DOI:
10.1039/C6RA13855A
(Paper)
RSC Adv., 2016,
6, 72999-73005
Design and preparation of silica tube/poly(aryl ether ketone) composites with low dielectric constant
Received
28th May 2016
, Accepted 22nd July 2016
First published on 25th July 2016
Abstract
Silica tubes with uniform size were designed and prepared by hydrolyzing tetraethyl orthosilicate using D,L-tartaric acid as the self-assembly template. And a novel series of silica tube/poly(aryl ether ketone) composite films were prepared in which silica tubes were covalently bonding onto the side chains of poly(aryl ether ketone) (PAEK-COOH) in the presence of silane coupling agent (γ-aminopropyltriethoxysilane, KH-550). The morphology of the silica tubes was observed by scanning electron microscopy (SEM) and transmission electron microscopy (TEM). The chemical structures of the polymer matrix and composites were confirmed by 1H NMR and FT-IR spectra. The patterns of wide-angle X-ray diffraction (WAXD) and X-ray photoelectron spectra (XPS) indicated that the silica tubes were incorporated into the PAEK successfully and the homogeneous dispersion of silica tubes in PAEK was illustrated by scanning electron microscopy (SEM). Furthermore, the impact of silica tubes on the properties of composites was investigated in detail. The composite films exhibited low dielectric constants ranging from 2.22 to 2.57 at 1 MHz owing to the introduction of silica tubes, which was found to be distinctly lower than that of the pristine PAEK-COOH film. Meanwhile, these composites still retained excellent thermal and mechanical properties.
Introduction
Over the last two decades, extensive attention has been paid to the applications of one-dimensional nanomaterials, such as nanotubes, nanofibers and nanowires, and so forth. These applications include chemical probes, nanoprobes, sensors, hydrogen storage devices, and displays.1–4 Recently, numerous new tubular nanomaterials, such as SiO2,5,6 V2O5,7–9 and MoS2,10–14 have been reported. These tubular materials have large specific surface areas and hollow structures, which make them potentially suitable for applications as catalysts, storage devices and templates to grow other nanosized materials. One of the new potential applications is to incorporate the hollow tubes into a polymer matrix to reduce the total dielectric constant of the composite, in consideration of the low dielectric constant of air (κ = 1),15–20 the incorporation of controllable porosity in materials has been envisioned as a promising method for developing low dielectric materials.21–29
Poly(aryl ether ketone) (PAEK) is an important species of high performance thermoplastics characterized by the excellent mechanical properties, high-temperature durability as well as low dielectric constant (κ = 3.2–3.3).30–33 To further reduce its dielectric constant, and retain the superior mechanical and thermal properties as far as possible, the silica tubes can be incorporated into the PAEK signify the air introduced. Silica is a kind of possible candidates to play a role of dielectric properties' modifier for its intrinsic low dielectric constant, good thermal properties as well as numerous hydroxyl attached on its surface which can be utilized to generate the dehydration condensation reaction with polymer.
The introduction of voids decreases the dielectric constant by lowering the density of the materials. In principle, one can vary the percentage of the porosity and therefore the material density and dielectric constant. The voids are incorporated into a network material that can be organic, inorganic, or a hybrid material. Because of the introduction of voids will compromise other material properties such as mechanical strength, the chemical structure of the porous network must be carefully designed to achieve sufficient thermomechanical stability. The incorporation of air, which has a dielectric constant of about 1, can greatly reduce the dielectric constant of the resulting porous structure. The conventional methods to prepare the porous materials or composites are restricted by various conditions. Such as the thermal decomposition of the labile components in the block copolymers or side chains of polymer and high-pressure CO2 gas injection, most of the materials prepared by these methods have large pore sizes, open pore structures and sacrifice the mechanical properties of materials.34,35 Also the introduction of porous nanoparticles into polymer matrix is another method, but most of the nanoparticles suffer from low porosity that could not effectively reduce the dielectric constant of polymer matrix.36 Silica tubes is high thermostability and high porosity, as well as tubular structure, which are beneficial to prepare the dielectric materials. On other hand, fluorine containing polymers have high thermal stability, improved chemical resistance and lower surface energy when compared to their non-fluorinated counterparts. The small size of the fluorine atom with its 2s and 2d electrons close to the nucleus is the most electronegative element. Therefore, the introduction of fluorine substituent into the polymer is regarded as effective ways to obtain a low dielectric constant property due to the low polarizability of the C–F bond. In light of this, herein we report a new method to prepare porous composites with low dielectric constant. The poly(aryl ether ketone) with carboxyl groups pendants and (3-trifluoromethyl) phenyl groups (PAEK-COOH) low dielectric constant polymer matrix was synthesized by copolymerization of 4,4′-difluorobenzophenone (DFB), (3-trifluoromethyl) phenyl hydroquinone (3FHQ) and phenolphthalin (PPL), then a variety amount of silica tubes (STs) modified by the silane coupling agent (γ-aminopropyltriethoxysilane, KH-550) were covalently linked with acyl chloride groups by the tethering onto the side chain to prepare a series of novel ST–PAEK-COOH composite films. Additionally, the influence of the content of silica tubes on the dielectric, thermal and mechanical properties of the ST–PAEK-COOH composites was also comparatively investigated.
