DOI:
10.1039/C6RA13167K
(Paper)
RSC Adv., 2016,
6, 84523-84535
Punica granatum (pomegranate) peel extract exerts potent antitumor and anti-metastasis activity in thyroid cancer
Received
20th May 2016
, Accepted 22nd August 2016
First published on 26th August 2016
Abstract
The incidence of thyroid carcinoma has obviously been rising throughout the world during the past ten years. However, over-treatment has usually occurred in thyroid carcinoma without new and effective approaches being explored. In this study, Punica granatum (pomegranate) peel extract (PoPx), a kind of herb, was evaluated for its anticancer activity to thyroid carcinoma in vitro and in vivo. PoPx potently suppressed proliferation in two kinds of thyroid cancer cell lines, and induced cancer cell apoptosis. PoPx could also decrease the mitochondrial membrane potential (ΔΨm), indicating that PoPx may induce apoptosis via a mitochondria-mediated apoptotic pathway. In addition, PoPx markedly impaired thyroid cancer cell migration and invasion by down-regulating the expression of matrix metalloproteinase-9 (MMP-9). More importantly, PoPx significantly inhibited tumor growth in the BCPAP-bearing mice model by reducing cell proliferation and inducing apoptosis. These findings suggest that PoPx could be an effective phytochemical agent.
Introduction
As one of the most common endocrine malignancies, the increasing incidence of thyroid cancer in the world has drawn public attention.1 About 95% of thyroid cancer derives from the thyroid follicular epithelial cells, including papillary thyroid carcinoma (PTC, 80%), follicular thyroid carcinoma (FTC, 10–15%), poorly differentiated thyroid cancer (PDTC) and anaplastic thyroid cancer (ATC, 1–2%).3 The survival rate of thyroid cancer is relatively higher than for any other malignant tumors, especially PTC. However, recurrence and mortality is still a challenge for thyroid cancer treatment. Traditional treatment of thyroid cancer includes surgical resection, postoperative radio therapy, thyroid stimulating hormone (TSH) suppression treatment and small molecule multi-targeted kinase inhibitors (MKI), which are emerging as a new choice for thyroid cancer.4 As the major treatment for thyroid cancer, surgery affects the patient's quality of life, with many postoperative complications. Postoperative radioactive iodine treatment can destroy a residual thyroid tumor or distant metastasis and obliterate the residual thyroid tumor tissue, but its curative effect depends on the uptake rate of I131, which limits its application. It is beneficial for patients to keep a low level of TSH after surgery to reduce cancer recurrence and prolong life, but subclinical hyperthyroidism caused by long-term TSH suppression also has side-effects on the cardiovascular system, skeletal system and other aspects.5,6 However, the efficacy and safety of targeted drugs remain to be confirmed by further research. Therefore, it is necessary to find a new way to prevent and treat thyroid cancer.7
Traditional Chinese Medicine (TCM) has precious value in disease prevention and health care.8–12 In recent years, the pomegranate as a natural medicine and health food has been widely used all over the world. Continuous research about the bioactivity of pomegranate has gradually drawn the attention of scholars.13–15 It was reported that pomegranate peel extract (PoPx) has various bioactivities in regulating blood glucose level,16 oxidation resistance,17 anti-bacteria,18 etc.19,20 Recently, some studies have shown that PoPx inhibits tumor growth in prostate cancer,21 colon cancer,22 skin cancer23 and breast cancer24 by inducing cell apoptosis.
However, the effect of PoPx on thyroid cancer has not been reported yet, and further research is needed to illustrate the mechanism of PoPx. To provide evidence for the wide application of PoPx in thyroid cancer prevention and treatment, the effect of PoPx was assessed on BCPAP and TPC-1 papillary thyroid carcinoma cells and the preliminary molecular mechanism was explored in our study.
Materials and methods
Preparation of PoPx
Pomegranate peel of Tunisia soft-seed pomegranate was obtained from Yuzhuang Ecological Green Industry Co. (Qianxi County, Guizhou Province, China). The preparation of pomegranate peel extract referred to previously described methods with minor modification.25 The fresh peel (400 g) was cut into pieces of 0.5 cm in size and extracted with a combination of ethanol (1550 mL) and H2O (480 mL), following soaking for 2 h at 60 °C. Then the crude extracts were filtered and concentrated under vacuum; 45 g brown powder solid was finally collected. The PoPx was stored at −20 °C and protected from light before use.
Reagents and antibodies
3-(4,5-Dimethylthiazol-2-yl)-2,5-diphenyltetrazoliumbromide (MTT), dimethyl sulfoxide (DMSO), 2-(6-amino-3-imino-3H-xanthen-9-yl)benzoic acid methyl ester (Rh123) and 2′,7′-dichlorodihydrofluorescein diacetate (DCFH-DA) were purchased from Sigma Chemical Co. (St Louis, MO, U.S.A.). Hoechst 33258 was purchased from Beyotime (Beijing, China). An Annexin V-FITC Apoptosis Detection Kit was purchased from KeyGen Biotech (Nanjing, China). For western blot experiments, the primary antibodies against matrix metalloproteinase-9 (MMP-9), Bax and β-actin were purchased from Cell Signaling Technology (Beverly, MA, U.S.A.) and secondary antibodies were purchased from ZSGB-BIO Co. (Beijing, China). For immunohistochemistry studies, the primary antibodies against cleaved caspase-3 (CC-3) and matrix metalloproteinase-9 (MMP-9) were purchased from Cell Signaling Technology (Beverly, MA, U.S.A.). Mouse monoclonal anti Ki-67 was purchased from Merck-Millipore. The DAB Detection Kit was from ZSGB-BIO Co. (Beijing, China).
For in vitro assays, PoPx was prepared as a 200 mg mL−1 stock solution in DMSO and stored at −20 °C. Then the stock solution was diluted in the relevant assay medium, and 0.1% DMSO served as a vehicle control. For in vivo studies, PoPx was dissolved in normal saline and dosed at 125 mg kg−1 and 62.5 mg kg−1 of body weight.
High performance liquid chromatography (HPLC) and mass spectrography (MS)
A Waters 2695 HPLC system (Waters Corp., Milford, Massachusetts, U.S.A.) was used in this experiment. The method and chromatographic conditions referred to previously described methods.26 Data were processed by Waters Empower 3 software. The chromatographic separation was conducted on a Waters Symmetry C18 column (5 μm, 4.6 × 250 mm). The mobile phase consisted of deionized water with glacial acetic acid (A; 99
:
1, v/v; pH 3.0) and methanol (B) with a flow rate of 1 mL min−1. The gradient program was set as follows: 0–70 min, 10–45% B; 70–80 min, 45% B. The chromatogram was detected at a wavelength of 256 nm throughout the assay. An Absciex Qtrap 5500 was used in the MS. We detected the samples by HPLC and MS after establishing the standard working curves of ellagic acid (EA) and punicalagin (PC).
Cell culture
The human papillary thyroid cancer cell lines BCPAP (harboring BRAF V600E mutation) were obtained from the University of Medicine Lyon-Sud and TPC-1 (harboring RET-PTC rearrangement) from the Institute of Interdisciplinary Research. Nthy-ori 3-1 (human thyroid follicular epithelial cell line) was obtained from the Health Protection Agency Culture Collection. All the other human normal cell lines used in our study were obtained from the American Type Culture Collection. Cells were propagated in RPIM 1640 or DMEM medium containing 10% heat-inactivated fetal bovine serum (FBS) and 1% antibiotics (penicillin and streptomycin) in 5% CO2 at 37 °C.
