DOI:
10.1039/C6RA12888B
(Paper)
RSC Adv., 2016,
6, 78755-78761
Influences of the Pb 6s2 lone pair effect and quantum size effect on the diffusion of oxygen atoms on Pb(111) films†
Received
18th May 2016
, Accepted 9th August 2016
First published on 10th August 2016
Abstract
Based on our previous studies revealing quantum oscillations in the adsorption energetics of atomic oxygen on Pb(111) films, here we study all the possible on-surface and subsurface adsorption sites of oxygen atoms on Pb(111) films at different coverages. The Pb 6s2 bilayer oscillation behavior is compared with relative basic physical and chemical properties such as adsorption energy or diffusion, allowing us to conclude that the intensity of the lone pair effect of Pb 6s2 directly correlates with the bilayer oscillation amplitudes of adsorption energy and diffusion barriers. A stronger Pb 6s2 lone pair effect depresses the density of states at the Fermi level, which leads to the prominent bilayer oscillations of the adsorption energy and diffusion barriers. In contrast, a weaker Pb 6s2 lone pair effect frees the Pb 6px and 6py electrons interacting with O atoms near the Fermi level, disrupting the bilayer oscillations.
1. Introduction
Deeply understanding the properties of materials may allow us to control them. Because metal surfaces play important roles in catalysis, molecular self-organization, and corrosion processes, the ability to manipulate surface chemical reactivity on metal thin films is an objective in physics and chemistry. Electrons in the well-defined metal geometry are confined in the direction perpendicular to the metal surface, giving rise to quantum well (QW) states.1–4 The electrons form a series of energy sub bands that cross the Fermi-level in the direction parallel to the metal surface, resulting in the quantum size effect (QSE).5,6 A variety of physical properties of the metal thin films have been found being affected by the quantum size effects, including the thermal stability, electron–phonon coupling, work function, superconducting transition temperatures, and surface reactivity. Moreover, the quantum size effect also plays an important role in determining the diffusion barrier and growth morphology. For example, the surface energy and work function of ultrathin lead (Pb) exhibits substantial bilayer oscillatory behavior because of the QSE. Then, numerous experimental and theoretical studies7–10 have demonstrated that the QSE can cause selective adsorption and enhancement of chemical processes on the terraces of wedge-shaped thin Pb(111) films formed on stepped Si(111) substrates. The adsorption energy, dissociation barriers and diffusion barriers of ultrathin Pb films present a bilayer oscillatory behavior. Although some correlations between surface reactivity (e.g., adsorption, dissociation, diffusion, and penetration) and the QSE have been discussed,11–13 the microscopic mechanisms that relate the QSE to the experimentally observed selectivity for specific chemical reactions are still not well understood.
In this paper, we focus on the microscopic mechanisms that relate to the QSE by investigating how the outermost electrons of Pb, especially Pb 6s2 ones, influence the QSE (shown in Fig. 1a), which controls the selective adsorption and chemical processes on stepped or wedge-shaped Pb(111) films. The wedge-shaped Pb(111) films shown in Fig. 1a are constructed through matching with the atomic layer thickness of Si(111), which can keep the surface of the Pb(111) smooth and precisely control the atomic layer thickness of Pb. Unlike previous studies10–16 that focused on the local density of states (DOS) at the Fermi level, surface energy and work function presenting a well-defined up/down oscillation with a period of two monolayers (ML), it is found that the thickness dependence of the energy levels of quantum-well states is found to be responsible for the variations in the physical properties of the film. They just offer a quantitative description of the quantum size effect in Pb thin films using a full consideration of the crystal band structure. Here, we mainly discuss how the different intensities of the lone pair effect of Pb 6s2 affect the electronic states of Pb films. Using first-principles calculations, we show that the QSE will induce a modulated oscillation at the position of Pb 6s2 on the surface of stepped or wedge-shaped Pb(111) films. The intensities of Pb 6s2 lone pair effects affect the amplitudes of bilayer oscillation and the persistence across the strips of different thickness on the wedge and influence the growth morphology and reactivity on the flat top of the wedge-shaped Pb(111) films. Such lone pair effects that influence the QSE modulation mechanism should be useful to design metal film geometries to control nanostructure morphologies and selective chemical reactions. We note that although QSEs in metal films have been studied intensively for over a decade, such the effect of lone pairs on the surface of wedge-shaped metal films has not been reported. The influence of such a lone pair effect on surface properties was not considered in any previous theoretical interpretations of the interesting phenomena observed on wedge-shaped Pb(111) films. In addition, how the surface oxide chemical reactions such as diffusion and penetration from on-surface to subsurface correspond to the intensity of the Pb 6s2 lone pair effect is studied, oxide growth on the Pb surface is considered and the oscillation of energy barriers is systematically investigated.