Experimental
Materials
Tetramethylene sulfone (TMS) was supplied from Jinzhou Oil Refinery and purified by distillation under reduced pressure. Toluene, tetraethyl orthosilicate (TEOS) and D,L-tartaric acid were purchased from Sinopharm Chemical Reagent Co. Ltd. China. Tetrahydrofuran (THF) and dimethylacetamide (DMAc) were provided by Beijing Chemical Reagent and further purified in accordance with the standard procedures. Phenolphthalin (PPL) was obtained from TCI Shanghai Development Co. Ltd. China. 4,4′-Difluorobenzophenone (DFB) was gotten from Changzhou Huashan Chemical Co. Ltd. China and further purified by recrystallization. (3-Trifluoromethyl) phenyl hydroquinone (3FHQ) was prepared in our lab according to the report we have published before.37 All other common reagents were supplied from commercial sources and used as received.
Measurements
FT-IR spectra were carried out on a Nicolet Impact 410 Fourier transform infrared spectrophotometer in transmission with a resolution of 4 cm−1 on a KBr pellet. 1H NMR was measured on a Bruker 510 NMR spectrometer (500 MHz) using CDCl3 as solvent with a reference of tetramethylsilane. X-ray diffraction (XRD) patterns were collected on a Japan Rigaku SmartLab X-ray diffractometer (D/max-2500, by using Cu Kα radiation at λ = 0.154 nm as the X-ray source), and the data were recorded from 5° to 40°. The chemical compositions of the polymer matrix and composite films were recorded on X-ray photoelectron spectra (ESCALAB 250 X-ray photoelectron spectrometer) using a monochromic X-ray source (Al Kα line, 1486.6 eV) and the charging shift was corrected by the binding energy of C (1s) at 284.6 eV. The microstructure and dispersion of the silica tubes in the polymer matrix were observed from JEM-1200EX transmission electron microscopy (TEM) and HITACHI-SU8020 scanning electron microscope (SEM), respectively. The dielectric constants of these films (circles with the thickness about 0.1 mm and a diameter of 5.5 mm, prepared by casting from DMAc solvents, then coating with silver by a vacuum evaporation method) were carried out on a Hewlett-Packard 4285A apparatus at the frequency range 103–106 Hz at room temperature. The thermal properties of materials were performed via thermal gravimetric analyses (TGA) experiments using a heating rate of 10 °C min−1 in nitrogen atmosphere on a Perkin Elmer Pyris 1 TGA analyzer. The mechanical properties of these rectangular films (45.0 (length) × 5.0 (width) × 0.1 (thickness) mm3, casting from DMAc) were evaluated at a strain rate of 10 mm min−1 on a Shimadzu AG-I Universal Tester at room temperature (25 °C), and all the composite films were measured for at least four times in parallel.
Synthesis of silica tubes by self-assembly
The silica tubes were synthesized by hydrolyzing the tetraethyl orthosilicate using D,L-tartaric acid as the template in accordance with the previous reports.5,38 The synthetic procedure was carried out as follows: 0.02 g of D,L-tartaric acid was entirely dissolved in 5 mL of ethanol, then 2 mL of aqueous ammonium was added into the resulting solution to generate the ammonium D,L-tartaric acid crystal template. Followed by 0.73 g of tetraethyl orthosilicate were dropwise added into the resulting solution for 1 h under magnetic stirring, and the resulting mixture was allowed to stand for 12 h at room temperature. The white product was thoroughly washed with distilled water on a 0.22 μm membrane filter to remove the ammonium D,L-tartaric acid crystal template and dried at 80 °C for 12 h.
Synthesis of PAEK-COOH fluoropolymer matrix
PAEK-COOH polymer matrix was synthesized via the copolymerization of (3-trifluoromethyl) phenyl hydroquinone (3FHQ), phenolphthalin (PPL) and 4,4′-difluorobenzophenone (DFB) as illustrated in Scheme 1. The synthetic procedure was carried out as follows: (3-trifluoromethyl) phenyl hydroquinone (3FHQ) (4.83 g, 0.019 mol), phenolphthalin (PPL) (0.32 g, 0.001 mol), 4,4′-difluorobenzophenone (DFB) (4.36 g, 0.020 mol) and anhydrous K2CO3 (2.90 g, 0.021 mol) were dissolved in TMS (36 mL) and toluene (20 mL) in a three necked flask equipped with a mechanical stirrer, a nitrogen inlet with a thermometer, and a Dean–Stark trap with a condenser. The mixture was heated under stirring and the system was allowed to reflux for 4 h at 125 °C under the nitrogen atmosphere to remove the resulting water. The reaction mixture was heated to 160–170 °C to complete the polymerization. After 6 h, the viscous solution was then poured into deionized water. The flexible threadlike polymer was pulverized into powder using a blender, and washed with boiling-water and ethanol several times thoroughly. The product was dissolved in 150 mL THF, and 5 mL condensed HCl was dropwise added to the solution followed by magnetic stirring the solution at room temperature for 24 h to ensure that all of the potassium carboxylate were converted to carboxyl groups completely. Then the polymer was precipitated in distilled water and washed several times, the product was dried to constant weight under vacuum at 80 °C for 48 h to give PAEK-COOH polymer matrix. Yield: 95%.