Cell viability assay
The cell viability of PoPx-treated thyroid cancer cells was assessed by MTT assay. Briefly, the exponentially growing cells (2–6 × 103 cells per well) were plated in 96-well plates (100 μL per well) and incubated for 24 h. Then the cells were treated with different concentrations of PoPx (0, 12.5, 25, 50, 100, 200 μg mL−1). After treatment for 24 h, 48 h and 72 h, respectively, 20 μL of 5 mg mL−1 MTT was added to each well, and the plates were incubated at 37 °C for an additional 2–4 h. The medium was subsequently removed, and the purple-colored precipitates of formazan were dissolved in 150 μL of DMSO. The color absorbance was recorded at 492 nm using a Spectra MAX M5 microplate spectrophotometer (Molecular Devices, CA, U.S.A.). In addition, a 72 h MTT assay was conducted on HEK293 (human embryonic kidney cell line) and LO2 (human normal liver cell line). Meanwhile, 24 h, 48 h and 72 h MTT assays were also performed on Nthy-ori 3-1 (human thyroid follicular epithelial cell line) to test the cytotoxicity of PoPx. The data shown represent the average of three independent experiments.
Colony formation assay
Colony formation assay was measured as previously described.27 Briefly, BCPAP and TPC-1 cells were seeded with a specified number of cells (2000 cells per well) in 6-well plates. After 24 h incubation, the cells were treated with designated concentrations of PoPx (0, 12.5, 25, 50, 100, 200 μg mL−1) and then incubated for an additional 10 days. Then the cells were fixed with methanol and stained with a 0.5% crystal violet solution for about 15 minutes and the colonies (>50 cells) were counted under a microscope. The data shown represent the average of three independent experiments.
Morphological analysis of nuclei by Hoechst staining
An apoptotic cell has distinct morphologic characteristics: cell body shrinkage, chromatin condensation and margination as well as emerging apoptotic bodies.28 To identify whether the PoPx-inducing reduction in cell proliferation was attributable to apoptosis, we stained the BCPAP and TPC-1 cells with Hoechst 33258 dye. In brief, BCPAP and TPC-1 cells (1–2 × 105 cells per well) were plated onto an 18 mm coverglass in a 6-well plate for 24 h each. After treatment with different concentrations (0, 12.5, 25, 50, 100, 200 μg mL−1) of PoPx for the following 48 h, the cells were washed with ice-cold phosphate-buffered saline (PBS) twice and fixed in methanol for 15 minutes. The cells were stained with the Hoechst 33258 solution in accordance with the manufacturer's instructions. Then the nuclear morphology of the apoptotic cells was photographed under a fluorescence microscope (Leica, DM4000B).
Apoptosis analysis by flow cytometry (FCM)
To further confirm the apoptosis induction by PoPx, an Annexin V-FITC apoptosis detection kit was used as described before.29 In short, cells (1–2 × 105 cells per well) were seeded in 6-well plates and treated with PoPx (0, 12.5, 25, 50, 100, 200 μg mL−1) for 48 h. Then the cells were harvested and washed twice with cold PBS. The level of apoptosis was detected by FCM using the apoptosis detection kit and referring to the manufacturer's instructions. The data were analyzed with FlowJo software. The data shown represent the average of three independent experiments.
Detection of mitochondrial membrane potential (Ψm) and reactive oxygen species (ROS)
A mitochondrial membrane potential assay was performed as previously reported, and examined by FCM using Rh123 staining.30 Cells were treated with indicated doses of PoPx (0, 12.5, 25, 50, 100, 200 μg mL−1) for 48 h and then incubated with 10 μM Rh123 at 37 °C in the dark for approximately 30 min. The stained cells were then washed with cold PBS twice, and then Rh123 fluorescence was detected by FCM. For the intracellular ROS detection,31 cells were treated with purposed concentrations of PoPx (0, 12.5, 25, 50, 100, 200 μg mL−1) for 48 h and then incubated with 10 μM DCFH-DA diluted in PBS at 37 °C for 30 min. The stained cells were then washed with cold PBS and analyzed by FCM. The data shown represent the average of three independent experiments.
Boyden chamber migration and invasion assay
A Boyden chamber (8 μm pore size) migration assay was conducted as previously described, with a few modifications.32 Briefly, a total of 1.5 × 105 cells (for BCPAP) or 1 × 105 cells (for TPC-1) in 100 μL serum-free medium were added into the top chamber of 24-well transwell plates (Millipore), and a 600 μL medium containing 10% FBS was added at the bottom. Different concentrations of PoPx (0, 25, 100, 200 μg mL−1) were added into both chambers. Cells were allowed to migrate for approximately 48 h and non-migrated cells in the top chamber were discarded. Then the migrated cells adhering to the lower surface of the transwell membrane were fixed in methanol and stained with 0.5% crystal violet. Migrated cells in 3 fields selected randomly were counted and photographed under a light microscope. An invasion assay was determined according to previous studies.33 In brief, the upper surface of the transwell membrane was covered with Matrigel (1
:
3, 60 μL per well, BD Biosciences, U.S.A.) diluted by serum-free medium and the lower compartment of the chamber was filled with 600 μL medium containing 10% FBS. 1.5 × 105 cells (for BCPAP) or 1 × 105 cells (for TPC-1) in 100 μL serum-free medium were placed in the upper part of each transwell and treated with designated concentrations of PoPx (0, 25, 100, 200 μg mL−1). After incubation for 48 h, cells located on the upper side of the filter were removed and those on the underside were fixed with methanol and stained with 0.5% crystal violet. Then, similarly, migrated cells were counted and photographed under a light microscope. The results were expressed as the percentage inhibition rate of migration compared with the vehicle group.
Western blot analysis
To determine the effect of PoPx on the signaling pathway involved, some proteins in BCPAP cells were evaluated by western blot. The western blot analysis was performed as described previously,34 with minor modification. Briefly, BCPAP cells were treated with PoPx in different concentrations (0, 25, 100, 200 μg mL−1) for 48 h; then cells were washed twice with cold PBS and lysed in RIPA buffer. After the cell lysates had been centrifuged at 13300 rpm at 4 °C for 15 min, the supernatant was harvested. The extractive protein concentrations were measured using the Coomassie brilliant blue G-250 method and equalized before loading. Equal amounts of protein from each sample were subjected to sodium dodecyl sulfate-polyacrylamide gel electrophoresis (SDS-PAGE) gels, following transfer onto polyvinylidene difluoride (PVDF) membranes (Amersham Bioscience, Piscataway, N.J.). Then, the membranes were blocked with 5% skimmed milk at 37 °C for 2 h and incubated with peculiar primary antibodies overnight at 4 °C. After incubation with the relevant secondary antibodies, the reactive bands were identified using an enhanced chemiluminescence kit (Amersham Bioscience, Piscataway, N.J.).
Xenograft tumor assay in nude mice
All animal experiments have been approved by the Institutional Animal Care and Treatment Committee of Sichuan University in China (Permit number: 20151109-2) and were carried out in accordance with the approved guidelines. Female BALB/c nude mice (5–6 weeks old) were obtained from Sichuan University Laboratory Animal Center (Chengdu, China). After the female BALB/c athymic mice had adapted to the environment for a week, they were randomized into three groups (8 mice per group), and received intragastric administration (i.g.) of PoPx 125 mg kg−1, 62.5 mg kg−1 or vehicle, respectively, once daily for 6 days. Then we inoculated the mice with BCPAP cells (2.0 × 106 cells per 120 μL per each). After subcutaneous ectopic cell implantation, treatment was discontinued for 7 days and then continued for 18 days. Tumor volume and body weight were measured every three days. The tumor size was calculated according to the formula: tumor volume (mm3) = 0.52 × L × W2 where L is the length and W is the width. At the end of the experiment (on the 31st day), all animals were euthanized by cervical dislocation. Tumors and internal organs, such as hearts, livers, spleens, lungs and kidneys, were excised from the animals.
