DOI:
10.1039/C6RA12776B
(Paper)
RSC Adv., 2016,
6, 71638-71651
Bis-naphthalimides bridged by electron acceptors: optical and self-assembly characteristics†
Received
17th May 2016
, Accepted 11th July 2016
First published on 11th July 2016
Abstract
The self-assembly of small organic molecules into molecular stacks plays a vital role in the construction of stable supramolecular structures. The self-assembly of highly linear and rigid naphthalimide-based electron deficient small molecules, which are synthesized via a Pd-catalyzed Sonogashira cross coupling reaction, leads to the formation of controlled morphologies and well-defined nanostructures, which are developed from the J-aggregated solid state packing mode. Their optical properties evidence the molecular self-aggregation in solution and the solid state. Fluorescence lifetime decay measurements for the monomeric and aggregated state of the dyes demonstrate the effect of aggregation on the radiative and non-radiative decay rates. Electrochemical studies reveal that these electron deficient compounds possess suitable energy levels for them to be used as airstable n-type semiconductors. The electronic features and coplanar arrangement of the functional entities, which were determined via density functional theoretical computations, ensure a close packing arrangement of molecules in the solid state. Field emission scanning electron microscopy was used to unravel the nanostructure formation of the as synthesized samples and those obtained from different solvents. The formation of one-dimensional (1D) to three dimensional (3D) nano to micro-scale fibers, rods, cubes or flower-like structures is attributed to the non-covalent π–π intermolecular stacking of the aromatic moieties and packing of the hydrophobic alkyl chains. Dynamic light scattering studies reveal the nanoscale dimension of the aggregates. Fluorescence images of the compounds were observed under an optical fluorescence microscope, which exhibit multicolor emission under different light sources. Furthermore, thermal analysis and powder X-ray diffraction characterization of the small molecules indicate high thermal stability, crystallinity and effective π–π interplanar morphology. The present study demonstrates the use of intermolecular interactions to achieve organized nanostructure designs with different morphologies and sizes.
Introduction
In the field of materials science, small organic molecules exhibit defined chemical structures, which make them quite attractive in terms of ease of synthesis and purification, and this greatly improves processing and device-fabrication reproducibility as compared to polymers and inorganic materials.1 They exhibit a strong affinity to self-aggregate into low dimensional structures through different assembly processes. Assembled morphologies, which result in supramolecular materials, have flagged their importance due to their high aspect ratios, and unique optical and electronic properties.2 Nano and microstructures with controllable hierarchical architectures are of increasing interest because of their utilization in broad application fields ranging from medicine to electronics to optoelectronics.3 The morphologies of the formed assemblies are closely related to the stacking mode of the building blocks and can be controlled through the alteration of intermolecular interactions, which are affected by a number of factors such as concentration, temperature and solvent dependence.4 Currently, various approaches, such as the use of templates, reprecipitation, electrochemical methods, and various solution techniques involving weak non-covalent interactions, such as hydrogen bonding, π–π stacking, electrostatic and hydrophobic interactions, are efficiently used for the construction of nanostructures.5 However, only a few examples among the numerous tedious strategies for designing self-assembled nanostructures have been found capable of producing nanostructures for use as potential molecular materials. Moreover, when compared with the synthetic approaches of inorganic nanostructures, very few approaches have been developed for the fabrication of organic small-molecule nanostructures.1j,2h,2j,3c,3d In 2001, Frank Würthner for first time demonstrated the self-assembling properties of organic molecules due to the formation of J-aggregates6 in perylene bisimide with a narrow J-band.7 Later, a number of such molecules were highlighted to show self-assembling supramolecular systems with the aid of various methods, as mentioned earlier. However, only a few reports are known for the self-assembled well defined nano-morphology of as-synthesized organic chromophores.1f,2a,8
π–π intermolecular stacking and hydrophobic interactions are highly explored in organic materials due to the presence of an aromatic core and easy incorporation of hydrophobic alkyl chains.5f,7,8i The general strategy for designing a molecular system for effective intermolecular interactions is to have a large, planar and extended π-core which enhances π-stacking and reduces steric interactions. The supramolecular assembly of extended π-conjugated chromophores,8 such as various polyaromatic hydrocarbons (PAHs),9 including anthracene,10 pyrene,11 perylene,12 perylene diimides (PDI),13 naphthalene diimides (NDI),14 many amide-based conjugated molecules,15 and heteroaromatic systems,16 into defined dimensional nanostructures provides a potential strategy to tune their optical and electronic properties. It is found that the incorporation of the alkyne group between aromatic building blocks is more adaptable to conformational and steric constraints than the alkene moiety due to the electronic symmetry and planarity induction in the molecule.17 Acetylene based functionalized large aromatic core assemblies enhance π–π interactions, reduce the intermolecular spacing between molecules and therefore strengthen the non-covalent interactions.18
It is well known that in organic electronics, n-type semiconductors lag far behind compared to their p-type counterparts due to their low mobility, poor stability and poor solubility for solution processing.19 Moreover, only a small amount of literature has addressed crystalline nano or microscale n-type organic semiconductors. Among the known frameworks, imides and π-conjugated molecules15a have received considerable attention as promising n-type organic semiconducting candidates. We used 1,8-naphthalimide20 because it possesses high carrier mobility, high electron affinity, promising fluorescence properties, chemical and thermal stability and easy functionalization at active C-4 and N-atom positions. Moreover, it facilitates long-range intermolecular charge delocalization of the π-electron cloud and can be explored successfully as a highly organized skeleton in various applications such as biological and cell bioimaging,21 fluorescent sensors22 and optoelectronic devices.23
Herein, we describe the synthesis and characterization of 4-ethynyl-1,8-naphthalimide based planar chromophores (3a–3d), which are shown in Fig. 1. They form self-assembled 1D to 3D nanostructures in a facile manner, which is attributed to effective intermolecular π–π stacking. These small molecules exhibit remarkable optical, electrochemical and thermal properties. The planar skeletons show a high degree of crystallinity and solubility in various organic solvents owing to the n-ethylhexyl chain introduced at the N-position.
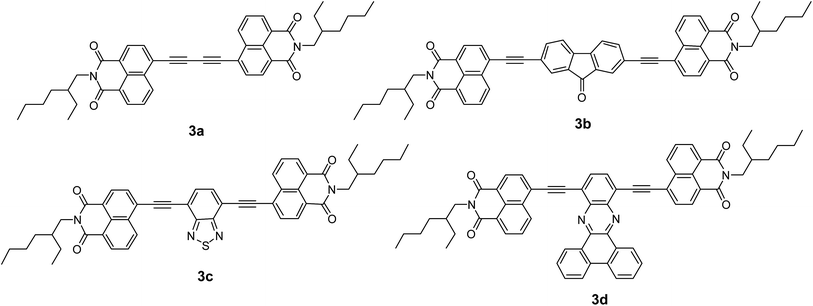 |
| Fig. 1 Structures of the naphthalimide end-capped small molecules. | |
Experimental
General methods and instrumentation
All commercial chemicals were used as received. Column chromatography was performed using silica gel (100–200 mesh) as the stationary phase. All solvents used in the synthesis and spectroscopic measurements were distilled over appropriate drying and/or degassing reagents. 1H and 13C NMR spectra were obtained on a Bruker FT-NMR and JEOL spectrometer operating at 500 MHz and 100 MHz, respectively, in CDCl3. Me4Si (0.00 ppm) or residual signals for CHCl3 (1H NMR, δ = 7.26 ppm; 13C NMR, δ = 77.23 ppm) served as the internal standard. IR spectra were obtained on an FT-IR spectrometer. High resolution mass spectra were obtained using the electron spray ionization (ESI) mode on a Bruker TOF-Q ESI mass spectrometer operating in positive ion mode. UV-Vis spectra were obtained at RT in quartz cuvettes on a Cary UV-100 spectrophotometer. Fluorescence measurements were conducted using freshly prepared diluted solutions and performed on an RF-5301-PC Shimadzu spectrofluorophotometer. Drop-cast thin films on quartz plates were prepared from a toluene solution and used for the measurement of solid state photoluminescence. The solid state emissions of the powders were recorded on a Horiba FluoroMax-4 spectrofluorometer. Fluorescence quantum yields (ΦF) were determined using the formula Φs = (Φr × Ar × Is × ηs2)/(As × Ir × ηr2), where A is the absorbance at the excitation wavelength, I is the integrated area under the fluorescence curve, and η is the refraction index. Subscripts r and s refer to the reference and the sample of unknown quantum yield, respectively. For a 90% water–THF, the refraction index (η) of 0.34 was calculated using the formula ηwater × Vwater + ηTHF × VTHF/Vwater + VTHF. Coumarin-6 in ethanol (ΦF = 0.78) was taken as the reference. Absolute solid state fluorescent quantum yield (±3% accuracy) was measured via the integrating sphere method using an Edinburgh FLS980 fluorescence spectrometer. Fluorescence lifetime decay measurements were recorded in a 1 cm quartz cell on a Horiba Jobin Yvon “FluoroCube Fluorescence Lifetime System” equipped with NanoLEDs and LDs as the excitation source(s) and an automated polarization accessory (Model 5000 U-02). Cyclic voltammetry (CV) measurements were performed using a BASi Epsilon electrochemical analyzer. All measurements in dichloromethane (DCM) solution were conducted at room temperature with the conventional three-electrode configuration comprising a glassy carbon working electrode, a platinum wire counter, and a nonaqueous acetonitrile Ag/AgNO3 reference electrode under nitrogen atmosphere. Potentials are quoted against a ferrocene internal standard. The solvent used in all measurements was dichloromethane (DCM) and the supporting electrolyte was 0.1 M tetrabutylammonium hexafluorophosphate (TBAP) at a scan rate of 100 mV s−1. Thermogravimetric analyses (TGA) were performed under nitrogen atmosphere at a heating rate of 10 °C min−1 using an SII 6300 EXSTAR analyser. The thermal transitions of the dyes were investigated on a Netzsch DSC 204F1 Phoenix differential scanning calorimeter (DSC) under nitrogen atmosphere at a heating rate of 10 °C min−1. Powder X-ray diffraction was performed using a Bruker D8 Advance diffractometer over the 2θ range of 5–90° and the scan rate was 1° min−1 for the copper target (Cu-Kα; λ = 1.5406 Å). Scanning electron microscopy (SEM) images of the samples were recorded using an FE-SEM Quanta 200 FEG instrument operated at 15–20 kV on substrates sputtered by gold. Samples for the SEM analysis were investigated in powder form or prepared via a drop-cast method from dye aggregates in various solvent systems on glass substrates. Dynamic light scattering (DLS) particle size distribution was measured on a Malvern Zetasizer Nano-ZS90, which was obtained from the intensity of the aggregates. Fluorescence microscopic images were acquired using a Nikon Eclipse LV100 microscope by air drying a drop of the diluted samples on a glass slide under various excitation filters such as B-2A (450–490 nm) and G-2A (510–560 nm) filter excitation. All images were taken at 100× magnification.