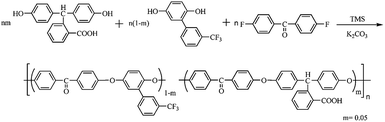 |
| Scheme 1 Synthesis of PAEK-COOH fluoropolymer matrix. | |
Preparation of ST–PAEK-COOH composite films
The composite films were prepared by solution casting and the preparation procedure of ST–PAEK-COOH-3 wt% composites was depicted in Scheme 2. Firstly, 1.0 g PAEK-COOH was dissolved in 10 mL anhydrous tetrahydrofuran (THF) at room temperature and three times mole amount (in comparison to the mole amount of carboxyl groups in polymer matrix) oxalyl chloride (33 μL) was added, the solution was stirred at room temperature for 12 h ensuring that all of the carboxyl groups were completely converted to acyl chloride groups, then the solvent and the residual oxalyl chloride were removed by distillation under reduced pressure. Afterwards, the product was redissolved in anhydrous dimethylacetamide (DMAc) to get the polymer solution and the same mole amount (in comparison to the mole amount of acyl chloride groups in polymer matrix) γ-aminopropyltriethoxysilane (KH-550) (53 μL) was added into the polymer solution, the solution was stirred for 6 h at room temperature, then 0.03 g silica tubes and a three times mole amount of deionized water (12 μL) (in comparison to the mole amount of KH-550) was added into the solution, and the solution was continued to stir for another 24 h to obtain a homogeneous solution at room temperature. The resulting solution was casted onto glass plate and dried at 100 °C for 24 h, and then the temperature was increased to 120 °C for another 48 h to completely remove the solvent and resulting water. Finally, the ST–PAEK–COOH composite film was obtained. The preparation of other weight percentage (5 wt% and 7 wt%) ST–PAEK–COOH composite films were conducted using the same method described above, only changed the addition amount of silica tubes.
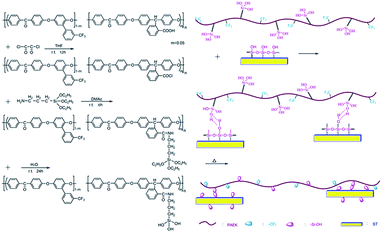 |
| Scheme 2 Preparation of ST–PAEK–COOH composite film. | |
Results and discussion
SEM and TEM characterization of silica tubes
Silica tubes with uniform size were prepared by hydrolyzing the tetraethyl orthosilicate at the existence of ammonium D,L-tartaric crystal template. Fig. 1(a) and (b) show the SEM and TEM images of the prepared silica tubes, respectively. From the SEM image of Fig. 1(a), a cubical structure of the silica tubes was observed. Meanwhile the hollow structure of the silica tubes can be clearly observed in the TEM image of Fig. 1(b). As shown in Fig. 1, the mean outer and inner sizes in cross-section length of the silica tubes are about 420 nm and 360 nm, respectively, the thickness of the wall is about 50–60 nm and the length is about 15–20 μm. Alongside the silica tubes, there are still some small quantities of silica particles. In the process of forming silica tubes, the tetraethyl orthosilicate was hydrolyzed into silica and then gradually attached onto the surface of ammonium D,L-tartaric templates to generate the silica tubes. The formation of ammonium D,L-tartaric template is almost simultaneous with the addition of aqueous ammonium. Since the crystal templates would grow further until the silica starts to deposit on the templates, the width of the tube would increase with increasing the ratio of crystal template growth rate along the width direction to the tetraethyl orthosilicate deposition rate. Therefore, the width of the silica tubes varies with different reaction conditions, tetraethyl orthosilicate concentrations and different acid, and so forth. This phenomenon can also be explained associated with the change in the rates of the silica deposition and template growth.39 On the other hand, the formation of hollow silica tubes in the presence of D,L-tartaric template is attributed to no silica deposition on the head faces of the crystal templates.