These tissues (tumors and internal organs) of the mice were fixed in formalin, embedded in paraffin, cut into 4 μm sections, dried, and kept at room temperature. Immunohistochemistry (IHC) staining was as described previously.35 Tumor sections were subjected to antigen retrieval, blocked with serum, incubated with primary antibodies (CC-3, Ki-67 and MMP-9), then stained with the DAB Detection Kit. Moreover, to evaluate the effect of PoPx on visceral organ damage to the animals, we performed hematoxylin and eosin (H&E) staining of tissue sections (heart, liver, spleen, lung and kidney). Images were taken with a Leica microscope (Leica, DM4000B).
Statistical analysis
Cell culture-based experiments were done in at least biological triplicates. Quantifications of staining were done on sections in at least three different views. Data were represented as means ± SD. The 2-tailed Student's t test was used for statistical analysis and statistically significant p values were labeled as follows: *p < 0.05; **p < 0.01; ***p < 0.001.
Results
The content and molecular weight of punicalagin and ellagic acid in PoPx
Linear regression analysis for punicalagin (PC) and ellagic acid (EA) was expressed by plotting the peak area (y) against the concentrations (x, μg mL−1) of standard solutions, as shown in Table 1. All the analytes presented high linearity in the investigated ranges. The sample of PoPx was analyzed using the optimized HPLC method and matched by the Similarity Evaluation System for Chromatographic Fingerprint of Traditional Chinese Medicine (Version 2012A). Components were identified as PC (peak 1 and peak 2) and EA (peak 3) by comparing with the retention time of standard substances, which is shown in Fig. 1A, and the chemical structure is shown in Fig. 1B. As shown in Fig. 1C, the molecular weight of EA (ellagic acid [M − H]−: 301.3) and PC (punicalagin [M − H]−: 1083.2) was detected from a mass spectrum. The content of PC and EA in PoPx was calculated according to the regression equation and the relevant peak area of PoPx. The results of the analysis reveal that PC accounts for 479.82 mg g−1 and EA accounts for 7.53 mg g−1 in PoPx.
Table 1 Linear range, regression equation and R2 of EA and PC
Component |
Linear range (mg L−1) |
Regression equation |
R2 |
y is the peak area; x refers to the concentration of compound (mg L−1). |
EA |
50–500 |
ya = 149 530x + 2 × 106 |
0.9935 |
PC |
125–2000 |
ya = 2 × 107x − 6 × 106 |
0.971 |
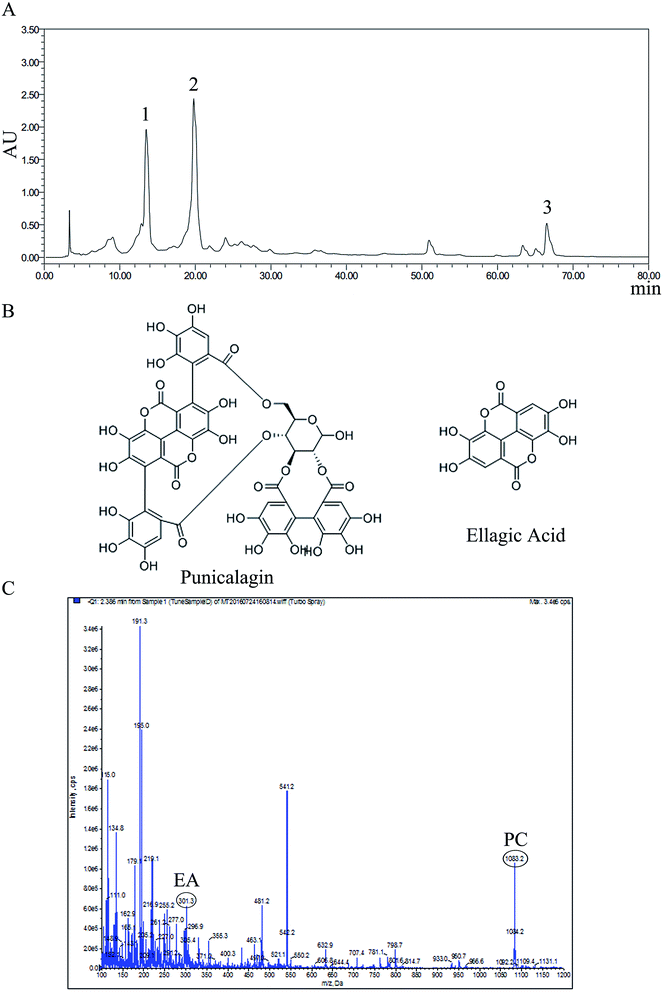 |
| Fig. 1 HPLC and MS. (A) Chromatogram of PoPx, PC (peak 1 and peak 2) and EA (peak 3). (B) The chemical structure of PC and EA. (C) The molecular weight of EA (ellagic acid [M − H]−: 301.3) and PC (punicalagin [M − H]−: 1083.2). | |
PoPx inhibits proliferation and influences the morphology of thyroid cancer cells
To evaluate the effect of PoPx on thyroid cancer, BCPAP and TPC-1 cell lines were used in this study. As shown in Fig. 2B, we investigated the time- and concentration-dependent effect of PoPx on thyroid cancer cells by exposing cells to various concentrations of PoPx (0, 12.5, 25, 50, 100 and 200 μg mL−1) for 24 h, 48 h and 72 h. TPC-1 proliferation increased with 12.5–50 μg mL−1 PoPx treatment for 24 h. However, 100–200 μg mL−1 PoPx treatment obviously inhibited the proliferation of TPC-1. Meanwhile, cell viability decreased with 25–200 μg mL−1 PoPx treatment for 48 h and 12.5–200 μg mL−1 PoPx treatment for 72 h. Moreover, low concentrations of PoPx treatment for 24 h also did not inhibit the proliferation of BCPAP and only in a concentration of 200 μg mL−1 of PoPx was there distinctly reduced proliferation. Furthermore, cell viability decreased with 50–200 μg mL−1 PoPx treatment for 48 h and 12.5–200 μg mL−1 PoPx treatment for 72 h in BCPAP. Therefore, we deduced that the anti-tumor effect of PoPx is not stable at lower doses in 24 hours. Concentration and drug treatment time both affect the effectiveness of anti-proliferation in thyroid cancer cells. After treatment with PoPx for 48 h and 72 h, respectively, the proliferation of both BCPAP and TPC-1 cell lines decreased obviously with IC50 values lessened from 110.8 μg mL−1 to 76.9 μg mL−1 for BCPAP and from 120.8 μg mL−1 to 68.4 μg mL−1 for TPC-1. Moreover, we examined the inhibitory effect of PoPx on three normal cell lines (Nthy-ori 3-1, HEK293 and LO2), while the IC50 values were more than 400 μg mL−1 (Table 2). In addition, a change in the morphology of the thyroid cancer cells was observed after 48 h of treatment with PoPx (Fig. 2A).