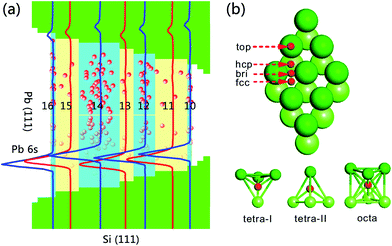 |
| Fig. 1 (a) Three-dimensional view of the thickness-dependent oscillatory adsorption of oxygen (red circles) by a typical Pb(111) mesa grown on a Si(111) substrate. Several thin Pb strips of different thickness are brought into contact to form a stepped or wedge-shaped thin film. From right to left, the thickness increases from 10 to 16 ML. The Pb 6s2 electrons corresponding to the film thicknesses with even (red) and odd (blue) numbers of ML are shown above the diagram. (b) (top) Four on-surface adsorption sites for oxygen atoms including top, hcp, bri and fcc sites. (bottom) Three subsurface adsorption sites including tetra-I, tetra-II, and octa. The surface- and second-layer Pb atoms are shown as dark and light green circles, respectively, and oxygen atoms are shown as smaller red circles. | |
To test our calculations, the electronic structures of clean Pb(111) films are shown in Fig. 2, highlighting the quantum size effect (QSE). As is known, QSE is induced when the particle size is reduced to a certain value. Then, the electronic energy level near the Fermi level is changed from quasi continuous to discrete energy level or wide band gap. To more explicit illustrate the quantum size effect, the band structure corresponding to the total electronic DOS of 5-ML Pb(111) films are illustrated in Fig. 2a. The calculated energies of QW states at Γ points in Pb(111) thin films as a function of thickness are presented in Fig. 2b. The energy dispersion in the bulk along the [111] direction,17 which determines the energy range for QW states, is shown to the right of the QW states. These results agree very well with those of previous theoretical studies,7–10 nearly bilayer oscillations cross the Fermi level, which is consistent with the experimental finding of bilayer stability. To better understand the energy near the Fermi level in Pb(111) films, we denote the energy gap ΔE between the highest occupied QW state and lowest unoccupied QW state. The relationship between ΔE and film thickness is presented in Fig. 2c. The derivative with respect to energy and evaluation at the Fermi level for a given N gives
, where νF is the Fermi velocity obtained from the slope of the band at the Fermi level, and ϕ′(EF) is the energy derivative of the interface electronic phase shift at the Fermi level.