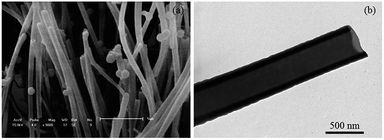 |
| Fig. 1 SEM (a) and TEM (b) images of the prepared silica tubes. | |
Structure characterization
The structure of synthesized PAEK-COOH polymer matrix was demonstrated by its 1H NMR spectra with CDCl3 as a solvent, as shown in Fig. 2. The coupling constant J and H number from the 1H NMR spectra of the synthesized PAEK-COOH are as follows: 8.054–8.067 (d, 1H, J = 6.637 Hz), 7.859–7.874 (d, 1H, J = 7.595 Hz), 7.665–7.790 (m, 9H), 7.552 (s, 1H), 7.471 (s, 1H), 7.258 (s, 1H), 7.113–7.217 (m, 11H), 7.004–7.014 (d, 8H, J = 5.458 Hz), 6.901–6.940 (t, 2H, J1 = 9.107 Hz, J2 = 10.5 Hz). Fig. 2 confirms that the PAEK-COOH polymer matrix has been synthesized successfully.
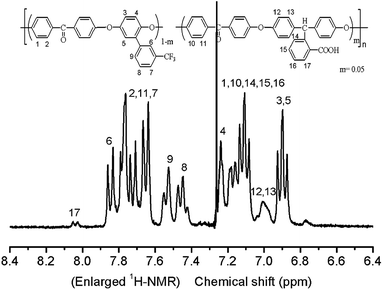 |
| Fig. 2 1H NMR spectrum of PAEK-COOH in CDCl3. | |
The structures of synthesized PAEK-COOH polymer matrix and corresponding ST–PAEK-COOH composites are further confirmed from their FT-IR spectra, as shown in Fig. 3. Fig. 3(a) confirms the chemical structure of synthesized PAEK-COOH: 1723 cm−1 (C
O bond of aromatic carboxyl groups in the side chain), 1653 cm−1 (C
O bond of aromatic ketone in the main-chain), 1598 cm−1 and 1500–1475 cm−1 (benzene ring), 1226 cm−1 (Ar–O–Ar in the main-chain), 1162 cm−1 and 1125 cm−1 (–CF3 groups). Fig. 3(b)–(d) confirm the chemical structures of prepared ST–PAEK-COOH: 1653 cm−1 (C
O bond of aromatic ketone in the main-chain), 1540 cm−1 (N–H bond of acid amide), 1598 cm−1 and 1500–1475 cm−1 (benzene ring), 1226 cm−1 (Ar–O–Ar in the main chain), 1162 cm−1 and 1125 cm−1 (–CF3 groups). In comparison with the FT-IR spectra, it can be found that the characteristic absorption peak at 1720 cm−1 of aromatic carboxyl groups almost disappears and the characteristic absorption peaks at 1540 cm−1 of amide groups (N–H bond) appears in the spectra of composites. In addition, a variation at 1096 cm−1 ascribed to the characteristic vibration of the Si–O–Si bond was observed. From the FT-IR spectra of the composites, the conclusion can be drawn that the silica tubes with KH-550 were covalent bonding onto the side chains of PAEK-COOH and the ST–PAEK-COOH composites were successfully prepared.
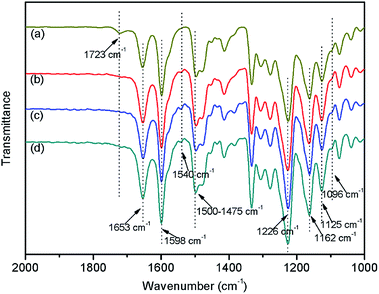 |
| Fig. 3 FT-IR spectra: (a) pure PAEK-COOH; (b) ST–PAEK-COOH-3 wt%; (c) ST–PAEK-COOH-5 wt%; (d) ST–PAEK-COOH-7 wt%. | |
The crystallization behaviour of PAEK-COOH and ST–PAEK-COOH composites
The dispersity of silica tubes within the PAEK-COOH polymer matrix has been demonstrated to have a tremendous influence on the mechanical and dielectric properties of the resultant composite films. The wide-angle X-ray diffraction patterns (WAXD) of silica tubes, neat PAEK-COOH and ST–PAEK-COOH composites were displayed in Fig. 4. The pattern of pure silica tubes exhibits two distinct diffraction peaks at 2θ = 5.6° and 22.8°, and the neat PAEK-COOH polymer matrix only exhibits a diffuse peak at 2θ = 18.5°, whereas the ST–PAEK-COOH composites exhibit two diffuse peaks at 2θ = 5.6° and 18.6°, respectively. The amorphous band of silica tubes at 22.8° cannot be found in the XRD pattern of the ST–PAEK-COOH composites, suggesting that the silica tubes within the polymer matrix did not generate macroscopic aggregation.