Synthesis of 2-(2-ethylhexyl)-6-ethynyl-1H-benzo[de]isoquinoline-1,3(2H)-dione, 2
To a two neck R.B. flask 6-bromo-2-(2-ethylhexyl)-1H-benzo[de]isoquinoline-1,3(2H)-dione (1) (13.0 g, 33.5 mmol), triphenylphosphine (176 mg, 0.2 mmol, 2 mol%), cuprous iodide (64 mg, 0.1 mmol, 1 mol%) and Pd(PPh3)2Cl2 (235 mg, 0.1 mmol, 1 mol%) were added and purged with N2 for 10 min. Then, trimethylsilylacetylene (4.0 g, 40.2 mmol, 1.2 eq.) was added and N2 was passed through the mixture for 5 min, then distilled degassed TEA 160 mL was added immediately and the mixture was kept stirring at r.t. After completion of the reaction was confirmed by TLC, the TMS intermediate was extracted using DCM and washed with brine water, dried over Na2SO4 and the solvent evaporated to yield a brown liquid, which was purified by silica column chromatography (eluent: CHCl3
:
hexane, 1
:
1) followed by deprotection in 50 mL distilled methanol and to the resulting intermediate 30 mmol each of KF and Bu4NBr and 10 mL distilled DCM was added and stirred for 30 minutes at 60 °C. The reaction mixture was cooled and water was added, which was extracted with DCM and washed thoroughly with water and dried to obtain a viscous liquid that solidified on cooling and then adsorbed on silica for chromatography (eluent: CHCl3
:
hexanes, 1
:
1). Yellow solid; yield 7.0 g (77%); mp: 82–84 °C; IR (KBr, cm−1) 1698 (νC
O), 2098 (νC
C), 3218 (νC
CH); 1H NMR (CDCl3, 500 MHz) δ 8.66 (d, J = 8.5 Hz, 2H), 8.63 (d, J = 7.0 Hz, 1H), 7.94 (d, J = 7.5 Hz, 1H), 7.83 (t, J = 7.5 Hz, 1H), 4.14–4.07 (m, 2H), 3.73 (s, 1H), 1.96–1.91 (m, 1H), 1.40–1.29 (m, 8H), 0.93 (t, J = 7.5 Hz, 3H), 0.87 (t, J = 7.0 Hz, 3H); 13C NMR (CDCl3, 100 MHz) δ 164.5, 164.3, 132.3, 132.1, 131.94, 131.85, 130.4, 128.1, 127.9, 126.3, 123.1, 123.0, 86.7, 86.5, 44.4, 38.1, 30.9, 28.9, 24.2, 23.3, 14.3, 10.9; HRMS (ESI, m/z) [M]+ calcd for C22H23NO2: 333.1723; found 333.1748.
General procedure for the synthesis of dyes 3b–d and byproduct 3a
Aryl dibromide (1 mmol), 2-(2-ethylhexyl)-6-ethynyl-1H-benzo[de]isoquinoline-1,3(2H)-dione (2) (0.668 g, 2 mmol) was added to a 100 mL two neck R.B. flask and N2 gas was passed through the flask for 5 minutes, then Pd(PPh3)2Cl2 (14 mg, 0.02 mmol), CuI (4 mg, 0.02 mmol), and PPh3 (11 mg, 0.04 mmol) were added and purged with nitrogen for 10 minutes. Then, degassed distilled triethylamine (20 mL) was added under a nitrogen atmosphere. Furthermore, the reaction mixture was refluxed for 5–12 h and monitored using TLC, and upon completion water was added to quench the reaction. The mixture was extracted with DCM and washed thoroughly with water. Then, the solvent was evaporated and absorbed on silica for purification.
6,6′-(Buta-1,3-diyne-1,4-diyl)bis(2-(2-ethylhexyl)-1H-benzo[de]isoquinoline-1,3(2H)-dione), 3a. This was obtained as a side product (yield: 0.15 g (23%)) during the preparation of 3b. It can also be prepared using the following oxidative coupling strategy in good yield.23a A mixture of 2 (0.668 g, 2 mmol), CuI (28 mg, 1.5 μmol) and triethylamine (10 mL) was allowed to react at room temperature under an N2 atmosphere for 12 h. After the completion of the reaction, the volatiles were removed under vacuum. The residue was purified by column chromatography using a CHCl3
:
hexanes mixture (3
:
2) as the eluent. Yield: 0.50 g (75%), yellow solid, mp: 119 °C; IR (KBr, cm−1) 1699 (νC
O); 1H NMR (CDCl3, 500 MHz) δ 8.70 (d, J = 8.5 Hz, 2H), 8.67 (d, J = 8.5 Hz, 2H), 8.57 (d, J = 7.5 Hz, 2H), 8.05 (d, J = 7.5 Hz, 2H), 7.89 (t, J = 7.5 Hz, 2H), 4.17–4.08 (m, 4H), 1.97–1.92 (m, 2H), 1.41–1.28 (m, 16H), 0.94 (t, J = 7.0 Hz, 6H), 0.88 (t, J = 7.0 Hz, 6H); 13C NMR (CDCl3, 100 MHz) δ 164.3, 164.0, 132.8, 132.5, 132.1, 132.1, 130.3, 128.3, 128.2, 125.3, 123.6, 123.4, 82.2, 81.7, 44.5, 38.1, 30.9, 28.9, 24.2, 23.3, 14.3, 10.8; HRMS (ESI, m/z) [M + H]+ calcd for C44H44N2O4: 665.3373; found 665.3342.
6,6′-((9-Oxo-9H-fluorene-2,7-diyl)bis(ethyne-2,1-diyl))bis(2-(2-ethylhexyl)-1H-benzo[de]isoquinoline-1,3(2H)-dione), 3b. Purification by silica column chromatography using a CHCl3
:
hexanes mixture (7
:
3) as the eluent to yield 0.3 g of the desired orange solid and 0.4 g of yellow mono coupled bromoderivative solid. Yield: 30%; mp: 296 °C; IR (KBr, cm−1) 1699 (νC
O), 2200 (νC
C); 1H NMR (CDCl3, 500 MHz) δ 8.68 (d, J = 8.0 Hz, 2H), 8.65 (d, J = 6.0 Hz, 2H), 8.55 (d, J = 7.5 Hz, 2H), 7.97–7.95 (m, 4H), 7.86 (t, J = 7.5 Hz, 2H), 7.82 (d, J = 8.5 Hz, 2H), 7.62 (d, J = 8.5 Hz, 2H), 4.15–4.07 (m, 4H), 1.96–1.93 (m, 2H), 1.39–1.30 (m, 16H), 0.93 (t, J = 7.5 Hz, 6H), 0.87 (t, J = 7.0 Hz, 6H); 13C NMR (CDCl3, 100 MHz) δ 191.8, 164.4, 164.1, 143.9, 138.4, 134.5, 132.1, 131.9, 131.6, 131.2, 130.4, 128.1, 127.9, 126.7, 123.9, 123.2, 122.6, 121.2, 97.9, 88.7, 44.4, 38.1, 30.9, 28.9, 24.2, 23.3, 14.3, 10.8; HRMS (ESI, m/z) [M]+ calcd for C57H50N2O5: 842.3714; found 842.3724.
6,6′-(Benzo[c][1,2,5]thiadiazole-4,7-diylbis(ethyne-2,1-diyl))bis(2-(2-ethylhexyl)-1H-benzo[de]isoquinoline-1,3(2H)-dione), 3c. Purification by silica column chromatography using a CHCl3
:
hexanes mixture (1
:
1) as the eluent. Orange solid, yield: 0.6 g (75%); mp: 272 °C; IR (KBr, cm−1) 1704 (νC
O), 2199 (νC
C); 1H NMR (CDCl3, 500 MHz) δ 9.02 (d, J = 8.5 Hz, 2H), 8.84 (d, J = 7.0 Hz, 2H), 8.59 (d, J = 8.5 Hz, 2H), 8.08 (d, J = 8.5 Hz, 2H), 7.97 (d, 2H), 7.94 (t, J = 7.5 Hz, 2H), 4.17–4.08 (m, 4H), 1.98–1.93 (m, 2H), 1.42–1.29 (m, 16H), 0.94 (t, J = 7.5 Hz, 6H), 0.88 (t, J = 7.0 Hz, 6H); 13C NMR (CDCl3, 100 MHz) δ 164.4, 164.2, 154.6, 132.9, 132.6, 132.0, 131.9, 131.4, 130.5, 128.3, 128.2, 126.5, 123.2, 123.2, 117.4, 95.1, 94.4, 44.4, 38.0, 30.8, 28.8, 24.1, 23.2, 14.2, 10.7; HRMS (ESI, m/z) [M + H]+ calcd for C50H46N4O4S: 799.3312; found 799.3298.