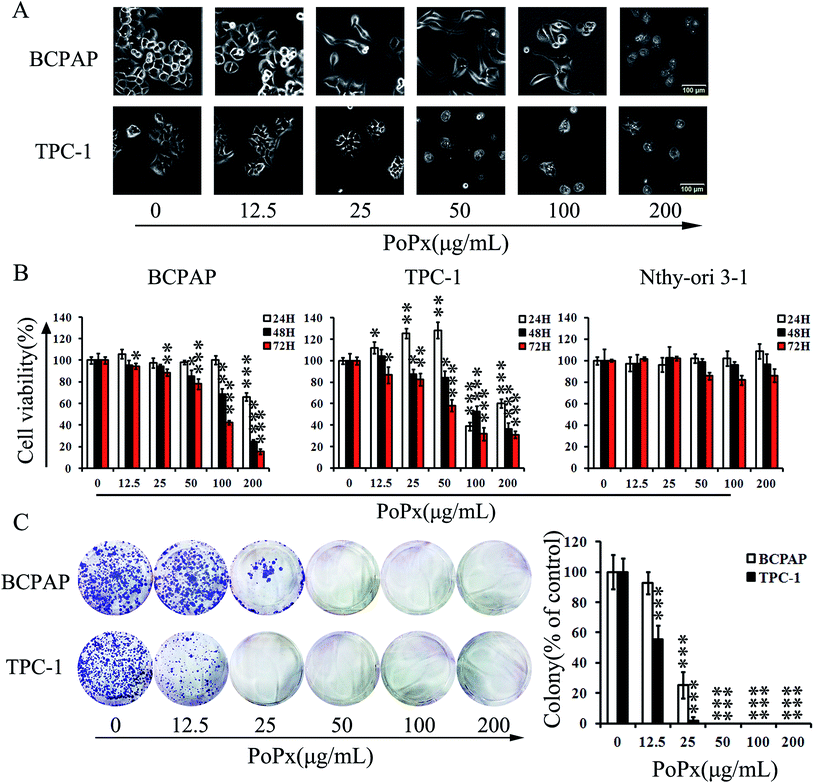 |
| Fig. 2 Inhibition of cell growth and colony formation on human cancer cell lines BCPAP and TPC-1 by PoPx. (A) Cells were exposed to various doses of PoPx (0–200 μg mL−1) for 48 h and cell morphologic changes were assessed (×10). (B) Proliferation of BCPAP, TPC-1 and Nthy-ori 3-1 cells treated with various concentrations (0–200 μg mL−1) of PoPx for 24, 48 and 72 h, respectively. Cell viability was evaluated by MTT assay. Data are expressed as means ± SD from three experiments (*p < 0.05; **p < 0.01; ***p < 0.001 vs. vehicle control). (C) The effect of PoPx (0–200 μg mL−1) on colony formation in BCPAP and TPC-1 cell lines for 10 days, the statistic results of colony formation assays presented as surviving colonies. Data are expressed as means ± SD from three experiments (*p < 0.05; **p < 0.01; ***p < 0.001 vs. vehicle control). | |
Table 2 The effect of PoPx on normal cell line viabilitya
Cell lines |
Cell type |
IC50 (μg mL−1) |
Each cell line was treated with various concentrations (0, 12.5, 25, 50, 100, 200 μg mL−1) of PoPx for 72 h. MTT assay was used to determine the IC50 values. Data are expressed as means ± SD for three independent experiments. |
Nthy-ori 3-1 |
Human thyroid follicular epithelial cell line |
>400 |
HEK293 |
Human embryonic kidney cell line |
>400 |
LO2 |
Human normal liver cell line |
>400 |
To further investigate whether PoPx could inhibit the proliferation of thyroid cancer cells, we performed a colony formation assay after PoPx treatment. Fig. 2C clearly shows that clone formation of BCPAP and TPC-1 cells was reduced in a concentration-dependent manner after exposure to PoPx. Moreover, the number of colonies treated with PoPx significantly reduced compared with the control. Taken together, these results implied that PoPx has strong a cytostatic and cytotoxic effect on thyroid cancer cells.
PoPx induced cell apoptosis in BCPAP and TPC-1 cell lines
We next explored whether PoPx induced thyroid cancer cells apoptosis. Hoechst 33258 staining assay showed that PoPx treatment resulted in cell shrinking, nuclear fragmentation and formation of condensed nuclei with bright-blue fluorescence. Also, these changes were concentration-dependent (Fig. 3A). The morphological changes were also observed in TPC-1 cells (Fig. 3A).
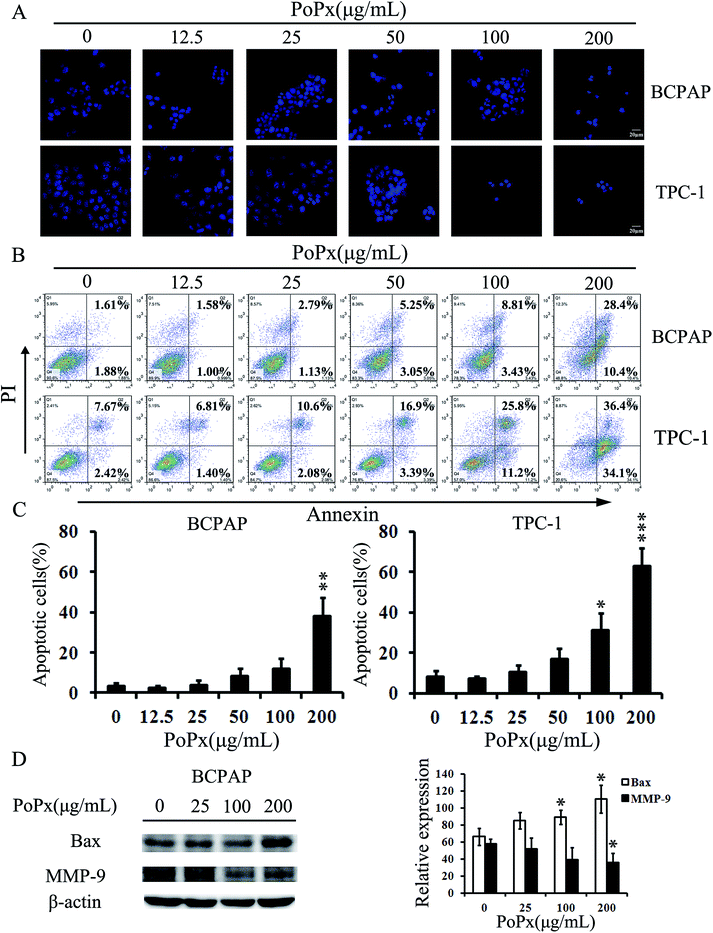 |
| Fig. 3 PoPx induces thyriod cancer cells apoptosis. (A) Cell nuclear changes of BCPAP and TPC-1 cells were determined by staining with Hoechst 33258 and visualized by a fluorescence microscope after treatment with increasing doses of PoPx for 48 h (×40). (B) BCPAP and TPC-1 cells were treated with PoPx at indicated doses for 48 h, and the level of apoptosis was evaluated using the Annexin V/PI dual-labeling technique, and determined by FCM. (C) The proportions of apoptotic cells, including the early apoptotic cells (LR, positive for Annexin V only) and the late apoptotic cells (UR, Annexin V and PI-positive), were analysed after various concentrations (0–200 μg mL−1) of PoPx treatment for 48 h. Data are expressed as means ± SD from three experiments (*p < 0.05; **p < 0.01; ***p < 0.001 vs. vehicle control). (D) Western blot analyses of BCPAP cells treated (48 h) with different concentrations (0, 25, 100 and 200 μg mL−1) of PoPx were used to evaluate protein expression of Bax and MMP-9. Protein expression was quantified by the densitometry analysis using Image J and normalized against β-actin expression. Statistics of the relative expression of Bax increased and MMP-9 decreased after treatment with PoPx are shown on the right. Data are expressed as means ± SD from three experiments (*p < 0.05; **p < 0.01; ***p < 0.001 vs. vehicle control). | |
To further confirm the induction of apoptosis in BCPAP and TPC-1 cells with PoPx treatment, we also investigated the levels of apoptosis using the Annexin V-FITC/PI dual-labeling technique by flow cytometry (FCM). As shown in Fig. 3B and C, PoPx induced BCPAP cell apoptosis in a concentration-dependent manner. After 48 h, the percentage of apoptotic cells (the early apoptotic cells plus the late apoptotic cells) increased from 3.49% to 38.8% as the concentration increased from 0 to 200 μg mL−1, indicating a change of nearly 35.3%. Similar results were observed in TPC-1 cells and the percentages of apoptotic cells at different concentrations are as follows: vehicle (10.09%), 12.5 μg mL−1 (8.21%), 25 μg mL−1 (12.68%), 50 μg mL−1 (20.29%), 100 μg mL−1 (37.00%) and 200 μg mL−1 (70.50%). In addition, western blotting also confirmed the characterization of PoPx-induced apoptosis. We examined Bax expression levels in BCPAP cells after PoPx-treatment for 48 h. As shown in Fig. 3D, the expression of Bax (a kind of pro-apoptotic protein) increased in a concentration-dependent manner, suggesting that PoPx could induce the apoptosis of thyroid cancer cells.