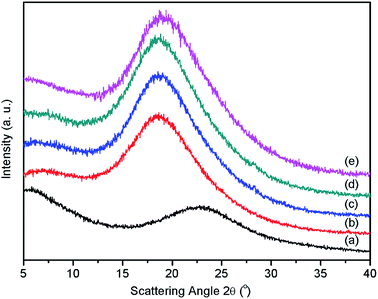 |
| Fig. 4 WAXD patterns of (a) silica tubes; (b) pure PAEK-COOH; (c) ST–PAEK-COOH-3 wt%; (d) ST–PAEK-COOH-5 wt%; (e) ST–PAEK-COOH-7 wt%. | |
The component analysis of PAEK-COOH and ST–PAEK-COOH composites
An X-ray photoelectron spectra (XPS) was utilized to expound the constituent of PAEK-COOH and ST–PAEK-COOH composites. It is evident that the PAEK-COOH polymer matrix contains the elements of F (691 eV, F 1s), O (531 eV, O 1s) and C (296 eV, C 1s) as shown in Fig. 5(a). In comparison, the spectra for ST–PAEK-COOH composites in Fig. 5(b)–(d) exhibit the additional new peaks at 101 eV for Si 2p, 153 eV for Si 2s and 398 eV for N 1s, respectively. Apparently, the pure PAEK-COOH polymer matrix only contains the elements of F, O and C, thus the additional new peaks of N and Si are derived from the introduction of KH-550 and silica tubes, which further suggests that the KH-550 with silica tubes are incorporated into the polymer matrix successfully.
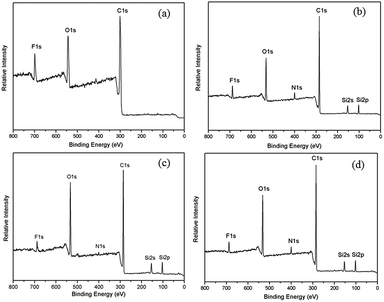 |
| Fig. 5 XPS wide scan spectra of (a) pure PAEK-COOH; (b) ST–PAEK-COOH-3 wt%; (c) ST–PAEK-COOH-5 wt%; (d) ST–PAEK-COOH-7 wt%. | |
The morphologies of ST–PAEK-COOH composite films
Scanning electron microscopy (SEM) is an intuitive and convenient method to observe the microstructure of the composite films. Fig. 6 presents the SEM photographs of the fracture surfaces of ST–PAEK-COOH composite films. It can be clearly observed that only fracture silica tubes are observed owing to the strong interface adhesion by covalent bonding. Moreover, as manifested in Fig. 6, the silica tubes were uniformly distributed in the PAEK-COOH polymer matrix, the photograph of high magnification indicate the excellent compatibility between the two components, which is attributed to the strong interaction between the filler and polymer matrix by the covalent linkage via dehydration condensation reaction. This result would be favorable for the mechanical properties and dielectric properties of the composite films.
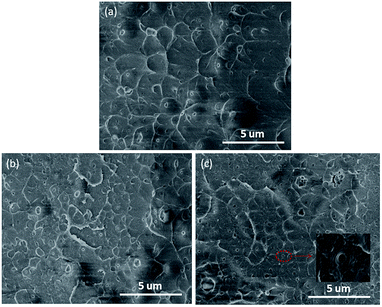 |
| Fig. 6 SEM photographs of (a) ST–PAEK-COOH-3 wt%; (b) ST–PAEK-COOH-5 wt%; (c) ST–PAEK-COOH-7 wt%. | |
Dielectric properties of PAEK-COOH and ST–PAEK-COOH composites
Fig. 7 presents the dielectric constants (κ) of PAEK-COOH polymer matrix and ST–PAEK-COOH composite films at the frequency range from 1000 Hz to 1 MHz. From Fig. 7, It can be seen that the dielectric constants of ST–PAEK-COOH composites (κ = 2.22–2.57 at 1 MHz) are distinctly lower than the pristine PAEK-COOH polymer matrix (κ = 2.92 at 1 MHz) under the same measurement conditions. Furthermore, the dielectric constants of the ST–PAEK-COOH composites decreased with increasing the contents of the silica tubes in composite films, and the lowest dielectric constant of 2.22 (at 1 MHz) can be achieved when the silica tubes content in the ST–PAEK-COOH composite films was 7 wt%. The increase of silica tubes in the composites signified the increase of air (the dielectric constant of air is about 1) to some extent in the composites which can greatly reduce the dielectric constants of the resulting porous structure composites.21–29,40 Besides, the presence of KH-550 and (3-trifluoromethyl) phenyl side groups into the polymer matrix decreased the dielectric constant due to an increase in free volume and a decrease in the polarizability.41–45 Hence, the introduction of silica tubes is a convenient and effective method to decrease the dielectric constant of composite films.