6,6′-(Dibenzo[a,c]phenazine-10,13-diylbis(ethyne-2,1-diyl))bis(2-(2-ethylhexyl)-1H-benzo[de]isoquinoline-1,3(2H)-dione), 3d. Recrystallized with hexanes and CHCl3. Orange solid; yield 0.92 g (97%); mp: 286 °C; IR (KBr, cm−1) 1698 (νC
O), 2200 (νC
C); 1H NMR (CDCl3, 500 MHz) δ 8.88 (d, J = 7.5 Hz, 2H), 8.68 (d, J = 8.0 Hz, 2H), 8.36 (d, J = 6.5 Hz, 2H), 8.27 (d, J = 7.0 Hz, 2H), 8.12 (d, J = 8.0 Hz, 2H), 7.62 (d, J = 7.5 Hz, 2H), 7.59–7.50 (m, 6H), 7.35 (t, J = 7.0 Hz, 2H), 4.11–4.00 (m, 4H), 1.98–1.93 (m, 2H), 1.44–1.35 (m, 16H), 0.98–0.91 (m, 12H); 13C NMR (CDCl3, 100 MHz) δ 163.7, 163.5, 141.7, 140.8, 132.6, 131.9, 131.5, 130.8, 130.4, 130.3, 129.6, 128.8, 127.3, 127.2, 126.9, 126.5, 125.8, 122.9, 122.5, 121.9, 95.9, 94.1, 44.3, 38.1, 30.9, 28.9, 24.1, 23.4, 14.4, 10.7; HRMS (ESI, m/z) [M + Na]+ calcd for C64H54N4O4: 942.4139; found 942.4170.
Computational methods
The Gaussian 09 36 program package was employed to perform all computations, which were fully optimized without any symmetry constrains and employed DFT using Becke's three parameters hybrid functional and Lee, Yang and Parr's correlational functional B3LYP37 using the 6-31G* basis set on all atoms in vacuum. Structure confirmation was performed by vibrational analyses on the optimized structures. The excitation energies and oscillator strengths for the lowest 10 singlet–singlet transitions at the optimized geometry in the ground state were obtained by TD-DFT calculations using the (M06-2x/6-31G(d,p)) method.
Results and discussion
Synthesis and characterization
The synthetic pathway used to prepare the dyes (3a–3d) is shown in Scheme 1. First, the acetylene derivative, 2 was synthesized via a two-step reaction involving the Sonogashira coupling of 6-bromo-2-(2-ethylhexyl)-1H-benzo[de]isoquinoline-1,3(2H)-dione (1) with trimethylsilyl acetylene. The latter compound, 1, was synthesized via the imidization of 4-bromonaphthalene anhydride according to a literature procedure.24 Finally, the naphthalimide-based functional materials were conveniently synthesized by the Sonogashira cross-coupling reaction between 2-(2-ethylhexyl)-6-ethynyl-1H-benzo[de]isoquinoline-1,3(2H)-dione (2) and the respective aryl dibromides25 using the Pd(PPh3)2Cl2/PPh3/CuI catalytic system in good to excellent yields. For 3b, 6-((7-bromo-9-oxo-9H-fluoren-2-yl)ethynyl)-2-(2-ethylhexyl)-1H-benzo[de]isoquinoline-1,3(2H)-dione was synthesized as the major product due to the low reactivity of the respective arylene dibromide. The dye 3a was obtained as a homocoupled byproduct in the cross coupling reactions targeted for the synthesis of the other dyes 3b–3d. All the dyes are intensely colored (yellow or orange) and exhibit bright solid state luminescence. Moreover, they are highly soluble in a wide variety of solvents such as toluene (Tol), tetrahydrofuran (THF), and dichloromethane (DCM). The dyes were thoroughly characterized using spectral methods (NMR, FTIR and HRMS) and the data obtained is found to be consistent with the proposed structures.
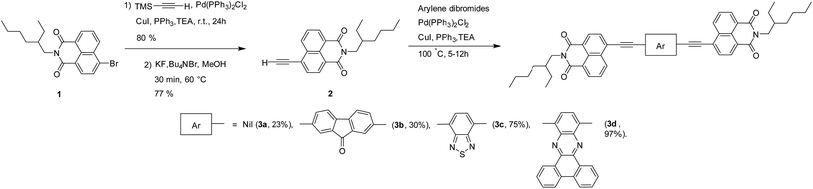 |
| Scheme 1 Synthesis of the compounds. | |
Optical properties
The UV-Vis absorption and emission spectra of the dyes were measured in DCM solution and are displayed in Fig. 2 and the corresponding optical parameters are listed in Table 1. The absorption spectra of the dyes are complex with multiple overlapping π–π* transition bands ranging from 350 to 500 nm. The naphthalimide moiety shows an absorbance band at 350–366 nm.20j In the present dyes, additional red-shifted absorption peaks were noticed. Among the dyes, the dyes containing benzothiadiazole (3c) and dibenzo[a,c]phenazine (3d) in the conjugation pathway exhibited the most red-shifted absorption peaks. This bathochromic shift is attributed to the extension of conjugation by the introduction of an additional electron accepting π-conjugating chromophore. Additional peaks attributable to the localized absorptions originating from the fluorenone and dibenzo[a,c]phenazine chromophores were observed for compounds 3b and 3d.16h,26
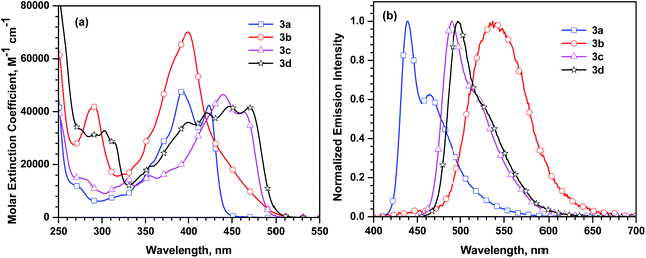 |
| Fig. 2 (a) Absorption and (b) emission spectra of the dyes recorded in DCM. | |
Table 1 Optical and electrochemical data of dyes 3a–3d recorded in DCM
Dye |
λmax, nm (εmax, M−1 cm−1 × 103) |
λema, nm (ΦF%) |
Stokes shift, cm−1 |
Eoxb, V |
Eredb, V |
HOMOd, eV |
LUMOc, eV |
E0-0e, eV |
Relative quantum yield was obtained by comparison with the standard coumarin-6. Redox potentials are reported with reference to the ferrocene internal standard using tetrabutylammonium perchlorate as the electrolyte. Deduced from the reduction potential using the formula LUMO = 4.8 − |Ered|. Obtained from the DPV oxidation potential peak. Calculated using the formula E0-0 = EHOMO − ELUMO. |
3a |
423 (42.4), 392 (47.8), 371 (28.9) |
438, 464 (62) |
810 |
1.51 |
−1.43 |
6.31 |
3.37 |
2.94 |
3b |
445 (20.9) s, 400 (70.0), 291 (41.8) |
540 (24) |
6798 |
1.17, 1.31 |
−1.50, −1.66 |
5.97 |
3.30 |
2.66 |
3c |
463 (39.8), 439 (46.4), 380 (18.1), 354 (15.6), 329 (14.6), 279 (14.6) |
490, 518 (70) |
1237 |
1.23 |
−1.32, −1.84 |
6.03 |
3.48 |
2.55 |
3d |
470 (41.5), 447 (41.9), 422 (39.5), 398 (35.8), 302 (32.7) |
500, 526 (82) |
1322 |
1.16 |
−1.48, −1.84 |
5.96 |
3.32 |
2.65 |
All the dyes, except the fluorenone derivative, are brightly luminescent in dilute solutions. The fluorescence spectra recorded for compounds 3a–3d in dilute DCM solution is displayed in Fig. 2b. The emission peak wavelengths showed a similar trend to that of the absorption with the exception of 3b. The emission profiles of compounds 3a, 3c and 3d exhibited vibronic features, which attest the rigidity of the molecules,17c whereas 3b featured broad structureless emission. The Stokes shift is also significantly large for 3b in the series. In addition, the quantum yield of 3b is the lowest in the group. The abovementioned observations suggest that the dye 3b probably undergoes structural relaxation in the excited state prior to emission.17c
Furthermore, the UV-Vis absorption and emission spectra of the compounds in thin film and solid state (Fig. 3a and b, S1 and Table S1†) were obtained to understand the intermolecular interaction and self-assembly process. A red-shifted absorption band at 20–70 nm was observed in the thin film as compared to the solution spectra, which is attributed to the stronger electronic interaction between the individual molecular skeletons in the solid state.5b,18b,27 Moreover, a broad featureless absorption band with the emergence of a new band was observed, which are attributed to the intermolecular π–π packing interactions or J-aggregation well reported in the literature.20e Such an ordered packing of small molecules is effective for long range charge carrier mobility and self-aggregation. It is well known that fluorescence spectral shifts and changes in the quantum yield for a solid with respect to its solution depend on the molecular packing in the solid state.20h,28 The presence of strong π–π interactions between cofacially stacked molecules lead to red-shifted fluorescence and a decrease in emission efficiency. In the thin film emission spectra of the compounds (Fig. 3a), the bathochromic spectral shift and broadening clearly indicates the presence of intermolecular interactions. Furthermore, the emission of the compounds in the solid state (Fig. 3b) is further red-shifted when compared to that in the thin film and is accompanied by a significant broadening of the fluorescence band with a large full width half maximum (FWHM) (∼90 nm), which is common for solid emitting materials.29 Moreover the quantum yield for the solid (ΦF) is found to be quenched (Table S1†) in comparison to the fluorescence quantum yield (ΦF) in DCM solvent, which supports molecular self-assembly arising from strong π–π intermolecular interactions.