PoPx induced loss of mitochondrial transmembrane potential (ΔΨm) and ROS generation
In the previous data, an increased level of Bax was observed after treatment with PoPx (Fig. 3D). We speculated that PoPx-induced apoptosis might be via the mitochondrial apoptotic pathway. In order to verify the hypothesis, we tested the changes in the mitochondrial membrane potential (ΔΨm) by FCM using a green fluorochrome Rh123. As shown in Fig. 4A, B and C, PoPx treatment led to the loss of ΔΨm in BCPAP and TPC-1 cells. Especially in the dose of 200 μg mL−1, the loss of ΔΨm in BCPAP increased significantly: to 56.13% compared with vehicle group after exposure to PoPx for 48 h. Similar results were observed in TPC-1 cells. These results suggested that the mitochondria-mediated pathway may be involved in PoPx-induced apoptosis.
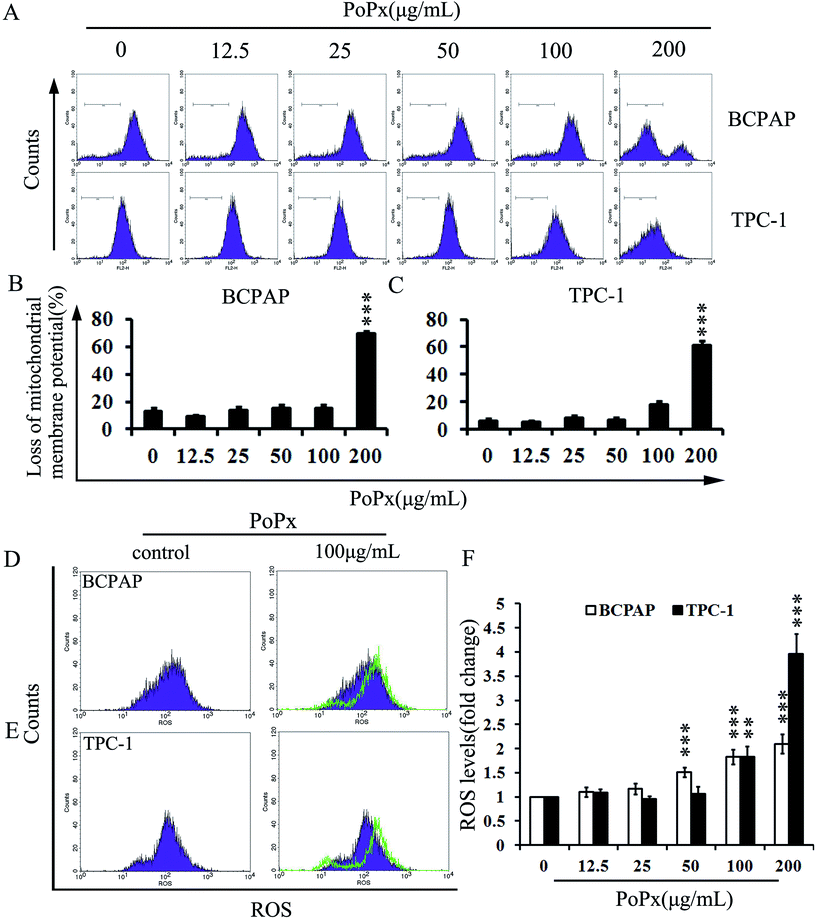 |
| Fig. 4 Effects of PoPx via the intrinsic apoptosis pathway. (A–C) PoPx reduced the mitochondrial membrane potential (ΔΨm) in BCPAP and TPC-1 cells. BCPAP and TPC-1 cells were treated with various concentrations (0–200 μg mL−1) of PoPx for 48 h and then stained with 10 μM Rh123 to detect the change in ΔΨm by FCM. Data are expressed as means ± SD from three experiments (*p < 0.05; **p < 0.01; ***p < 0.001 vs. vehicle control). BCPAP (D) and TPC-1 (E) cells were treated with designated concentrations (0–200 μg mL−1) of PoPx for 48 h. The harvested cells were loaded with 10 μM DCFH-DA and then ROS levels in the cells were measured by FCM. (F) Quantification of ROS is shown. Data are expressed as means ± SD from three experiments (*p < 0.05; **p < 0.01; ***p < 0.001 vs. vehicle control). | |
Much research has shown that mitochondria play central roles in cellular metabolism and apoptosis and are a major source of reactive oxygen species (ROS).36,37 Excessive ROS induces mitochondrial permeability transition (PT) pore opening and cytochrome c release from mitochondria, which results in a drop in Δψm, apoptosome formation and caspase activation.38 In order to test the levels of ROS, we stained the cells with DCFH-DA reagent and estimated ROS accumulation by FCM. The results showed that the levels of ROS increased in a dose-dependent manner. The changes in ROS level increased up to 2.1 times in BCPAP cells and up to 4.0 times in TPC-1 cells when 200 μg mL−1 PoPx was administered (Fig. 4D–F). These results confirmed that the inhibition of thyroid cancer cells by PoPx is mediated by the induction of apoptosis via the mitochondria-mediated apoptotic pathway.
PoPx inhibits thyroid cancer cell migration and invasion
We performed a transwell migration assay using BCPAP and TPC-1 cell lines. As shown in Fig. 5A, PoPx inhibits migration of both BCPAP and TPC-1 cells in a dose-dependent manner. Then, we performed a Matrigel invasion assay. The invasion ability of both BCPAP and TPC-1 cells significantly decreased in the presence of PoPx over that of the vehicle, which can be explicitly observed in Fig. 5B.
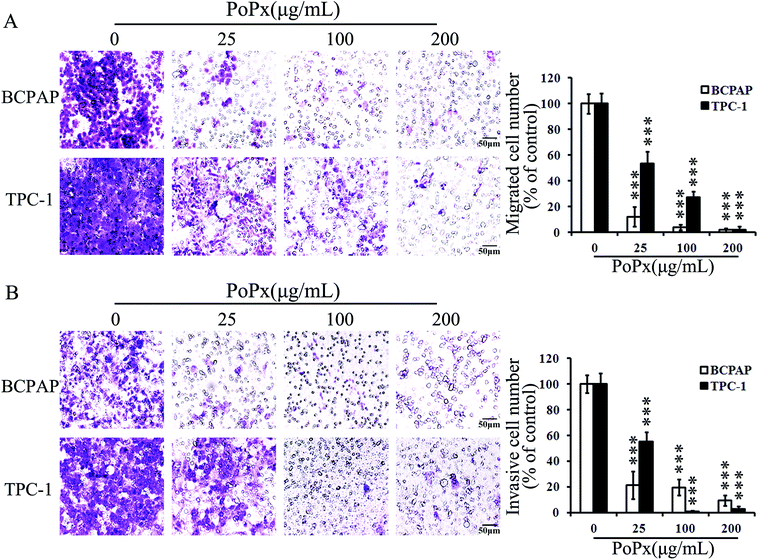 |
| Fig. 5 PoPx inhibits thyriod cancer cells BCPAP and TPC-1 migration and invasion. (A) PoPx inhibits BCPAP and TPC-1 migration. Tumor cells were seeded in the top chamber of the transwell with a serum-free medium and treated with vehicle or designated concentrations (25, 100, 200 μg mL−1) of PoPx. After about 48 h, the migrated cells were fixed, stained, photographed (×20) and quantified (*p < 0.05, **p < 0.01, ***p < 0.001). (B) PoPx inhibits BCPAP and TPC-1 invasion. Tumor cells were planted in which were pre-treated with Matrigel on the upper chamber membrane and treated with various concentrations (0, 25, 100, 200 μg mL−1) of PoPx, and the bottom chamber was filled with the complete medium containing 10% FBS. After about 48 h, invaded cells were fixed, stained, photographed (×20) and counted (*p < 0.05, **p < 0.01, ***p < 0.001). | |
Furthermore, considering the relationship between matrix metalloproteinase-9 (MMP-9) and cell migration and invasion, we investigated whether MMP-9 is involved in an inhibitory effect on migration and invasion of PoPx. As Fig. 3D indicates, PoPx treatment decreased the expression of MMP-9 in BCPAP cells. Taken together, all of these results implied that PoPx inhibited the migration and invasion of thyroid cancer cells.