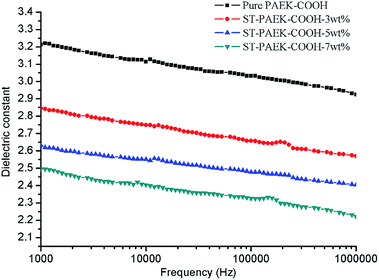 |
| Fig. 7 Frequency dependency of dielectric constant of pure PAEK-COOH and ST–PAEK-COOH composites. | |
Thermal properties of PAEK-COOH and ST–PAEK-COOH composites
The thermal stability of composites is vital to their applications in microelectronics. To examine the thermal durability of these polymer matrix and composites, PAEK-COOH and ST–PAEK-COOH composites were evaluated by thermogravimetric analysis (TGA) at a heating rate of 10 °C min−1 in nitrogen atmosphere as shown in Fig. 8 and Table 1. It was observed that the 10% decomposition temperatures of the composites are slightly lower than that of the PAEK-COOH polymer matrix, which might originate from the thermal degradation of the silane coupling agent covalently linked the polymer matrix and silica tubes in composites. Besides, the 10% decomposition temperatures of the composites increased with the increasing content of the silica tubes in the composite films, the improved thermal stabilities might be due to the transformation from aminopropyltriethoxysilane of the low thermal decomposition temperature in the side chain of PAEK-COOH polymer matrix to Si–O–Si of high thermal stability by incorporating silica tubes, as well as the high thermal stability of the inorganic silicon and oxygen framework of silica tubes. Overall, these results indicate that the excellent thermal properties of poly(aryl ether ketone)s were retained in the ST–PAEK-COOH composites.
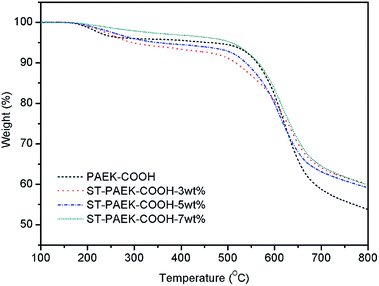 |
| Fig. 8 TGA analysis of PAEK-COOH with different contents of silica tubes. | |
Table 1 10% Decomposition of PAEK-COOH and ST–PAEK-COOH composites
Samples |
DT10a (°C) |
10% weight loss temperature measured by TGA at a heating rate of 10 °C min−1 in N2. |
Pure PAEK-COOH |
561 |
ST–PAEK-COOH-3 wt% |
517 |
ST–PAEK-COOH-5 wt% |
538 |
ST–PAEK-COOH-7 wt% |
562 |
Mechanical properties of PAEK-COOH and ST–PAEK-COOH composites
As is known to all that the mechanical properties of the composite films are determined by the nature of polymer matrix, the loading amount and dispersity of fillers, as well as the interfacial interaction between fillers and polymer matrix, etc. Well load transfer effectively between fillers and polymer matrix can enhance the tensile strength and modulus of the composite films owing to the reinforcement effect of fillers.46–49 Table 2 summarizes the data about the tensile strength, Young's modulus and elongation at break of the films casting from the DMAc solutions of PAEK-COOH and ST–PAEK-COOH composites. These films have a tensile strength of 61.8–93.0 MPa, Young's modulus of 1.74–2.12 GPa, and elongation at break of 11.4–61.2%. The elongation at break of ST–PAEK-COOH composite films are visibly lower than that of pristine PAEK-COOH polymer matrix and decreases with increasing the loading ratio of silica tubes, but the worst elongation at break of these composite films is still more than 10%. In addition, the tensile strength and the Young's modulus of these composite films increased compared with the pristine PAEK-COOH polymer matrix to some extent. The improvements in tensile strength of the ST–PAEK-COOH composite films was attributed to the fact that the load can be effectively transferred to the silica tubes to reinforce the PAEK-COOH matrix by the covalent bonding between the silica tubes and the PAEK-COOH polymer matrix with KH-550.50–53 Therefore, the well mechanical properties of these composites films can be achieved by the utilization of covalent linkage between the silica tubes and polymer matrix via KH-550 (Table 2).
Table 2 Mechanical properties of PAEK-COOH and ST–PAEK-COOH composite films
Samples |
Tensile strength (MPa) |
Young's modulus (GPa) |
Elongation at break (%) |
Pure PAEK-COOH |
61.8 ± 0.1 |
1.74 ± 0.06 |
61.2 ± 2.2 |
ST–PAEK-COOH-3 wt% |
73.9 ± 0.1 |
2.12 ± 0.11 |
27.6 ± 0.9 |
ST–PAEK-COOH-5 wt% |
93.0 ± 0.2 |
2.08 ± 0.09 |
20.2 ± 0.8 |
ST–PAEK-COOH-7 wt% |
79.4 ± 0.2 |
1.89 ± 0.09 |
11.4 ± 0.5 |
Conclusions
In summary, we have presented a new approach to prepared the porous composites with low dielectric constant, in which the silica tubes with uniform size have been synthesized successfully from the hydrolysis of tetraethyl orthosilicate and D,L-tartaric acid by a modified templating method, then a novel series of ST–PAEK-COOH composite films were prepared by the incorporation of γ-aminopropyltriethoxysilane with silica tubes into the PAEK-COOH polymer matrix. The prepared ST–PAEK-COOH composites exhibited low dielectric constants ranging from 2.22–2.57 at the frequency of 1 MHz. In addition, the excellent thermal and mechanical properties of PAEK-COOH polymer matrix were maintained. These results pave a new way for the preparation of porous composites with low dielectric constant and other functional materials.