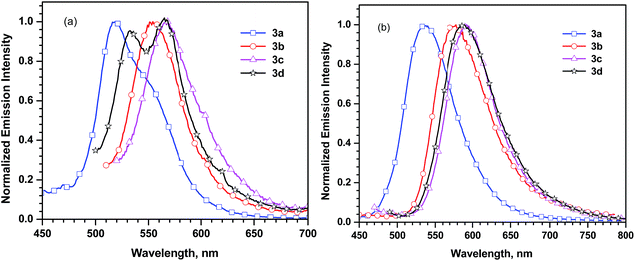 |
| Fig. 3 Emission spectra of the dyes as (a) drop-cast thin films and (b) solid powder. | |
Self-assembly studies by absorption and emission spectra
The self-assembly of the compounds was investigated via absorption and emission measurements in a binary THF
:
H2O solvent system (Fig. 4 and S2–4†). The aggregation behaviours of the compounds were confirmed by the gradual change in the ratio of water to solvent. The solution color of the dyes was found to change from 100% THF to 40–60% H2O accompanied by a new 20–30 nm red shifted absorption shoulder as compared to the π–π* transitions in pure THF solvent. On moving from 100% THF to 90% water, the absorption spectra showed a hypochromic shift upon an increase in water content, which suggests π-stacking among the chromophores. The levelling off of the spectra observed in the longer wavelength region at high water content is attributed to the scattering of light due to the formation of aggregates. This result clearly points towards the occurrence of π–π stacking in the aggregates due to the formation of self-assembled structures.
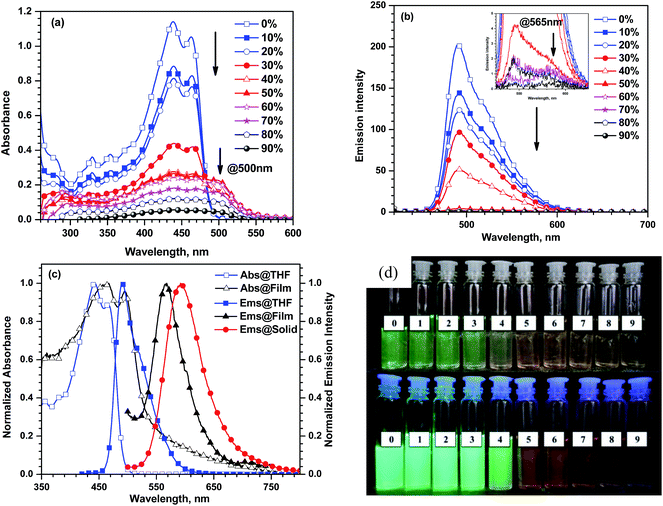 |
| Fig. 4 (a and b) Absorption and emission spectra of 3c from THF : H2O titration studies, respectively. (c) Normalized absorption and emission spectra in THF solution, thin film and solid state. (d) Images of the solutions (top: under normal light and bottom: under UV light) obtained by varying the water concentration in THF solvent for 3c (left to right – increasing water concentration 0–90%). | |
In the fluorescence spectra, the aggregate emission bathochromically shifts towards the solid state emission region. The fluorescence emission intensity in THF solution is 3–5 folds higher than that of the (1
:
1) THF
:
H2O system. Such red-shifted and quenched emission intensity at high water content (Fig. S5†) could be attributed to the presence of π–π stacking interactions that induce the self-assembly. This supports the formation of aggregates driven by non-covalent interactions, which is corroborated by the red shifted absorption and emission bands and emission quenching in the solid state.
Effect of aggregation on excited state decay dynamics
To investigate and analyze the effect of aggregation on radiative and non-radiative transition rates (kr and knr), time resolved fluorescence lifetime decay measurements were conducted for the compounds in THF solution and a 90% water–THF mixture (Fig. 5, Table 2). The fluorescence lifetime measurements display the multi-exponential behavior of emission decay, which was best fitted with a double or triple exponential function (Table S2†). According to Sillen et al. the amplitude average lifetime is an effective parameter to derive the transition rate constants of multi-exponential decays caused by the exposure of fluorescent molecules to different environments.30 The calculated average lifetime27a (τavg) of the excited state of the dyes in solution is found to be long lived compared with the corresponding aggregates. This can be correlated to the quenching of ΦF in the aggregated and solid state. Furthermore, the lifetime calculated is found to be consistent with the order of quantum yields observed in solution, aggregates and solid state of the dyes. The correlated data reveal that the long lifetime value of 3.58 ns in solution for 3d is attributed to delayed radiative rate decay, i.e., high quantum yield. Moreover, in the aggregated state the lifetime acquired is shorter, indicating a more efficient non-radiative decay pathway. This is clearly reflected by the magnitude of knr, which is significantly larger than the kr value for the aggregates. Moreover, F. C. Spano et al. theoretically studied exciton delocalization in molecular aggregates due to exciton-vibration coupling.31 Thus, on comparing the radiative decay rate of solution (0.4 ns−1) and aggregates for 3b (1 ns−1) and 3c (8 ns−1), the radiative decay rate is greatly enhanced, which is characteristic for J-aggregates and is referred to as super-radiance.32 This is clear evidence of the formation of J-aggregates in the solid state, whereas the non-radiative decay rate for the aggregation state is observed to be faster than the corresponding knr values in solution, which reflects effective intermolecular interactions that result in a significant decline in fluorescence quantum yield.
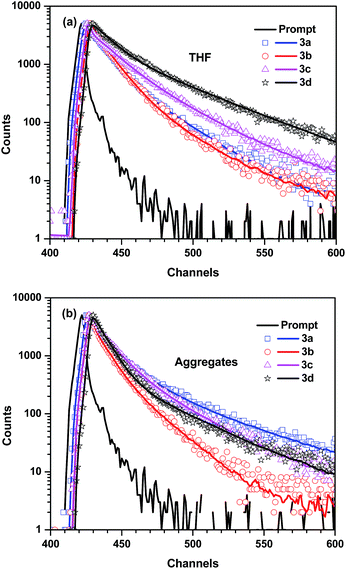 |
| Fig. 5 Fluorescence decay profiles of the dyes in (a) THF solution and (b) 90% water–THF mixture for 2 × 10−5 M aggregate solution. | |
Table 2 Time-resolved fluorescence and photophysical data for the dyes in THF and 90% water–THF mixture
Dye |
(τavga), ns |
ΦFb |
krc, ns−1 |
knrd, ns−1 |
Sol. |
Agg. |
Sol. |
Agg. |
Sol. |
Agg. |
Sol |
Agg. |
τavg = fluorescence lifetime decay measured as τavg = (A1 × τ1) + (A2 × τ2) + (A3 × τ3) in ns. Fluorescence relative quantum yield calculated using coumarin 6 as a reference (0.78 in EtOH). Radiative decay rates (kr) calculated using k = ΦF/τ. Non-radiative decay rate (knr) calculated using Φ = kr/(kr + knr). |
3a |
1.73 |
2.43 |
0.66 |
0.06 |
0.38 |
0.03 |
0.20 |
0.39 |
3b |
1.40 |
0.01 |
0.52 |
0.01 |
0.37 |
1.00 |
0.34 |
99.00 |
3c |
1.83 |
0.01 |
0.74 |
0.08 |
0.40 |
8.00 |
0.14 |
92.00 |
3d |
3.58 |
1.84 |
0.90 |
0.15 |
0.25 |
0.08 |
0.03 |
0.46 |
If we look into the calculated lifetimes of the dyes, the first lifetime (τa) is equivalent to that of the 3a lifetime and therefore can be ascribed to the emission from the naphthalimide skeleton,20j whereas the lifetime of less than a 1 ns time scale with ultrafast decay channels can be correlated to the non-emissive aggregated state of the dyes. We found it interesting to correlate this fluorescence decay analysis with the degree of assembling properties of the dyes. The long lifetime value of 1.84 ns for the aggregates of 3d as compared to 0.01 ns for the other two dyes in the 90% water–THF system reflects the better self-assembly properties and solid state quantum yield of the corresponding dye, whereas for 3a τagg is 0.7 ns longer than in solution, i.e., the monomeric state. This could be due to stronger intermolecular interactions in the excited state.18b Moreover, the longer life time in the aggregated state and micro structured flower-like morphology for this dye is possibly due to the involvement of a large number of molecules in the aggregates. Thus, the fluorescence lifetime decay data for solution and aggregates offer us sufficient evidence for the existence of molecular aggregation in the solid state.