Antitumor efficacy of PoPx in a xenograft model of human thyroid cancer BCPAP
To determine whether the antitumor activity of PoPx in vivo is consistent with its effect in vitro, BCPAP tumor-bearing mice were dosed daily at a dose of 62.5 mg kg−1 and 125 mg kg−1 for a total of 24 days before and after inoculation. As shown in Fig. 6C, tumor volumes were significantly lower in PoPx-treated mice as compared to control mice. However, as an indicator of health, body weight did not show a significant difference between these three groups throughout the experiments (Fig. 6D). At the end of the experiments, the tumors were isolated and weighed (Fig. 6A and B). As shown in Fig. 6B, the mean tumor weight was significantly less in PoPx-treated mice than in control mice. To verify the underlying mechanism of PoPx activity, BCPAP-induced tumors collected on day 31 were examined for proliferation and apoptosis. As shown in Fig. 6E and F, PoPx significantly increased CC-3-positive cells and decreased Ki-67-positive cells. In addition, there is increasing evidence to indicate that MMPs have vital roles in tumor invasion and metastasis.39 We also found that treatment with PoPx could inhibit the expression of MMP-9 in BCPAP tumor tissues (Fig. 6G). Overall, these data suggest that PoPx suppresses a thyroid tumor by inhibiting cell proliferation, inducing apoptosis, and blocking metastasis, which is consistent with the in vitro data. More importantly, no pathological changes after PoPx treatment were observed in the heart, liver, spleen, lung and kidney by H&E staining (Fig. 7), implying that this may be a safe and effective agent for thyroid cancer therapy, at least in xenograft tumor models.
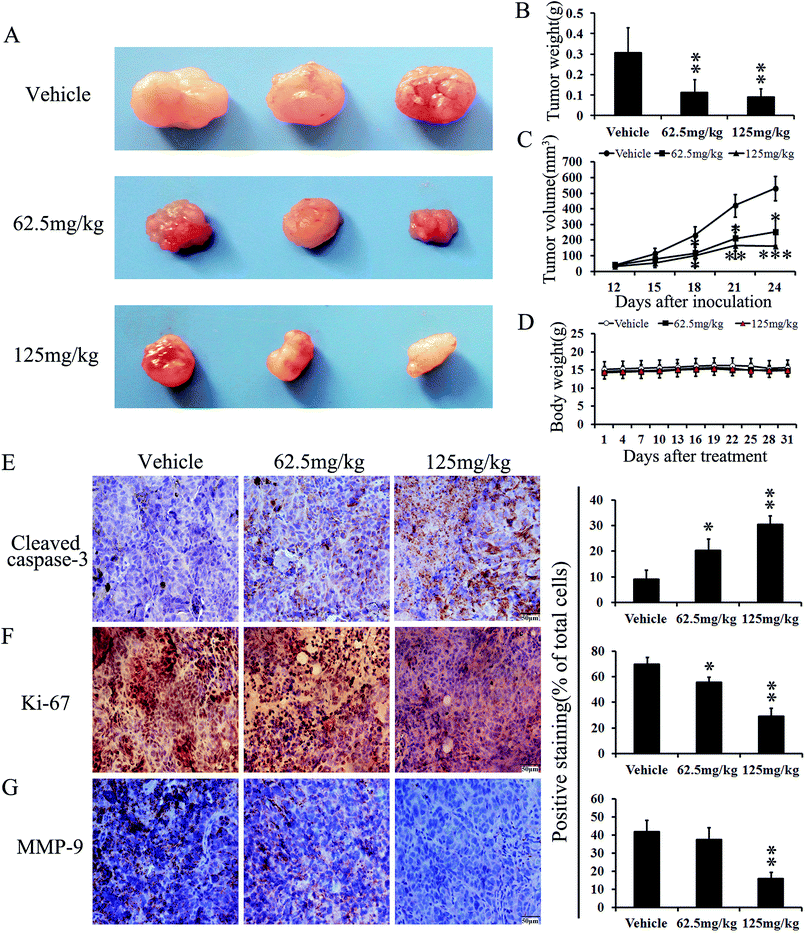 |
| Fig. 6 Antitumor effects of PoPx in vivo. (A) Tumors were visualized to show the inhibitory effect of PoPx on BCPAP tumor 24 days after subcutaneous ectopic cell implantation. (B) Representing weight of tumor from mice of different groups, respectively. Data are expressed as means ± SD (n = 5; **p < 0.01). (C) Tumor size was measured and calculated every three days and presented as means ± SD (n = 5; *p < 0.05, **p < 0.01, ***p < 0.001). (D) The difference in body weight between the two administrated groups and one vehicle group were not significant. Data are expressed as means ± SD. (E–G) Tumors were fixed in formalin, processed for paraffin embedding and then stained with CC-3, Ki-67 and MMP-9 using the DAB Detection Kit. Images shown are representatives from each group (×20). (E) Apoptosis was measured on paraffin-embedded BCPAP tumor sections by CC-3 immunohistochemical staining. The statistical data of CC-3 positive cell number are shown on the right. (F) Tumor cell proliferation was evaluated through immunohistochemical analysis staining with Ki-67, and the statistical data of Ki-67 positive cell number are shown on the right. (G) Immunohistochemistry was performed to measure the expression of MMP-9 in tumor tissues isolated from vehicle and PoPx-treated mice. The treatment with PoPx reduced the MMP-9-positive cells compared to the vehicle group (*p < 0.05; **p < 0.01 vs. vehicle control). | |
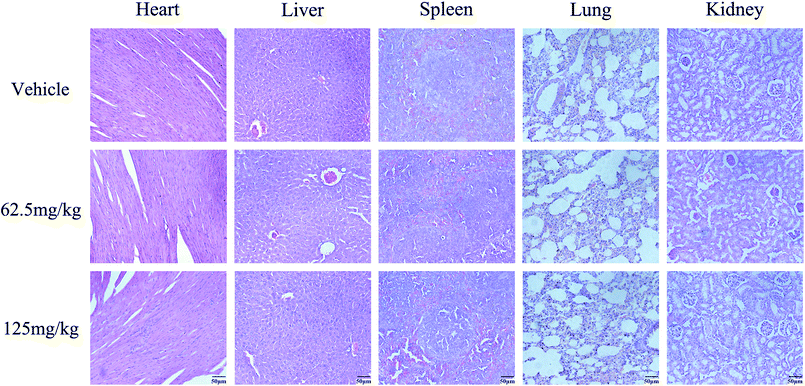 |
| Fig. 7 Evaluation of the damage of PoPx to visceral organs of animals. Mice were divided into 3 groups and intragastrically administered (i.g.) with PoPx 125 mg kg−1, 62.5 mg kg−1 or vehicle. After 31 days, the animals were sacrificed and the main organs were obtained for further study. H&E staining of paraffin-embedded sections of heart, liver, spleen, lung and kidney are shown from BCPAP tumor-bearing mice (×20). | |
Discussion
The incidence of thyroid cancer has been rapidly increasing worldwide in recent years and the prevalence among women is about three times that of men, especially among those aged 25–65.2 Although thyroid cancer has a relatively good prognosis, cervical lymph node metastasis and aggressive subset are highly associated with the risk of recurrence or death.5 In addition, as the major treatment for thyroid cancer, surgery inevitably affects the patient's quality of life and it also requires strict indications and contraindications.6 Therefore, the development of new clinical approaches for the treatment of thyroid cancer is essential.