Acknowledgements
This work was financially supported from the PhD Program Foundation of Ministry of Education of China (No. 20120061110017), Jilin Provincial Science and Technology Development Project of China (20150204001GX), Jilin Provincial Economic Structure Strategic Adjustment Guide Fund Special Project (High Technology Industry) (2015Y045).
Notes and references
- J. Kong, N. R. Franklin, C. Zhou, M. G. Chapline, S. Peng, K. Cho and H. Dai, Science, 2000, 287, 622 CrossRef CAS PubMed.
- C. Liu, Y. Y. Fan, M. Liu, H. T. Cong, H. M. Cheng and M. S. Dresselhaus, Science, 1999, 286, 1127 CrossRef CAS PubMed.
- P. M. Ajayan, Chem. Rev., 1999, 99, 1787 CrossRef CAS PubMed.
- S. S. Wong, E. Joselevich, A. T. Woolley, C. L. Cheung and C. M. Lieber, Nature, 1998, 394, 52 CrossRef CAS PubMed.
- H. Nakamura and Y. Matsui, J. Am. Chem. Soc., 1995, 117, 2651 CrossRef CAS.
- Z. L. Yang, Z. W. Niu, X. Y. Cao, Z. Z. Yang, Y. F. Lu, Z. B. Hu and C. C. Han, Angew. Chem., Int. Ed., 2003, 42, 4201 CrossRef CAS PubMed.
- M. E. Spahr, R. Stoschitizki, P. Bitterci and R. Nesper, Angew. Chem., Int. Ed., 1998, 37, 1263 CrossRef CAS.
- V. V. Ivanovskaya, A. N. Enyashin, A. A. Sofronov, Y. N. Makurin, N. I. Medvedeva and A. L. Ivanovskii, Solid State Commun., 2003, 126, 489 CrossRef CAS.
- H. H. Yin, K. Yu, H. Peng, Z. L. Zhang, R. Huang, J. T. Sejdic and Z. Q. Zhu, J. Mater. Chem., 2012, 22, 5013 RSC.
- Y. Feldman, E. Wasserman, D. J. Srolovitz and R. Tenne, Science, 1995, 267, 222 CrossRef CAS PubMed.
- Y. Y. Peng, Z. Y. Meng, C. Zhong, J. Lu, Z. P. Yang and Y. T. Qian, Mater. Chem. Phys., 2002, 73, 327 CrossRef CAS.
- A. Kis, D. Mihailovic, M. Remskar, A. Mrzel, A. Jesih, I. Piwonski, A. J. Kulik, W. Benoit and L. Forro, Adv. Mater., 2003, 15, 733 CrossRef CAS.
- M. Stefanov, A. N. Enyashin, T. Heine and G. Seifert, J. Phys. Chem. C, 2008, 112, 17764 CAS.
- S. F. Zhuo, Y. Xu, W. W. Zhao, J. Zhang and B. Zhang, Angew. Chem., Int. Ed., 2013, 125, 8764 CrossRef.
- B. Krause, K. Diekmann, N. F. A. van der Vegt and M. Wessling, Macromolecules, 2002, 35, 1738 CrossRef CAS.
- B. Krause, G. H. Koops, N. F. A. van der Vegt, M. Wessling, M. Wubbenhorst and J. van Turnhout, Adv. Mater., 2002, 14, 1041 CrossRef CAS.
- G. D. Fu, W. C. Wang, S. Li, E. T. Kang, K. G. Neoh, W. T. Tseng and D. J. Liaw, J. Mater. Chem., 2003, 13, 2150 RSC.
- Y. W. Chen, W. C. Wang, W. H. Yu, Z. L. Yuan, E. T. Kang, K. G. Neoh, B. Krauter and A. Greiner, Adv. Funct. Mater., 2004, 14, 471 CrossRef CAS.
- Y. W. Chen, W. C. Wang, W. H. Yu, E. T. Kang, K. G. Neoh, R. H. Vora, C. K. Ong and L. F. Chen, J. Mater. Chem., 2004, 14, 1406 RSC.
- W. C. Wang, R. H. Vora, E. T. Kang, K. G. Neoh, C. K. Ong and L. F. Chen, Adv. Mater., 2004, 16, 54 CrossRef CAS.
- G. D. Fu, Z. H. Shang, L. Hong, E. T. Kang and K. G. Neoh, Adv. Mater., 2005, 17, 2622 CrossRef CAS.
- J. J. Lin and X. D. Wang, Polymer, 2007, 48, 318 CrossRef CAS.
- G. F. Zhao, T. Ishizaka, H. Kasai, M. Hasegawa, T. Furukawa, H. Nakanishi and H. Oikawa, Chem. Mater., 2009, 21, 419 CrossRef CAS.