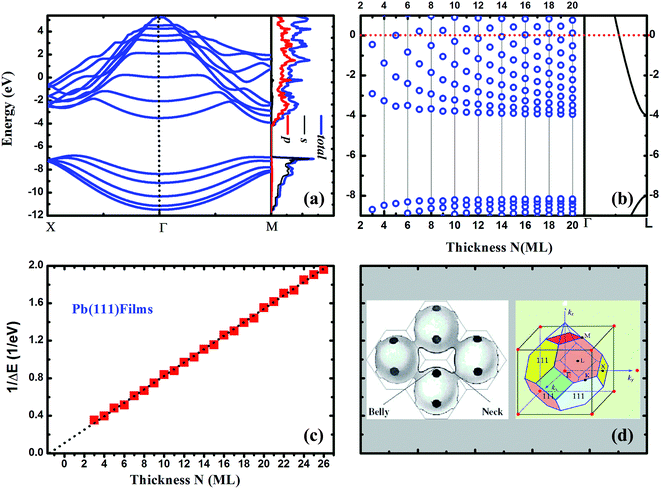 |
| Fig. 2 (a) Calculated bandgaps of 5-ML Pb(111) corresponding to the total and partial density orbital states listed on the right. (b) Calculated energies of QW states at Γ points in Pb(111) thin films as a function of thickness, with the energy set to zero at the Fermi level. The results were obtained using the local density approximation for the experimental lattice constant. The right panel shows the bulk energy dispersion in the (111) direction. (c) Inverse of the energy gap 1/ΔE between the highest and lowest occupied QW states as a function Pb(111) film thickness. The squares are the Γ point in the surface Brillouin zone, and the dashed line is a linear fit to the calculated values. (d) The Pb Fermi surface showing belly and neck regions. The bulk and surface Brillouin zone are also depicted. | |
2. Computational method
Total energy calculations were performed based on density functional theory (DFT) using the Vienna ab initio simulation package,18 in which a plane-wave basis set is used to solve Kohn–Sham equations.19 The generalized gradient approximation (GGA) of Perdew et al.20 and the projector augmented wave (PAW) potentials21 were used to describe the exchange–correlation energy and the electron–ion interaction, respectively. For Pb atoms, the 6s2, 6p2, and 5d electrons were treated as valence electrons. All the Pb layers as well as the oxygen atoms are free to relax until the forces on them are less than 0.02 eV Å−1. The plane-wave energy cutoff is set to be 400 eV. A Fermi broadening22 of 0.1 eV is chosen to smear the occupation of the bands around EF by a finite-T Fermi function and extrapolating it to T = 0 K. Integration over the Brillouin zone was performed using the Monkhorst–Pack scheme23 with 25 × 25 × 1 grid points at Θ = 1.0 coverage and 19 × 19 × 1 grid points at Θ = 0.25 coverage. The nudged elastic band method24 was used to find the minimum energy path and transition state for oxygen (O) atoms to diffuse on and penetrate ultrathin Pb(111) films.
One central quantity tailored for the present study is the average binding energy of the adsorbed O atom defined as Eb = −1/No[Ea − Ec − nEo], where No is the total number of adsorbed O atoms, and n is the layer number. Ea, Ec, and Eo are the total energies of the slabs containing O, the corresponding clean Pb(111) slab, and a free O atom, respectively. According to the definition, Eb is also the adsorption energy per O atom, i.e., the energy that a free O atom gains upon its adsorption. However, because the process of O atom chemisorption involves the dissociation of O2 molecules in most cases, the adsorption energy per O atom can alternatively be referenced to the energy that the O atom has in an O2 molecule by subtracting half the dissociation energy D of the O2 molecule, Ead(1/2O2) = Eb − D/2.