Herbal plants have universally been the basis for almost all medicinal therapies throughout human history.8,9 Plant extracts have drawn great attention in the fields of pharmaceutics and food since the late 19th century. Recently, natural extracts used in anti-cancer studies have been reported continually: potato extracts kill cancer stem cells,40 magnolia extracts hold an anti-cancer effect,41 cinnamon extracts suppress cancer cell growth,42 rose hip extracts treat triple-negative breast cancer (ER-, PR-, and HER2-).43 On account of their safety and nutrition, phytochemicals would be a promising strategy to provide a supplementary approach for cancer prevention and treatment. Currently, lots of research on different parts of the pomegranate has been reported and it is known for containing enormous antioxidant and anticancer activity.19,20 Our study, therefore, focused on the anticancer effects on thyroid cancer of the extract of pomegranate peel (PoPx).
In this study, PoPx, a kind of herbal extract, was evaluated for its potency against thyroid cancer in vitro and in vivo. We firstly detected the content of PC and EA in PoPx, which was 479.82 mg g−1 and 7.53 mg g−1, respectively. These polyphenols constituted the main composition of the extract and played a crucial role in anticancer activity.44,45 The MTT assay results showed that PoPx decreased the viability of thyroid cancer cells, and colony formation assay data indicate that PoPx inhibited the proliferation of thyroid cancer cells in a time- and dose-dependent manner. Moreover, classic apoptotic morphological changes were observed in drug-treated cells, including reduction in cell volume and formation of a circular morphology; these were not seen in the control group cells.
Apoptosis, a cell suicide program, maintains tissue homeostasis and eliminates unwanted or damaged cells from multicellular organisms, which is fundamental for their development.46 Recently, with in-depth studies of cell apoptosis, scientists have realized that apoptosis is a key mechanism in tumor cell death.47 Hoechst 33258 staining and FCM assays both revealed that PoPx treatment induced apoptosis of thyroid cancer cells in a concentration-dependent manner. There are two main kinds of apoptosis pathway: the cell death receptor-mediated extrinsic pathway and the mitochondrial-mediated intrinsic pathway. The Bcl-2 family pro-apoptotic protein Bax is involved in the induction of intrinsic apoptosis.48,49 In our study, Bax was up-regulated. Furthermore, we found that PoPx significantly increased ROS production in BCPAP and TPC-1 cells. This was consistent with our result of the dose-dependent decrease in the mitochondria membrane potential. In this regard, PoPx may activate some other signaling pathway results in apoptosis. This warrants further investigation. Therefore, in part, these data indicated that PoPx treatment induced apoptosis in thyroid cancer might be through a ROS-mediated mitochondrial apoptotic pathway.
Since not all compounds that have potent antitumor activity in vitro could exhibit anticancer activity in vivo, we examined the antitumor effects of PoPx in our established BCPAP tumor model in BALB/c athymic mice. The results showed that tumor growth was significantly inhibited by PoPx administration (125 mg kg−1 d−1) with an inhibitory rate on 69.8%. Meanwhile, increased expression of CC-3 and reduced expression of Ki-67 in tumor tissue were observed after PoPx treatment compared with the untreated groups.
Thyroid cancer starts as a local disease, but it can also metastasize to the lymph node, lung and other organs.50 The metastatic process is complex: tumor cells need to enter into the blood or lymph vessels as well as extravasate into the secondary organs. Therefore, tumor cell migration and invasion is a vital step in successful cancer metastasis, so inhibiting this step is a practical approach to anti-metastasis treatment. It has been reported that a focal adhesion kinase (FAK)/MMP-involved pathway is critical to cancer invasion and metastasis.51 MMP-9 up-regulation can significantly enhance tumor cell metastatic potential in thyroid cancer.52 In this study, PoPx down-regulated MMP-9 expression in BCPAP cells and tumor tissues. The results suggested that PoPx may inhibit thyroid cancer metastasis by blocking MMP's signaling pathway.
In summary, our study provided important information regarding the antitumor activities of Punica granatum (pomegranate) peel extract (PoPx) in thyroid cancer and showed that PoPx inhibits thyroid cancer cell proliferation, migration and invasion, and induces cell apoptosis through the mitochondria-mediated intrinsic pathway. Furthermore, PoPx may have a particularly high potential for thyroid cancer treatment, as supported by its profound inhibitory effect on the growth of xenograft thyroid tumors without significant visceral organ injury. In addition, PoPx is easy to produce. Although this is the first study to demonstrate the antitumor effect of PoPx in thyroid cancer, the compelling evidence indicated that PoPx might be a potential candidate for blocking thyroid cancer growth and metastasis.
Acknowledgements
The authors would like to thank Chen Fan for her technical assistance. This study was funded by the grants from the National Natural Science Foundation of China (81500054 and 81402564), China Postdoctoral Science Foundation (2015M570788 and 2016T90859), Technology Department of Sichuan Province (2014SZ0170 and 2015SZ0174).
References
- B. A. Kilfoy, T. Zheng, T. R. Holford, X. Han, M. H. Ward, A. Sjodin, Y. Zhang, Y. Bai, C. Zhu, G. L. Guo, N. Rothman and Y. Zhang, Canc. Causes Contr., 2009, 20, 525–531 CrossRef PubMed.
- P. Nix, A. Nicolaides and A. P. Coatesworth, Int. J. Clin. Pract., 2005, 59, 1340–1344 CrossRef CAS PubMed.
- S. K. G. Grebe, Expert Rev. Endocrinol. Metab., 2009, 4, 25–43 CrossRef CAS.
- P. Perros, K. Boelaert, S. Colley, C. Evans, R. M. Evans, G. G. Ba, J. Gilbert, B. Harrison, S. J. Johnson and T. E. Giles, Clin. Endocrinol., 2014, 81(S1), 1–122 CrossRef CAS PubMed.
- S. Yamashita, N. Amino and Y. K. Shong, Thyroid, 2011, 21, 577–580 CrossRef PubMed.
- S. J. Mandel and L. Mandel, Thyroid, 2003, 13, 265–271 CrossRef CAS PubMed.
- R. M. Carneiro, B. A. Carneiro, M. Agulnik, P. A. Kopp and F. J. Giles, Cancer Treat. Rev., 2015, 41, 690–698 CrossRef CAS PubMed.
- J. M. Luk, X. Wang, P. Liu, K. F. Wong, K. L. Chan, Y. Tong, C. K. Hui, G. K. Lau and S. T. Fan, Liver Int., 2007, 27, 879–890 CrossRef CAS PubMed.
- Y. Chen, J. Zhu and W. Zhang, Anticancer Drugs, 2014, 25, 983–991 CrossRef CAS PubMed.
- T. S. Cheung, T. H. Song, T. B. Ng, F. H. Wu, L. X. Lao, S. C. Tang, J. C. Ho, K. Y. Zhang and S. C. Sze, Curr. Med. Chem., 2015, 22, 2392–2403 CrossRef CAS PubMed.
- G. D. Sun, C. Y. Li, W. P. Cui, Q. Y. Guo, C. Q. Dong, H. B. Zou, S. J. Liu, W. P. Dong and L. N. Miao, J. Diabetes Res., 2016, 5749857 Search PubMed.
- A. Wang, L. Lin and Y. Wang, Am. J. Chin. Med., 2015, 43, 601–620 CrossRef CAS PubMed.