- Z. M. Dang, L. J. Ma, J. W. Zha, S. H. Yao, D. Xie, Q. Chen and X. Duan, J. Appl. Phys., 2009, 105, 044104 CrossRef.
- X. Y. Zhao and H. J. Liu, Polym. Int., 2010, 59, 597 CAS.
- K. Taki, K. Hosokawa, S. Takagi, H. Mabuchi and M. Ohshima, Macromolecules, 2013, 46, 2275 CrossRef CAS.
- Y. K. Zou, Y. Q. Zhan, R. Zhao and X. B. Liu, J. Mater. Sci.: Mater. Electron., 2013, 24, 1238 CrossRef CAS.
- X. Y. Huang, C. Y. Zhi, P. K. Jiang, D. Golberg, Y. Bando and T. Tanaka, Adv. Funct. Mater., 2013, 23, 1824 CrossRef CAS.
- M. K. Kim, D. W. Kim, D. W. Shin, S. J. Seo, H. K. Chung and J. B. Yoo, Phys. Chem. Chem. Phys., 2015, 17, 2416 RSC.
- T. E. Attwood, P. C. Dawson, J. L. Freeman, L. R. J. Hoy, J. B. Rose and P. A. Staniland, Polymer, 1981, 22, 1096 CrossRef CAS.
- P. M. Hergenrother, B. J. Jensen and S. J. Havens, Polymer, 1988, 29, 358 CrossRef CAS.
- J. B. Rose, Polymer, 1974, 15, 456 CrossRef CAS.
- A. Jonas and R. Legras, Polymer, 1991, 32, 2691 CrossRef CAS.
- T. Fukumaru, T. Fujigaya and N. Nakashima, Polym. Chem., 2012, 3, 369 RSC.
- K. Taki, K. Hosokawa, S. Takagi, H. Mabuchi and M. Ohshima, Macromolecules, 2013, 46, 2275 CrossRef CAS.
- X. Y. Huang, C. Y. Zhi, P. K. Jiang, D. Golberg, Y. Bando and T. Tanka, Adv. Funct. Mater., 2013, 23, 1824 CrossRef CAS.
- G. B. Wang, C. H. Chen, H. W. Zhou, Z. H. Jiang, W. J. Zhang and Z. W. Wu, Chem. Res. Chin. Univ., 2000, 21, 1325 CAS.
- F. Miyaji, Y. Watanabe and Y. Suyama, Mater. Res. Bull., 2003, 38, 1669 CrossRef CAS.
- F. Miyaji, S. A. Davis, J. P. H. Charmant and S. Mann, Chem. Mater., 1999, 11, 3021 CrossRef CAS.
- K. Taki, K. Hosokawa, S. Takagi, H. Mabuchi and M. Ohshima, Macromolecules, 2013, 46, 2275 CrossRef CAS.
- C. Yuan, K. Jin, K. Li, S. Diao, J. Tong and Q. Fang, Adv. Mater., 2013, 25, 4875 CrossRef CAS PubMed.
- L. Kong, Y. Cheng, Y. Jin, Z. Ren, Y. Li and F. Xiao, J. Mater. Chem. C, 2015, 3, 3364 RSC.
- M. G. Dhara and S. Banerjee, Prog. Polym. Sci., 2010, 35, 1022 CrossRef CAS.
- J. Wang, K. Jin, F. He, J. Sun and Q. Fang, RSC Adv., 2014, 4, 40782 RSC.
- Y. T. Chern and H. C. Shiue, Macromolecules, 1997, 30, 4646 CrossRef CAS.
- L. Zheng, R. J. Farris and E. B. Coughlin, Macromolecules, 2001, 34, 8034 CrossRef CAS.
- H. Y. Xu, S. W. Kuo, J. S. Lee and F. C. Chang, Macromolecules, 2002, 35, 8788 CrossRef CAS.
- J. Choi, J. Harcup, A. F. Yee, Q. Zhu and R. M. Laine, J. Am. Chem. Soc., 2001, 123, 11420 CrossRef CAS PubMed.
- Y. Z. Pan, Y. Xu, L. An, H. B. Lu, Y. L. Yang, W. Chen and S. Nutt, Macromolecules, 2008, 41, 9245 CrossRef CAS.
- S. Y. Fu, X. Hu and C. Y. Yue, J. Mater. Sci., 1998, 33, 4953 CrossRef CAS.
- S. Y. Fu and B. Lauke, Compos. Sci. Technol., 1998, 58, 1961 CrossRef CAS.
- B. Lauke and S. Y. Fu, Compos. Sci. Technol., 1999, 59, 699 CrossRef.
- S. Y. Fu, C. Y. Yue, X. Hu and Y. W. Mai, Compos. Sci. Technol., 2000, 60, 569 CrossRef.
|
This journal is © The Royal Society of Chemistry 2016 |