3. Results and discussion
3.1 Lone pair effect in O atom adsorption on the Pb(111) surface/subsurface modulated by the QSE
A stereochemically active lone pair is expected for divalent lead ions due to their formal electronic configuration of 6s2. The formation of Pb 6s2 lone pairs which was previously attributed to intra-atomic hybridization of the Pb 6s2 and 6p2 states has recently been shown to have strong anion dependence. The interaction between Pb 6p2–O 2p bonding states and Pb 6s2–O 2p antibonding states, the degree of the lone pair effect activity can be determined by the ratio of Pb 6s2 states. The lone pairs on Pb are characterized mainly by Pb 6s2–O 2p bonding interactions with energies of 7.5–8.0 eV accompanying a greatly depressed DOS at the Fermi level.25 We calculated the partial DOS of O atoms adsorbed on the Pb(111) surface/subsurface at high coverage and then at lower coverage. By comparing the intensities of Pb 6s2 and O 2p, which reflect the lone pair effects, we found that the most prominent Pb 6s2 lone pair effect on surface adsorption is observed at Θ = 1.0 coverage. There is a marked difference in the electronic interactions especially between Pb 6s2 on-surface adsorption and subsurface adsorption, as shown in Fig. 3a and b, leading to substantial oscillation behavior. To explicate analysis of the lone pair effect, the calculated energy positions of Pb 6s2 for on-surface and subsurface adsorption at Θ = 1.0 coverage are depicted in Fig. 3c and d, respectively. The calculated energy positions of Pb 6s2 clearly oscillate with the film thickness with a bilayer period. The on-surface adsorption has a crossover at the film thicknesses of 7 and 16 ML, proving the existence of the 9-ML oscillation range and that the oscillation orientation is reversed for Pb(111) films with a thickness of 3–7 ML. The on-surface oscillation also persists for a long period (up to 16 ML), different from the subsurface oscillation, which only persists for 7 ML. Meanwhile, for the subsurface adsorption, the smaller lone pair effect decreases the oscillation amplitude compared with that for on-surface adsorption, although the unclear bilayer oscillation behavior does exhibit an obvious crossover at a film thickness of 16 ML. Therefore, the calculated results show that the prominent lone pair effect at the Pb(111) surface can induce clear bilayer oscillation behavior modulated by the QSE. The oscillation of the basic physical properties of materials may be related to prominent lone pair effects.
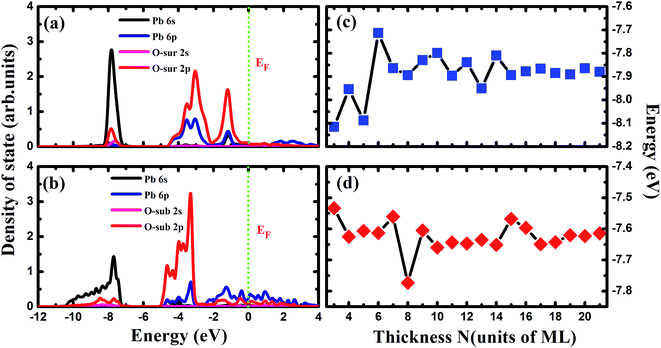 |
| Fig. 3 Orbital-resolved partial DOS for the surface Pb layer and (a) an on-surface O adatom (face-centered cubic site) with coverage Θ = 1.0, and (b) a subsurface O adatom (tetra-II site) with coverage Θ = 1.0. Corresponding (c) on-surface and (d) subsurface calculated positions of Pb 6s2 as a function of the thickness of the Pb(111) film. | |
The above discussions explain the intensity of the of Pb 6s2 can influent the basic physical properties in the system of O/Pb(111). How to define the “intensity” of Pb 6s2 which reflecting the lone pair effect in the O/Pb(111) seems more directly to explain the influent of lone pair effect in the O/Pb(111) system. Luckily, the method used by Jing et al.26,27 can quantitatively analyze the “intensity” of lone pair effect. Here, the “intensity” of lone pair effect can be described by the ratio of Pb 6p states in the Pb 6p–(Pb 6s–O 2p)* states. The ratio can be calculated by relation R(Pb 6p) = I(Pb 6p)/(I(Pb 6s) + I(Pb 6p) + I(O 2p)), where R(Pb 6p) is the ratio of Pb 6p states to Pb 6p–(Pb 6s–O p)* states, and the I(Pb 6s), I(Pb 6p) and I(O 2p) are the intensity of Pb 6s states, Pb 6p states, and O 2p states, respectively. The obtained results can be as an evidence to prove that the intensity of lone pair effects in the O adatom on-surface with coverage Θ = 1.0 are more prominent than that of subsurface.