Electrochemical properties
Electrochemical analysis of the dyes was carried out using cyclic voltammetry (CV) and differential pulse voltammetry (DPV) measurements and the results are summarized in Table 1. All the compounds exhibit a reversible redox couple attributed to the reduction of the naphthalimide unit (Fig. S6a†).20j Compound 3a exhibits a single two-electron reduction, while the other dyes show two or more reduction couples. This clearly indicates that the reduction propensity of the molecules is enhanced upon the introduction of an additional acceptor in the conjugation pathway. However, it is interesting that only the benzothiadiazole derivative 3c possessed a reduction potential larger than the dimer 3a, whereas the elongation of conjugation with the incorporation of fluorenone and dibenzo[a,c]phenazine in 3b and 3d, respectively, contributes to the decrement in the bandgap.16h,26 The benzothiadiazole derivative, 3c displays a third reduction couple, which is attributable to the reduction of the benzothiadiazole moiety.16f The LUMO energy values deduced from the reduction potentials are close to −3.3 eV, which lie among those of typical air stable n-type organic semiconductors.15c The high electron affinity across the π-conjugated backbone afforded by the two naphthalimides and an electron deficient central unit helps to deepen the HOMO energy level, which lies in the range from −5.9 eV to −6.3 eV. All the dyes exhibit an irreversible oxidation peak. It is worth mentioning that the electronic structure of the naphthalimide core is finely tuned by the introduction of electron withdrawing moieties and the energy levels of all the dyes are favorable for them to act as n-type semiconducting materials in organic light emitting diodes (OLEDs), organic solar cells (OSCs) and organic thin film transistors (OTFTs).19b,23
Theoretical studies
To understand the electronic features of the compounds, density functional theoretical computations were performed on model structures of the compounds in which the lengthy alkyl chains are replaced by a methyl group to save on computational time (Fig. 6). The geometry of the compounds was optimized using the B3LYP functional and 6-31G(d,p) basis set (Fig. S7†). The optimized structures were then used for TDDFT computations at the (M06-2x/6-31G(d,p)) level (Table S6†). The optimized structures assume a coplanar arrangement of functional entities, which ensures a close packing arrangement of molecules in the solid state.8j,18b,23d The HOMO of the molecules is mainly contributed by the π-linker and the naphthalene segment of naphthalimide, whereas the HOMO−1 orbital is mainly spread over the naphthalimide unit. In compound 3d, the dibenzo[a,c]phenazine unit also contributes to the formation of HOMO−1. The LUMO is mainly constructed by the central π-linker with little contribution from the naphthalimide units. Interestingly, the LUMO+1 is restricted to the naphthalimide unit in all the compounds. The observed electronic distributions in the molecules suggest the electron-withdrawing nature of the constituting units. The prominent contribution of the π-linker to the LUMO suggests the electron deficiency of the central bridge over the naphthalimide periphery.
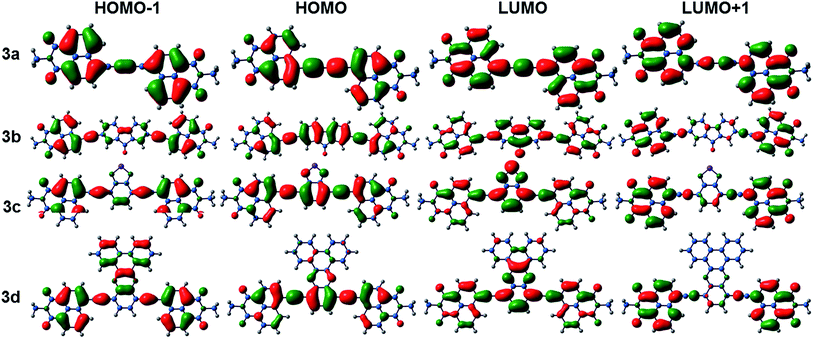 |
| Fig. 6 Electronic distribution in the frontier molecular orbitals of the model compounds 3a–3d. | |
Morphological properties
The morphologies of the as-synthesized compounds and those obtained from the solvent system were investigated via field emission scanning electron microscopy (FESEM). SEM images of the as synthesized samples (Fig. 7) reveal the presence of an ample amount of rod-like nanostructures with diameters and lengths in the range of hundreds of nanometers (nm) to several micrometers (μm), respectively, and the rods/belts have a uniform nano sized diameter along their lengths. SEM images show that the typical diameter of the nanostructures is approximately 120 ± 20 nm.
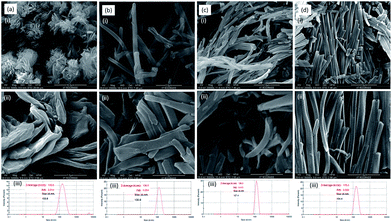 |
| Fig. 7 FESEM images of the as synthesized compounds (a–d) 3a–3d, respectively; scale bar: (a) (i) 10 μm, (ii) 1 μm; (b–c) (i) 2 μm, (ii) 1 μm and (iii) DLS-determined size distribution profiles for the aggregates formed from (a–d) 3a–3d in THF/H2O (1 : 9; 2 × 10−5 M), respectively. | |
The dyes 3b–3d possessing a skeleton derived from elongated conjugation, exhibits nanorods/belts, whereas 3a forms microflowers (6 ± 1 μm) composed of a number of nanoflakes as petals in nanodimensions. Thus, the morphological difference between these chromophores is attributed to the difference in conjugation length. Moreover, it is observed that the SEM images of compound 3d reveal the formation of well-defined nanorods compared to the others for the as synthesized compounds due to the effective intermolecular interactions arising from the more extended planar π-conjugated aromatic system. The mechanism for the formation of rods arises from the effective π–π intermolecular head to tail stacking between the molecules, whereas the flower-like morphology for 3a is formed by the arrangement of a number of nanopetals and is driven by the intermolecular interactions, which can be correlated to the results obtained from UV-Vis spectroscopy and powder XRD analysis. It is also observed that as the density of sample increases, the number of aggregates increases, as observed at the core center in the SEM images, which results in the formation of heaps of clusters. When the stacking forces between the molecules are too large, ill-defined super structural assemblies are formed. This is also confirmed by the SEM images of the compounds, as shown in Fig. S8.†
In Fig. 8, the FESEM images of the aggregates of 3c obtained from the binary THF
:
H2O solvent system at 50% and 90% H2O, which were prepared for absorption and emission studies, clearly support the formation of aggregates due to solvophobicity and π–π intermolecular interactions resulting in long-range ordering and finally induces the formation of nanostructures. Very long and thin fibrous nano to micro scale structures of several micrometer lengths are obtained for the sample in the 50% THF
:
H2O system, whereas for the 90% H2O system a bunch of nanosized rigid aggregates are observed, revealing the formation of nanorods. Moreover, after 1 week at room temperature the same sample solution gives nano to micro scale crystalline SEM images in the form of typical rods and cubes of nano dimensions due to the facile crystallization induced by the conjugated core, hydrophobicity of the alkyl chains and effective intermolecular forces between the aromatic moieties.
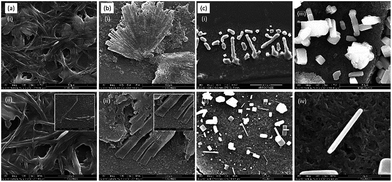 |
| Fig. 8 FESEM images of 3c (a) long entangled fibres in 50% THF : H2O; scale bar: (i) 5 μm and (ii) 2 μm; inset showing a long fibrous strand; scale bar 2 μm. (b) Formation of nanorods in 90% THF : H2O; scale bar: (i) 5 μm and (ii) 2 μm; inset showing magnified image of nanorods at the scale bar of 1 μm. (c) (i–iv) Formation of crystalline 3-D rods and cubes at different scale bars: 50, 10, 5, and 1 μm respectively from 90% THF : H2O after 1 week. | |
Furthermore, the fine and uniform nanostructures obtained for compound 3d allow us to investigate the SEM images of the drop-casted precipitates obtained from DMF solution at the low concentration of 1 mM, which give spindle shaped thin and long fibers of diameter 120 ± 10 nm and length of approximately 10 μm throughout the sample area under investigation. Moreover, the presence of strong intermolecular interactions and formation of thin fibrous network-like assemblies motivated us to organogelate the compound.13f,14e,18b,33 Therefore, a supersaturated sample of 1 wt% (16 mM) DMF solution concentration was prepared via the heating–cooling method; however, due to the crystalline nature of the compound it reprecipitated out during the cooling process. SEM images of the reprecipitated crystalline compound show uniform sized cubic crystals of approximately 1 × 1 μm2 throughout the glass plate, which assemble to form large crystals in the form of cuboids and rods, and is driven by the minimization of the surface area, as shown in Fig. 9a and b. We tried to grow crystals of the dyes in a number of solvent systems for single crystal X-ray diffraction studies but were unsuccessful in obtaining crystals that could diffract, and instead ended up with fibers or a mosaic. On the other hand, 3a (Fig. 9c and d) exhibits clusters of nanoflakes as heaps in 1 mM DMF, while it precipitates out as supramolecular sheets and flowers from the 1 wt% DMF solution system. These morphological changes are due to the concentration dependence of self-aggregation.
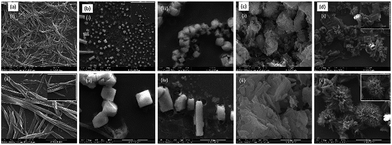 |
| Fig. 9 FESEM images of (a) 3d from 1 mM DMF showing spindle shaped nanofibers; scale bar (i) 10 μm and (ii) 2 μm, inset 1 μm. (b) (i–iv) 3d crystalline 3-D structures from 1 wt% DMF. (c) 3a from 1 mM DMF; scale bar (i) 10 μm and (ii) 2 μm. (d) Precipitates of 3a from 1 wt% DMF as sheets and flowers; scale bar (i) 100 μm, inset 50 μm and (ii) 50 μm, inset 20 μm. | |
Thus, it can be stated that the facile tunable morphology at the nanoscale of the planar and rigid end-capped naphthalimide based organic n-type semiconductors is of great importance for a large number of efficient device applications.