- S. T. Lee, M. H. Lu, L. H. Chien, T. F. Wu, L. C. Huang and G. I. Liao, BMC Complementary Altern. Med., 2013, 13, 2049–2057 Search PubMed.
- S. M. Finegold, P. H. Summanen, K. Corbett, J. Downes, S. M. Henning and Z. Li, Nutrition, 2014, 30, 1210–1212 CrossRef CAS PubMed.
- F. Les, J. M. Prieto, J. M. Arbonesmainar, M. S. Valero and V. Lopez, Food Funct., 2015, 6, 2049–2057 CAS.
- S. Akhtar, T. Ismail, D. Fraternale and P. Sestili, Food Chem., 2015, 174, 417–425 CrossRef CAS PubMed.
- G. Yuan, H. Lv, B. Yang, X. Chen and H. Sun, Molecules, 2015, 20, 11034–11045 CrossRef CAS PubMed.
- S. R. Foss, C. V. Nakamura, T. Uedanakamura, D. A. Cortez, E. H. Endo and B. P. D. Filho, Ann. Clin. Microbiol. Antimicrob., 2014, 13, 1–6 CrossRef PubMed.
- D. Z. Lantzouraki, V. J. Sinanoglou, T. Tsiaka, C. Proestos and P. Zoumpoulakis, RSC Adv., 2015, 5, 101683–101692 RSC.
- D. Z. Lantzouraki, V. J. Sinanoglou, P. G. Zoumpoulakis, J. Glamoclija, A. Ciric, M. Sokovic, G. Heropoulos and C. Proestos, RSC Adv., 2015, 5, 2602–2614 RSC.
- S. Modaeinama, M. Abasi, M. M. Abbasi and R. J. Esfahlan, Asian Pacific Journal of Cancer Prevention: APJCP, 2015, 16, 5697–5701 CrossRef PubMed.
- M. I. Waly, A. Ali, N. Guizani, A. S. Al-Rawahi, S. A. Farooq and M. S. Rahman, Asian Pacific Journal of Cancer Prevention: APJCP, 2012, 13, 4051–4055 CrossRef PubMed.
- M. N. Aslam, E. P. Lansky and J. Varani, J. Ethnopharmacol., 2006, 103, 311–318 CrossRef CAS PubMed.
- M. Dikmen, N. Ozturk and Y. Ozturk, J. Med. Food, 2011, 14, 1638–1646 CrossRef CAS PubMed.
- P. Panichayupakaranant, A. Itsuriya and A. Sirikatitham, Pharm. Biol., 2010, 48, 201–205 CrossRef CAS PubMed.
- U. A. Fischer, R. Carle and D. R. Kammerer, Food Chem., 2011, 127, 807–821 CrossRef CAS PubMed.
- F. Wang, D. Scoville, X. C. He, M. M. Mahe, A. Box, J. M. Perry, N. R. Smith, N. Y. Lei, P. S. Davies and M. K. Fuller, Gastroenterology, 2013, 145, 383–395 CrossRef CAS PubMed.
- G. Holmquist, Chromosoma, 1975, 49, 333–356 CrossRef CAS PubMed.
- A. Adan, G. Alizada, Y. Kiraz, Y. Baran and A. Nalbant, Crit. Rev. Biotechnol., 2016, 14, 1–14 CrossRef PubMed.
- I. Rowe, M. Chiaravalli, V. Mannella, V. Ulisse, G. Quilici, M. Pema, X. W. Song, H. Xu, S. Mari and F. Qian, Nat. Med., 2013, 19, 488–493 CrossRef CAS PubMed.
- Q. Zhang, D. Hou, Z. Luo, P. Chen, B. Lv, L. Wu, Y. Ma, Y. Chu, H. Liu and F. Liu, Cell Death Dis., 2015, 6, e1867 CrossRef CAS PubMed.
- Y. Zhu, Y. Xia, T. Ye, X. Shi, X. Song, L. Liu, J. Zeng, N. Wang, Y. Luo and Y. Han, Cell. Physiol. Biochem., 2014, 33, 933–944 CrossRef CAS PubMed.
- K. Garber, Nat. Biotechnol., 2005, 23, 409–411 CrossRef CAS PubMed.
- A. J. Hughes, D. P. Spelke, Z. Xu, C. C. Kang, D. V. Schaffer and A. E. Herr, Nat. Methods, 2014, 11, 749–755 CrossRef CAS PubMed.
- T. Ye, Y. Xiong, Y. Yan, Y. Xia, X. Song and L. Liu, PLoS One, 2014, 9, e85887 Search PubMed.
- E. M. Deqli, Methods, 2002, 26, 335–340 CrossRef.
- A. A. Starkov, Methods Mol. Biol., 2010, 648, 245–255 CAS.
- S. C. Gupta, D. Hevia, S. Patchva, B. Park, W. Koh and B. B. Aggarwal, Antioxid. Redox Signaling, 2012, 16, 1295–1322 CrossRef CAS PubMed.
- S. O. Yoon, S. J. Park, C. H. Yun and A. S. Chung, J. Biochem. Mol. Biol., 2003, 36, 128–137 CrossRef CAS PubMed.
- M. N. Ombra, F. Fratianni, T. Granese, F. Cardinale, A. Cozzolino and F. Nazzaro, Nat. Prod. Res., 2014, 29, 1087–1091 CrossRef PubMed.
- H. Park, H. S. Kim, S. J. Eom, K. T. Kim and H. D. Paik, Molecules, 2015, 20, 12154–12165 CrossRef PubMed.
- H. K. Kwon, J. S. Hwang, J. S. So, C. G. Lee, A. Sahoo, J. H. Ryu, W. K. Jeon, B. S. Ko, C. R. Im and S. H. Lee, BMC Cancer, 2010, 10, 392 CrossRef PubMed.
- P. Cagle, T. Coburn and P. M. Martin, Cancer Res., 2014, 74, 3213 Search PubMed.
- T. Ismail, P. Sestili and S. Akhtar, J. Ethnopharmacol., 2012, 143, 397–405 CrossRef CAS PubMed.
- L. Wang and M. M. Green, Int. J. Mol. Sci., 2014, 15, 14949–14966 CrossRef CAS PubMed.
- L. Sun and X. Wang, Trends Biochem. Sci., 2014, 39, 587–593 CrossRef CAS PubMed.
- M. S. Ricci and W. X. Zong, Oncologist, 2006, 11, 342–357 CrossRef CAS PubMed.
- S. A. Quast, A. C. Berger and J. Eberle, Cell Death Dis., 2013, 4, e839 CrossRef CAS PubMed.
- W. Shen, R. Du, J. Li, X. Luo, S. Zhao, A. Chang, W. Zhou, R. Gao, D. Luo, J. Wang, N. Hao, Y. Liu, Y. Chen, Y. Luo, P. Sun, S. Yang, N. Luo and R. Xiang, Signal Transduction and Targeted Therapy, 2016, 1, 16013 CrossRef.
- J. A. Morrison, L. A. Pike, G. Lund, Q. Zhou, B. E. Kessler, K. T. Bauerle, S. B. Sams, B. R. Haugen and R. E. Schweppe, Horm. Cancer, 2015, 6, 87–99 CrossRef CAS PubMed.
- Y. L. Jia, L. Shi, J. N. Zhou, C. J. Fu, L. Chen, H. F. Yuan, Y. F. Wang, X. L. Yan, Y. C. Xu and Q. Zeng, Hepatology, 2011, 54, 1808–1818 CrossRef CAS PubMed.
- E. J. Lee, S. J. Lee, S. Kim, S. C. Cho, Y. H. Choi, W. J. Kim and S. K. Moon, Cell. Signalling, 2013, 25, 2025–2038 CrossRef CAS PubMed.
Footnote |
† These authors contributed equally to this work. |
|
This journal is © The Royal Society of Chemistry 2016 |