3.2 Influence of the lone pair effect on O atom adsorption on the Pb(111) surface/subsurface
When gas molecules interact with a surface, many chemical and physical properties such as bonding, charge transfer, electronic structure, and adsorption (binding) energy in the adsorption system change considerably with coverage.28–30 Much of the available research on coverage focuses on the quantities of basic physical properties of materials.31,32 However, the microscopic mechanisms that relate the QSE to basic physical properties such as adsorption (binding) energy are still not well understood. Therefore, it is important to relate the change of physical properties at different coverage with the semicroscopic mechanisms. Here, to determine the lone pair effects of O atom adsorption on the Pb(111) surface/subsurface at coverages of Θ = 1 and 0.25 ML, we calculated the on-surface/subsurface adsorption energies of atomic O at coverages of Θ = 0.25 and 1 ML. The calculated adsorption energy for the on-surface adsorption at 1-ML coverage is shown in Fig. 4a and b, while that for 0.25-ML coverage is displayed in Fig. 4c and d. All the calculated adsorption energies exhibit prominent bilayer oscillation. The adsorption energies for 1-ML coverage with on-surface adsorption and 0.25 ML coverage with on-surface/subsurface adsorption exhibit prominent bilayer oscillation until the films are up to 21 layers thick. This interesting phenomenon agrees well with the above results suggesting that the strongest lone pair effect of the systems is for 1-ML coverage and on-surface adsorption. The prominent Pb 6s2 lone pair effect depressed the DOS at the Fermi level for on-surface adsorption with 1-ML coverage. A weaker Pb 6s2 lone pair effect allowed Pb 6px and 6py to interact with O atoms near the Fermi level, so it is not surprising that the bilayer oscillation is not obvious for the subsurface adsorption.
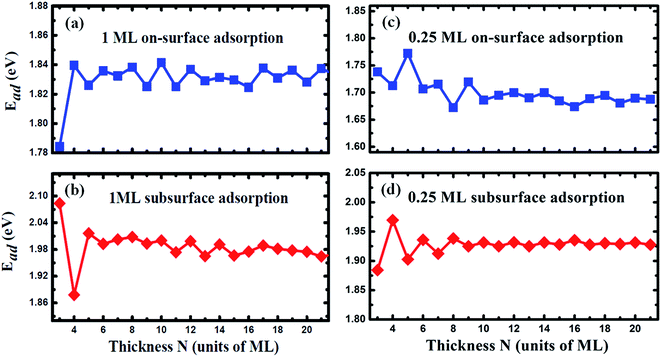 |
| Fig. 4 Adsorption energies for (a) 1-ML on-surface, (b) 1-ML subsurface, (c) 0.25-ML on-surface and (d) 0.25 ML subsurface adsorption of O atoms as a function of Pb(111) film thickness. | |
For the on-surface adsorption, the adsorption energy for 0.25-ML coverage has a crossover at the films with thicknesses of 10 and 17 ML, and the oscillation orientation of the adsorption energy is reversed for films with 1-ML coverage that are 3–11 ML thick. Meanwhile, for the subsurface adsorption, the oscillatory behavior for both 0.25- and 1-ML coverage of O atoms seems to be interrupted, which is not consistent with the behavior observed at the surface.
3.3 Influences of lone pair effects and the QSE on O atom diffusion on the Pb(111) surface/subsurface
The dissociation of O2 molecules on a surface can form patterns of O atom diffusion on the surface or below the surface during the oxidation process. Understanding the diffusion behavior can be used to expand the application of catalytic reactions. Previous studies33 indicate that electron transfer breaks the bonds of O2 molecules and the induced work function produces a modulated oscillatory electrostatic potential on the surface. This means that the surface electrons will encounter a higher energy barrier during O atom diffusion on the surface than that without oxygen present. The electro static potential and related work function modulated by the QSE can display prominent bilayer oscillation, so electron diffusion should also present the same interesting behavior.