Dynamic light scattering analysis
Dynamic light scattering (DLS) was used to determine the particle size distribution of the aggregates of the dyes in THF
:
H2O mixtures (2 × 10−5 M) with water fractions (fw) of 90%, which indicates a homogenous dispersion (Fig. 3, iii). The median diameter (dm) and average diameter (davg) for the aggregates was found to be in range of ∼125–155 nm and >200 nm, respectively (Table S3†), which is in good agreement with the SEM data. The particle size distribution peak for 3b–3d is narrower than 3a, suggesting a well-ordered particle distribution.34 Bimodal distributions are observed for 3a, whereas the others exhibited unimodal distributions. The additional less intense peak observed for the 3a dispersion centered at ∼6 μm is attributed to the diameter of the flower like morphology, as observed in the FESEM images.
Fluorescence microscopy analysis
Furthermore, fluorescence images of the dyes (Fig. S9†) were obtained using optical fluorescence microscopy, exhibiting multicolor emission35 under different light sources due to the broad emission region. The drop-casted film obtained from dichloromethane solution (1 mM) shows bright yellow-green and orange-red fluorescence upon excitation with blue and green light, respectively. Therefore, the multicolor emission properties of these small molecules are useful for the design and fabrication of displays and flat screens.
Powder X-ray diffraction analysis
To investigate the molecular arrangement in the solid state, powder X-ray diffraction (PXRD) was carried out, which is depicted in Fig. 10 and the calculated d-spacing values are summarized in Table S4.† The pattern obtained clearly shows intensive and sharp peaks within the range of 2θ = 5° to <50°, suggesting a well-ordered crystalline structure. The intermolecular distance calculated from the peak positions is found to be effective for π–π intermolecular interactions.2g,19h The sharp diffraction peak at 2θ = ∼25° corresponds to the d-spacing of 3.49 Å, 3.43 Å, 3.55 Å and 3.49 Å for 3a–3d, respectively, and can be attributed to the π–π stacking intermolecular distance responsible for the self-assembling, whereas the sharp diffraction peak at 2θ = ∼6° analogous to the d-spacing of ∼13 Å can be attributed to the translational slip stacking of the small molecules into J-aggregates, as reported in earlier reports.16i,23f Therefore, we conclude that the pattern obtained reflects an organized assembly and crystallinity of these π-conjugated molecules in the solid state, which are suitable for excellent charge transportation by an enhanced intermolecular transfer rate.
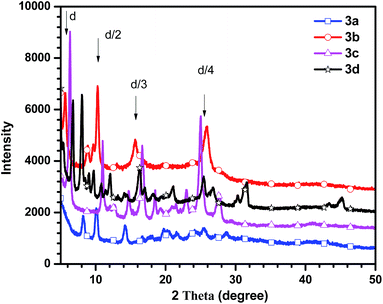 |
| Fig. 10 PXRD of the small molecules (3a–3d). | |
Thermal properties
The thermal stability of materials based on nanostructures is of paramount importance for the construction of electronic devices to withstand high temperatures. Therefore, we investigated the melting temperature (Tm) and decomposition temperatures (Td) of the compounds via differential scanning calorimetry (DSC) and thermo-gravimetric analysis (TGA). The relevant traces are shown in Fig. 11 and the data is summarized in Table S5.† The endothermic sharp peak in the DSC plots represents Tm, which increases in the order of 3a < 3c < 3d < 3b and points to the increasing trend of molecular interactions and presence of some degree of molecular ordering within the molecules, which are favorable for charge transportation, whereas the broad exothermic peak above 300 °C corresponds to the weight loss of the compound because of the deimidization process. Furthermore, the crystalline behavior of compounds is proven by the absence of a glass transition temperature (Tg).23a,23f Their excellent thermal stability is confirmed from the two-step decomposition pathway with Td at 10% weight loss above 400 °C. The weight loss in the first step between 400 and 550 °C may be due to the degradation of the alkyl chains and other labile units, whereas the second step between 500 and 700 °C corresponds to the loss of aromatic residue and other stronger bonds. Thus, the thermal analysis clearly reflects both the crystalline behavior and high thermal stability of the non-covalent interactions based self-assembled small molecules.
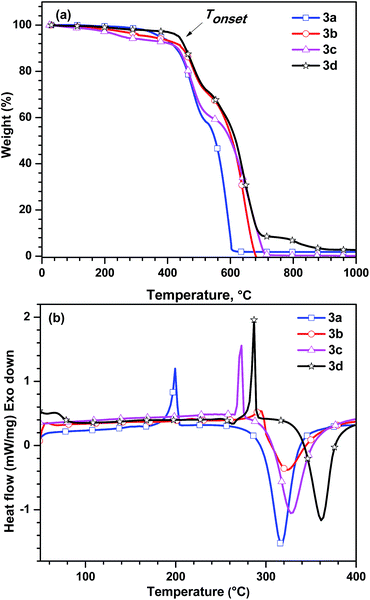 |
| Fig. 11 (a) TGA and (b) DSC curves of the compounds. | |
Conclusions
In summary, we shed light on the self-assembly properties of rigid, linear and highly electron deficient π-conjugated naphthalimide derivatives synthesized via the Sonogashira coupling reaction. The solution, thin film and solid state optical properties of the dyes support the presence of intermolecular interactions and π–π stacking, which enhance charge transport properties. This is further supported by the powder XRD analysis of the as synthesized samples. Fluorescence lifetime decay measurements highlight the effect of aggregation on the decay dynamics of the excited state. Furthermore, the thermal and electrochemical data indicate high thermal stability, crystallinity and suitable energetics, which support the high electron deficiency of the molecules with high electron affinity and deep HOMO energy levels that make the molecules suitable for use as electron accepting semiconductors. Density functional theoretical investigations highlight the electronic properties and ensure the coplanar geometries of the compounds. The morphology patterns observed in the FESEM images of these organic molecules confirm π–π stacking interactions and establish the well-defined formation of nanostructures with rod shaped or flower like morphologies in the solid state. It is also found that the morphology varies with concentration and solvent. DLS analysis further strengthens the formation of the nanomorphology for the aggregates. Therefore, it is possible to use varying aromatic units that exhibit planarity and rigidity as a tool to control the molecular packing, orientation, and intermolecular spacing of aromatics in the solid state. Judiciously and efficiently tuning the properties of such class of nano-structural materials could be beneficial for n-type organic semiconducting materials to study electronic transport properties in a number of solution processed nano-optoelectronic devices. Application of these small molecules as emitters and electron transporting layers in OLEDs and non-fullerene acceptors in OSCs is ongoing work in our laboratory.
Acknowledgements
KRJT is thankful to CSIR (Ref. No. 02/(0230)/15/EMR-II dated 05-06-2015), New Delhi for generous financial support. AS acknowledges a research fellowship from Ministry of Human Resources and Development (MHRD), Government of India. We are also thankful to DST for the purchase of ESI mass spectrometer via the FIST grant to the Chemistry Department, IIT Roorkee.
Notes and references
-
(a) W. Yao and Y. S. Zhao, Nanoscale, 2014, 6, 3467 RSC;
(b) Y. Lin, Y. Li and X. Zhan, Chem. Soc. Rev., 2012, 41, 4245 RSC;
(c) J. L. Atwood and J. W. Steed, Organic Nanostructures, Wiley, New York, 2008 Search PubMed;
(d) L. Magginia and D. Bonifazi, Chem. Soc. Rev., 2012, 41, 211 RSC;
(e) B. K. An, S. H. Gihm, J. W. Chung, C. R. Park, S. K. Kwon and S. Y. Park, J. Am. Chem. Soc., 2009, 131, 3950 CrossRef CAS PubMed;
(f) Y. S. Zhao, H. B. Fu, F. Q. Hu, A. D. Peng, W. S. Yang and J. N. Yao, Adv. Mater., 2008, 20, 79 CrossRef CAS;
(g) P. S. Weiss, Acc. Chem. Res., 2008, 41, 1772 CrossRef CAS PubMed;
(h) F. Würthner, Supramolecular Dye Chemistry, Topics in Current Chemistry, Springer, Berlin, 2005, p. 258 Search PubMed;
(i) S. I. Stupp, Chem. Rev., 2005, 105, 1023 CrossRef CAS;
(j) D. B. Xiao, X. Lu, W. S. Yang, H. B. Fu, Z. G. Shuai, Y. Fang and J. N. Yao, J. Am. Chem. Soc., 2003, 125, 6740 CrossRef CAS PubMed.
-
(a) Q. Yan, Z. Luo, K. Cai, Y. Ma and D. Zhao, Chem. Soc. Rev., 2014, 43, 4199 RSC;
(b) M. R. Molla and S. Ghosh, Phys. Chem. Chem. Phys., 2014, 16, 26672 RSC;
(c) A. Das and S. Ghosh, Angew. Chem., Int. Ed., 2014, 53, 2038 CrossRef CAS PubMed;
(d) A. C. Mendes, E. T. Baran, R. L. Reis and H. S. Azevedo, Wiley Interdiscip. Rev.: Nanomed. Nanobiotechnol., 2013, 5, 582 CrossRef CAS PubMed;
(e) B. Rybtchinski, ACS Nano, 2011, 5, 6791 CrossRef CAS PubMed;
(f) D. G. Rodríguez and A. P. H. J. Schenning, Chem. Mater., 2011, 23, 310 CrossRef;
(g) T. Nakanishi, Chem. Commun., 2010, 46, 3425 RSC;
(h) S. Yagai and A. Kitamura, Chem. Soc. Rev., 2008, 37, 1520 RSC;
(i) J. W. Steed, D. R. Turner and K. J. Wallace, Core concepts in supramolecular chemistry and nanochemistry, Wiley, 2007 Search PubMed;
(j) F. J. M. Hoeben, P. Jonkheijm, E. W. Meijer and A. P. H. J. Schenning, Chem. Rev., 2005, 105, 1491 CrossRef CAS PubMed;
(k) C. P. Poole, Introduction to nanotechnology, John Wiley & Sons, Hoboken, New Jersey, USA, 2003 Search PubMed;
(l) R. K. Castellano and J. Rebek, J. Am. Chem. Soc., 1998, 120, 3657 CrossRef CAS;
(m) M. Li, H. Schnablegger and S. Mann, Nature, 1999, 402, 393 CrossRef CAS;
(n) J.-M. Lehn, Supramolecular Chemistry: Concepts and Perspectives, Wiley, New York, 1995 Search PubMed.
