Lett., 1996, 77, 3865 CrossRef CAS PubMed.
- G. Kresse and J. Furthmüller, Phys. Rev. B: Condens. Matter Mater. Phys., 1996, 54, 11169 CrossRef CAS.
- G. Kresse and J. Furthmüller, Comput. Mater. Sci., 1996, 6, 15 CrossRef CAS.
- G. Kresse and D. Joubert, Phys. Rev. B: Condens. Matter Mater. Phys., 1999, 59, 1758 CrossRef CAS.
- S. Geller, J. Chem. Phys., 1960, 33, 676 CrossRef CAS.
- V. I. Anisimov, F. Aryasetiawan and A. Lichtenstein, J. Phys.: Condens. Matter, 1997, 9, 767 CrossRef CAS.
- S. Dudarev, G. Botton, S. Savrasov, C. Humphreys and A. Sutton, Phys. Rev. B: Condens. Matter Mater. Phys., 1998, 57, 1505 CrossRef CAS.
- J. Heyd, G. E. Scuseria and M. Ernzerhof, J. Chem. Phys., 2003, 118, 8207 CrossRef CAS.
- J. Heyd, G. E. Scuseria and M. Ernzerhof, J. Chem. Phys., 2006, 124, 8207 CrossRef.
- J. Atanelov and P. Mohn, Phys. Rev. B: Condens. Matter Mater. Phys., 2015, 92, 104408 CrossRef.
- W. Lei, T. Maxisch and G. Ceder, Phys. Rev. B: Condens. Matter Mater. Phys., 2006, 73, 195107 CrossRef.
- V. Anisimov and O. Gunnarsson, Phys. Rev. B: Condens. Matter Mater. Phys., 1991, 43, 7570 CrossRef CAS.
|
This journal is © The Royal Society of Chemistry 2016 |