The nudged elastic band method,24 which can easily find the saddle points and minimum energy paths on a complicated potential surface, was used to determine the diffusion paths of atomic O both on-surface and subsurface. Fig. 5a shows the on-surface and subsurface diffusion of an O atom on 13-ML films at a coverage of Θ = 0.25. The energy barrier for an O atom adsorbed on-surface to diffuse from the hcp (fcc) to the fcc (hcp) hollow site passes through the bridge hollow site. Meanwhile, an O atom adsorbed at the subsurface diffuses from the tetra-II (tetra-I) to the tetra-I (tetra-II) and octa hollow sites. The on-surface O atom will also penetrate into the subsurface. Here, we focus our attention on the three most energetically favorable diffusion processes on-surface, subsurface and on-to-subsurface. The calculated energy barriers for the fcc-to-hcp, hcp-to-fcc, tetra-II-to-tetra-I, tetra-I-to-tetra-II and fcc-to-octa diffusion of an adsorbed O atom on different Pb(111) films are shown in Fig. 5b–d. The energy barriers of these processes allow us to discuss the influence of the QSE on the diffusion barriers. Bilayer oscillation behavior is clearly seen in Fig. 5b–d for the energy barriers of both on-surface and subsurface diffusion. Similar modulation by the QSE of Pb(111) films is also observed for the on-to-subsurface diffusion of O atoms. Therefore, we believe that the QSE can modulate the diffusion behavior of atomic species on ultrathin metal films. Because of the lower intensity of the QSE of Pb 6s2 in the subsurface than on the surface, the energy barrier is relatively lower in the subsurface compared with those for on-surface and on-to-subsurface diffusion.
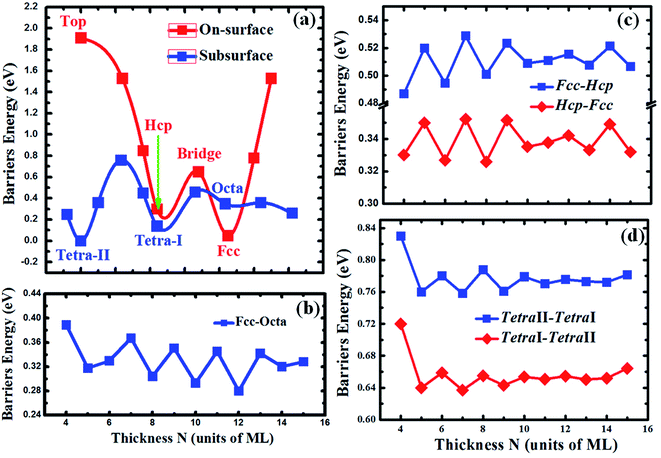 |
| Fig. 5 (a) Total energy of an O atom during on-surface (red squares) or subsurface (blue squares) diffusion on a Pb(111) face for an O coverage of Θ = 0.25. Here the total energy is given with respect to the most stable subsurface tetra-II occupation. (b) Energy barriers for an adsorbed O atom to penetrate into the subsurface along fcc-to-octa as a function of the Pb(111) film thickness. (c) Energy barriers for diffusion of an O oxygen atom on the surface along fcc-to-hcp and hcp-to-fcc as a function of film thickness. (d) Energy barriers for an adsorbed oxygen atom to diffuse on the subsurface along tetra-II-to-tetra-I and tetra-I-to-tetra-II as a function of film thickness. | |
4. Conclusions
Based on DFT, we studied the lone pair effect associated with the QSE on the properties of oxygen-adsorbed Pb thin films, especially the diffusion and penetration behaviors. By considering the Pb 6s2 bilayer oscillation behavior and comparison with relative basic physical or chemical properties such as adsorption energy and diffusion barriers, we found that on-surface high-coverage adsorption has a prominent Pb 6s2 lone pair effect, which depressed the DOS at the Fermi level, causing the bilayer oscillation to become obvious. Meanwhile, in the systems with weaker Pb 6s2 lone pair effects, Pb 6px and 6py interacted with O atoms near the Fermi level, so the bilayer oscillation was not clear. The intensity of the lone pair effect of Pb 6s2 directly correlated with the amplitude of bilayer oscillation. Regarding the diffusion of adsorbed O atoms, we found that the energy barriers of on-surface, subsurface and on-to-subsurface diffusion all oscillated with a bilayer period. Because of the lower intensity of the lone pair effect of Pb 6s2 in the subsurface than that on-surface, the energy barriers for subsurface diffusion are relatively lower than those of on-surface and on-to-subsurface diffusion. These calculated results may be used as a guide to tailor catalysis, chemical reactions, and diffusion of adatoms on the surface of metallic nanomaterials.