-
(a) Y. S. Zhao, Organic Nanophotonics: Fundamentals and Applications, Wiley, New York, 2014 Search PubMed;
(b) B. Z. Tian, J. Liu, T. Dvir, L. H. Jin, J. H. Tsui, Q. Qing, Z. G. Suo, R. Langer, D. S. Kohane and C. M. Lieber, Nat. Mater., 2012, 11, 986 CrossRef CAS PubMed;
(c) C. Zhang, Y. S. Zhao and J. Yao, Phys. Chem. Chem. Phys., 2011, 13, 9060 RSC;
(d) H. Zheng, Y. Li, H. Liu, X. Yin and Y. Li, Chem. Soc. Rev., 2011, 40, 4506 RSC;
(e) F. S. Kim, G. Ren and S. A. Jenekhe, Chem. Mater., 2011, 23, 682 CrossRef CAS;
(f) Y. Zhao, H. Fu, A. Peng, Y. Ma, Q. Liao and J. Yao, Acc. Chem. Res., 2010, 43, 409 CrossRef CAS PubMed;
(g) H. Liu, J. Xu, Y. Li and Y. Li, Acc. Chem. Res., 2010, 43, 1496 CrossRef CAS PubMed;
(h) A. Mishra, C.-Q. Ma and P. Bauerle, Chem. Rev., 2009, 109, 1141 CrossRef CAS PubMed;
(i) L. Zang, Y. Che and J. F. Moore, Acc. Chem. Res., 2008, 41, 1596 CrossRef CAS PubMed.
-
(a) Y. Li, T. Liu, H. Liu, M. Z. Tian and Y. Li, Acc. Chem. Res., 2014, 47, 1186 CrossRef CAS PubMed;
(b) R. J. Li, W. P. Hu, Y. Q. Liu and D. B. Zhu, Acc. Chem. Res., 2010, 43, 529 CrossRef CAS PubMed;
(c) Z. Chen, A. Lohr, C. R. Saha-Möller and F. Würthner, Chem. Soc. Rev., 2009, 38, 564 RSC;
(d) J. Lv, H. B. Liu and Y. L. Li, Pure Appl. Chem., 2008, 80, 639 CrossRef CAS;
(e) L. Zang, Y. Che and J. S. Moore, Acc. Chem. Res., 2008, 41, 1596 CrossRef CAS PubMed;
(f) C. Li, T. Hatano, M. Takeuchi and S. Shinkai, Chem. Commun., 2004, 20, 2350 RSC.
-
(a) C. Sutton, C. Risko and J.-L. Bredas, Chem. Mater., 2016, 28, 3 CrossRef CAS;
(b) S. Mukherjee and P. Thilagar, Chem.–Eur. J., 2014, 20, 8012 CrossRef CAS PubMed;
(c) S. Ehrlich, J. Moellmann and S. Grimme, Acc. Chem. Res., 2012, 46, 916 CrossRef PubMed;
(d) L. C. Palmer and S. I. Stupp, Acc. Chem. Res., 2008, 41, 1674 CrossRef CAS PubMed;
(e) A. C. Grimsdale and K. Müllen, Angew. Chem., Int. Ed., 2005, 44, 5592 CrossRef CAS PubMed;
(f) F. Würthner, Chem. Commun., 2004, 14, 1564 RSC;
(g) T. Q. Nguyen, R. Martel, P. Avouris, M. L. Bushey, L. Brus and C. Nuckolls, J. Am. Chem. Soc., 2004, 126, 5234 CrossRef CAS PubMed.
-
(a) E. E. Jelley, Nature, 1936, 138, 1009 CrossRef CAS;
(b) G. Scheibe, Angew. Chem., 1937, 50, 212 CrossRef CAS.
- F. Würthner, C. Thalacker, S. Diele and C. Tschierske, Chem.–Eur. J., 2001, 7, 2245 CrossRef.
-
(a) R. Hu, J. W. Y. Lam, H. Deng, Z. Song, C. Zheng and B. Z. Tang, J. Mater. Chem. C, 2014, 2, 6326 RSC;
(b) L. Chen, C. Li and K. Müllen, J. Mater. Chem. C, 2014, 2, 1938 RSC;
(c) M. R. Rao and S.-S. Sun, Langmuir, 2013, 29, 15146 CrossRef CAS PubMed;
(d) B. K. An, J. Gierschner and S. Y. Park, Acc. Chem. Res., 2012, 45, 544 CrossRef CAS PubMed;
(e) D. G. Rodríguez and A. P. H. J. Schenning, Chem. Mater., 2011, 23, 310 CrossRef;
(f) N. Sakai, J. Mareda, E. Vauthey and S. Matile, Chem. Commun., 2010, 46, 4237 Search PubMed;
(g) Z. Chen, A. Lohr, C. R. Saha-Mçller and F. Würthner, Chem. Soc. Rev., 2009, 38, 564 RSC;
(h) A. Mishra, C.-Q. Ma and P. Bauerle, Chem. Rev., 2009, 109, 1141 CrossRef CAS PubMed;
(i) A. Ajayaghosh and V. K. Praveen, Acc. Chem. Res., 2007, 40, 644 CrossRef CAS PubMed;
(j) F. J. M. Hoeben, P. Jonkheijm, E. W. Meijer and A. P. H. J. Schenning, Chem. Rev., 2005, 105, 1491 CrossRef CAS PubMed.
-
(a) J. E. Park, M. Son, M. Hong, G. Lee and H. C. Choi, Angew. Chem., Int. Ed., 2012, 51, 6383 CrossRef CAS PubMed;
(b) T. Jiu, Y. Li, H. Liu, J. Ye, X. Liu, L. Jiang, M. Yuan, J. Li, C. Li, S. Wang and D. Zhu, Tetrahedron, 2007, 63, 3168 CrossRef CAS;
(c) S. Yagai, Y. Goto, X. Lin, T. Karatsu, A. Kitamura, D. Kuzuhara, H. Yamada, Y. Kikkawa, A. Saeki and S. Seki, Angew. Chem., Int. Ed., 2012, 51, 6643 CrossRef CAS PubMed.
-
(a) J. Zhu, K. Zhong, Y. Liang, Z. Wang, T. Chen and L. Y. Jin, Tetrahedron, 2014, 70, 1230 CrossRef CAS;
(b) C. Wang, Y. Liu, Z. Ji, E. Wang, R. Li, H. Jiang, Q. Tang, H. Li and W. Hu, Chem. Mater., 2009, 21, 2840–2845 CrossRef CAS;
(c) Y. S. Zhao, J. J. Xu, A. D. Peng, H. B. Fu, Y. Ma, L. Jiang and J. N. Yao, Angew. Chem., Int. Ed., 2008, 47, 7301–7305 CrossRef CAS PubMed.
-
(a) J. Xiao, X. Xiao, Y. Zhao, B. Wu, Z. Liu, X. Zhang, S. Wang, X. Zhao, L. Liu and L. Jiang, Nanoscale, 2013, 5, 5420 RSC;
(b) Y. Sagara, T. Mutai, I. Yoshikawa and K. Araki, J. Am. Chem. Soc., 2007, 129, 1520 CrossRef CAS PubMed;
(c) X. J. Zhang, X. H. Zhang, W. S. Shi, X. M. Meng, C. Lee and S. Lee, J. Phys. Chem. B, 2005, 109, 18777 CrossRef CAS PubMed.
-
(a) Y. Yan, C. Zhang, J. Yao and Y. S. Zhao, Adv. Mater., 2013, 25, 3627 CrossRef CAS PubMed;
(b) Y. L. Lei, Q. Liao, H. B. Fu and J. N. Yao, J. Phys. Chem. C, 2009, 113, 10038 CrossRef CAS;
(c) C. Ego, D. Marsitzky, S. Becker, J. Zhang, A. Grimsdale, K. Müllen, J. MacKenzie, C. Silva and R. Friend, J. Am. Chem. Soc., 2003, 125, 437 CrossRef CAS PubMed.
-
(a) F. Würthner, C. R. Saha-Möller, B. Fimmel, S. Ogi, P. Leowanawat and D. Schmidt, Chem. Rev., 2015, 116, 962 CrossRef PubMed;
(b) S. Chen, P. Slattum, C. Wang and L. Zang, Chem. Rev., 2015, 115, 11967 CrossRef CAS PubMed;
(c) L. Xu, D. Gao, J. Song, L. Shen, W. Chen, Y. Chen and S. Zhang, New J. Chem., 2015, 39, 5553 RSC;
(d) C. Li and H. Wonneberger, Adv. Mater., 2012, 24, 613 CrossRef CAS PubMed;
(e) D. Görl, X. Zhang and F. Würthner, Angew. Chem., Int. Ed., 2012, 51, 6328 CrossRef PubMed;
(f) J. Lambrecht, T. P. I. Saragi and J. Salbeck, J. Mater. Chem., 2011, 21, 18266 RSC;
(g) K. Balakrishnan, A. Datar, R. Oitker, H. Chen, J. M. Zuo and L. Zang, J. Am. Chem. Soc., 2005, 127, 10496 CrossRef CAS PubMed;
(h) G. D. Sharma, M. S. Roy, J. A. Mikroyannidis and K. R. J. Thomas, Org. Electron., 2012, 13, 3118 CrossRef CAS;
(i) A. S. Sayyad, K. Balakrishnan and P. M. Ajayan, Nanoscale, 2011, 3, 3605 RSC;
(j) K. Balakrishnan, A. Datar, T. Naddo, J. L. Huang, R. Oitker, M. Yen, J. C. Zhao and L. Zang, J. Am. Chem. Soc., 2006, 128, 7390 CrossRef CAS PubMed;
(k) P. Yan, A. Chowdhury, M. W. Holman and D. M. Adams, J. Phys. Chem. B, 2005, 109, 724 CrossRef CAS PubMed;
(l) X.-Q. Li, V. Stepanenko, Z. Chen, P. Prins, L. D. A. Siebbeles and F. Würthner, Chem. Commun., 2006, 3871 RSC;
(m) R. Dobrawa and F. Würthner, Chem. Commun., 2002, 1878 RSC;
(n) F. Würthner, A. Sautter and J. Schilling, J. Org. Chem., 2002, 67, 3037 CrossRef;
(o) G. R. J. Müller, C. Meiners, V. Enkelmann, Y. Greerts and K. Müllen, J. Mater. Chem., 1998, 8, 61 RSC.