Acknowledgements
This work was supported by the National Natural Science Foundation of China [grant number 21403109]. We also acknowledge support from the National Science Foundation of Jiangsu Province [grant number BK20140769], and the Fundamental Research Funds for the Central Universities (No. 30916015106), and Scientific computing and simulation of physical systems (Grant No. U1530401). Calculations were performed on parallel computers at Tianhe2-JK in the Beijing Computational Science Research Center.
References
- T. C. Chiang, Surf. Sci. Rep., 2000, 39, 181 CrossRef CAS.
- J. J. Paggel, T. Miller and T. C. Chiang, Science, 1999, 283, 1709 CrossRef CAS PubMed.
- Z. Y. Zhang, Q. Niu and C. K. Shih, Phys. Rev. Lett., 1998, 80, 5381 CrossRef CAS.
- N. J. Speer, S. J. Tang, T. Miller and T. C. Chiang, Science, 2006, 314, 804 CrossRef CAS PubMed.
- P. Jiang, X. C. Ma, Y. X. Ning, C. L. Song, X. Chen, J. F. Jia and Q. K. Xue, J. Am. Chem. Soc., 2008, 130, 7790 CrossRef CAS PubMed.
- X. Ma, P. Jiang, Y. Qi, J. Jia, Y. Yang, W. Duan, W. X. Li, X. Bao, S. B. Zhang and Q. K. Xue, Proc. Natl. Acad. Sci. U. S. A., 2007, 104, 9204 CrossRef CAS PubMed.
- M. H. Upton, C. M. Wei, M. Y. Chou, T. Miller and T.-C. Chiang, Phys. Rev. Lett., 2004, 93, 026802 CrossRef CAS PubMed.
- Y. Jia, B. Wu, C. Li, T. L. Einstein, H. H. Weitering and Z. Zhang, Phys. Rev. Lett., 2010, 105, 066101 CrossRef PubMed.
- J. Kim, S. Qin, W. Yao, Q. Niu, M. Y. Chou and C.-K. Shih, Proc. Natl. Acad. Sci. U. S. A., 2010, 107, 12761 CrossRef CAS PubMed.
- X. J. Liu, C. Z. Wang, M. Hupalo, H. Q. Lin, K. M. Ho and M. C. Tringides, Phys. Rev. B: Condens. Matter Mater. Phys., 2014, 89, 041401 CrossRef.
- K. Thürmer, E. Williams and J. Reutt-Robey, Science, 2002, 297, 2033 CrossRef PubMed.
- Y. Yang, G. Zhou, J. Wu, W. H. Duan, Q. K. Xue, B. L. Gu, P. Jiang, X. C. Ma and S. B. Zhang, J. Chem. Phys., 2008, 128, 164705 CrossRef PubMed.
- Z. Y. Hu, Y. Yang, B. Sun, X. H. Shao, W. C. Wang and P. Zhang, J. Chem. Phys., 2010, 132, 024703 CrossRef PubMed.
- C. M. Wei and M. Y. Chou, Phys. Rev. B: Condens. Matter Mater. Phys., 2007, 75, 195417 CrossRef.
- P. Czoschke, H. Hong, L. Basile and T.-C. Chiang, Phys. Rev. B: Condens. Matter Mater. Phys., 2005, 72, 075402 CrossRef.
- P. Czoschke, H. Hong, L. Basile and T.-C. Chiang, Phys. Rev. B: Condens. Matter Mater. Phys., 2005, 91, 226801 Search PubMed.
- C. M. Wei and M. Y. Chou, Phys. Rev. B: Condens. Matter Mater. Phys., 2002, 66, 233408 CrossRef.
- G. Kresse and J. Hafner, Phys. Rev. B: Condens. Matter Mater. Phys., 1993, 47, 558 CrossRef CAS.
- G. Kresse and J. Furthmüller, Phys. Rev. B: Condens. Matter Mater. Phys., 1996, 54, 11169 (Comput. Mater. Sci., 1996, 6, 15) CrossRef CAS.
- J. P. Perdew and Y. Wang, Phys. Rev. B: Condens. Matter Mater. Phys., 1992, 45, 13244 CrossRef.
- G. Kresse and D. Joubert, Phys. Rev. B: Condens. Matter Mater. Phys., 1999, 59, 1758 CrossRef CAS.
- M. Weinert and J. W. Davenport, Phys. Rev. B: Condens. Matter Mater. Phys., 1992, 45, 13709 CrossRef.
- H. J. Monkhorst and J. D. Pack, Phys. Rev. B: Solid State, 1976, 13, 5188 CrossRef.
- G. Mills, H. Jonsson and G. K. Schenter, Surf. Sci., 1995, 324, 305 CrossRef CAS.
- B. Sun, P. Zhang, Z. G. Wang, S. Q. Duan, X. G. Zhao and Q. K. Xue, Phys. Rev. B: Condens. Matter Mater. Phys., 2008, 78, 035421 CrossRef.
- Q. Jing, Z. H. Yang, S. L. Pan and D. F. Xue, Phys. Chem. Chem. Phys., 2015, 17, 21968 RSC.
- D. N. Li, Q. Jing, C. Lei, S. L. Pan, B. B. Zhang and Z. H. Yang, RSC Adv., 2015, 5, 79882 RSC.
- L. Y. Ma, L. Tang, Z. L. Guan, K. He, K. An, X. C. Ma, J. F. Jia, Q. K. Xue, Y. Han, S. Huang and F. Liu, Phys. Rev. Lett., 2006, 97, 266102 CrossRef PubMed.
- A. A. Khajetoorians, W. Zhu, J. Kim, S. Qin, H. Eisele, Z. Zhang and C. K. Shih, Phys. Rev. B: Condens. Matter Mater. Phys., 2009, 80, 245426 CrossRef.
- G. Comelli, V. R. Dhanak, M. Kiskinova, K. C. Prince and R. Rosei, Surf. Sci. Rep., 1998, 32, 165 CrossRef CAS.
- B. Sun, P. Zhang, S. Q. Duan, X. G. Zhao and Q. K. Xue, Phys. Rev. B: Condens. Matter Mater. Phys., 2007, 75, 245422 CrossRef.
- H. Over, Y. D. Kim, A. P. Seitsonen, S. Wendt, E. Lundgren, M. Schmid, P. Varga, A. Morgante and G. Ertl, Science, 2000, 287, 1474 CrossRef CAS PubMed.
- O. Bikondoa, C. L. Pang, R. Ithnin, C. A. Muryn, H. Onishi and G. Thornton, Nat. Mater., 2006, 5, 189 CrossRef CAS.
Footnote |
† CCDC 96501. For crystallographic data in CIF or other electronic format see DOI: 10.1039/c6ra12888b |
|
This journal is © The Royal Society of Chemistry 2016 |
Click here to see how this site uses Cookies. View our privacy policy here.