-
(a) M. Mastalerz, E. Menke, Y. Vaynzof and V. Lami, Chem. Commun., 2016, 52, 1048 RSC;
(b) H. Kar, D. W. Gehrig, F. Laquai and S. Ghosh, Nanoscale, 2015, 7, 6729 RSC;
(c) R. Fernando, Z. Mao and G. Sauve, Org. Electron., 2013, 14, 1683 CrossRef CAS;
(d) E. Ahmed, G. Ren, F. S. Kim, E. C. Hollenbeck and S. A. Jenekhe, Chem. Mater., 2011, 23, 4563 CrossRef CAS;
(e) S. Basak, N. Nandi, A. Baral and A. Banerjee, Chem. Commun., 2015, 51, 780 RSC.
-
(a) Z. Liu, G. Zhang, Z. Cai, X. Chen, H. Luo, Y. Li, J. Wang and D. Zhang, Adv. Mater., 2014, 26, 6965 CrossRef CAS PubMed;
(b) Y. Li, P. Sonar, L. Murphy and W. Hong, Energy Environ. Sci., 2013, 6, 1684 RSC;
(c) H. Usta, A. Facchetti and T. Marks, Acc. Chem. Res., 2011, 44, 501 CrossRef CAS PubMed;
(d) X. Zhan, A. Facchetti, S. Barlow, T. Marks, M. Ratner, M. Wasielewski and S. Marder, Adv. Mater., 2011, 23, 268 CrossRef CAS PubMed;
(e) C. B. Nielsen, M. Turbiez and I. McCulloch, Adv. Mater., 2013, 25, 1859 CrossRef CAS PubMed;
(f) Y. Zhao, Y. Guo and Y. Liu, Adv. Mater., 2013, 25, 5372 CrossRef CAS PubMed;
(g) Y. Li, P. Sonar, L. Murphy and W. Hong, Energy Environ. Sci., 2013, 6, 1684 RSC.
-
(a) W. Yao, Y. Yan, L. Xue, C. Zhang, G. Li, Q. Zheng, Y. S. Zhao, H. Jiang and J. Yao, Angew. Chem., Int. Ed., 2013, 52, 8713 CrossRef CAS PubMed;
(b) N. Chandrasekhar and R. Chandrasekar, Angew. Chem., Int. Ed., 2012, 51, 3556 CrossRef CAS PubMed;
(c) Y. Wang, H. Fu, A. Peng, Y. Zhao, J. Ma, Y. Ma and J. Yao, Chem. Commun., 2007, 1623 RSC;
(d) Z. Tian, Y. Chen, W. Yang, J. Yao, L. Zhu and Z. Shuai, Angew. Chem., Int. Ed., 2004, 43, 4060 CrossRef CAS PubMed;
(e) S. Chen, N. Chen, Y. Yan, T. Liu, Y. Yu, Y. Li, H. Liu, Y. Zhao and Y. Li, Chem. Commun., 2012, 48, 9011 RSC;
(f) S. Chen, Y. Li, W. Yang, N. Chen, H. Liu and Y. Li, J. Phys. Chem. C, 2010, 114, 15109 CrossRef CAS;
(g) Y. Wang, J. L. Liu, H. D. Tran, M. Mecklenburg, X. N. Guan, A. Z. Stieg, B. C. Regan, D. C. Martin and R. B. Kaner, J. Am. Chem. Soc., 2012, 134, 9251 CrossRef CAS PubMed;
(h) J. Xu, S. Semin, D. Niedzialek, P. H. J. E. Kouwer, E. Fron, E. Coutino, M. Savoini, Y. Li, J. Hofkens, H.-I. Uji, D. Beljonne, T. Rasing and A. E. Rowan, Adv. Mater., 2013, 25, 2084 CrossRef CAS PubMed;
(i) A. Ghodbane, S. D'Altério, N. Saffon, N. D. McClenaghan, L. Scarpantonio, P. Jolinat and S. F. Forgues, Langmuir, 2012, 28, 855 CrossRef CAS PubMed;
(j) S. Sampath, A. A. Boopathi and A. B. Mandal, Phys. Chem. Chem. Phys., 2016 10.1039/c6cp02662a.
-
(a) D. Gudeika, J. V. Grazulevicius, D. Volyniuk, G. Juska, V. Jankauskas and G. Sini, J. Phys. Chem. C, 2015, 119, 28335 CrossRef CAS;
(b) P. Gautam, R. Maragani, S. M. Mobin and R. Misra, RSC Adv., 2014, 4, 52526 RSC;
(c) K. R. J. Thomas, N. Kapoor, M. N. K. P. Bolisetty, J.-H. Jou, Y.-L. Chen and Y.-C. Jou, J. Org. Chem., 2012, 77, 3921 CrossRef CAS PubMed;
(d) A. L. Thompson, T. S. Ahn, K. R. J. Thomas, S. Thayumanavan, T. J. Martinez and C. J. Bardeen, J. Am. Chem. Soc., 2005, 127, 16348 CrossRef CAS PubMed;
(e) J. T. Bloking, X. Han, A. T. Higgs, J. P. Kastrop, L. Pandey, J. E. Norton, C. Risko, C. E. Chen, J. L. Brédas, M. D. McGehee and A. Sellinger, Chem. Mater., 2011, 23, 5484 CrossRef CAS.
-
(a) P. Josse, C. Dalinot, Y. Jiang, S. Dabos, J. Roncali, P. Blanchard and C. Cabanetos, J. Mater. Chem. A, 2016, 4, 250 RSC;
(b) X. Cao, L. Meng, Y. Mao, H. Lan, L. Chen, Y. Fan and T. Yi, Langmuir, 2014, 30, 11753 CrossRef CAS PubMed;
(c) M. Kivala and F. Diederich, Acc. Chem. Res., 2009, 42, 235 CrossRef CAS PubMed.
-
(a) A. F. Furcate, T. Higashino, D. Lorcy and T. Mori, J. Mater. Chem. C, 2015, 3, 3569 RSC;
(b) H. Usta, A. Facchetti and T. J. Marks, Acc. Chem. Res., 2011, 44, 501 CrossRef CAS PubMed;
(c) X. Gao and Y. Hu, J. Mater. Chem. C, 2014, 2, 3099 RSC;
(d) A. Lv, S. R. Puniredd, J. Zhang, Z. Li, H. Zhu, W. Jiang, H. Dong, Y. He, L. Jiang, Y. Li, W. Pisula, Q. Meng, W. Hu and Z. Wang, Adv. Mater., 2012, 24, 2626 CrossRef CAS PubMed;
(e) L. Tan, Y. Guo, G. Zhang, Y. Yang, D. Zhang, G. Yu, W. Xu and Y. Liu, J. Mater. Chem., 2011, 21, 18042 RSC;
(f) Y. Hu, X. Gao, C. Di, X. Yang, F. Zhang, Y. Liu, H. Li and D. Zhu, Chem. Mater., 2011, 23, 1204 CrossRef CAS;
(g) T. Kono, D. Kumaki, J. Nishida, S. Tokito and Y. Yamashita, Chem. Commun., 2010, 46, 3265 RSC;
(h) H. Wada, K. Shibata, Y. Bando and T. Mori, J. Mater. Chem., 2008, 18, 4165 RSC.
-
(a) C. Peebles, C. D. Wight and B. Iverson, J. Mater. Chem. C, 2015, 3, 12156 RSC;
(b) A. R. Mallia, P. S. Salini and M. Hariharan, J. Am. Chem. Soc., 2015, 137, 15604 CrossRef CAS PubMed;
(c) Q. Zhang, H. Zhuang, J. He, S. Xia, H. Li, N. Li, Q. Xu and J. Lu, J. Mater. Chem. C, 2015, 3, 6778 RSC;
(d) T. Inari, M. Yamano, A. Hirano, K. Sugawa and J. Otsuki, J. Phys. Chem. A, 2014, 118, 5178 CrossRef CAS PubMed;
(e) S. Mukherjee and P. Thilagar, Phys. Chem. Chem. Phys., 2014, 16, 20866 RSC;
(f) B. Ventura, A. Bertocco, D. Braga, L. Catalano, S. d'Agostino, F. Grepioni and P. Taddei, J. Phys. Chem. C, 2014, 118, 18646 CrossRef CAS;
(g) H. Herrera, P. de Echegaray, M. Urdanpilleta, M. J. Mancheño, E. Mena-Osteritz, P. Bäuerle and J. L. Segura, Chem. Commun., 2013, 49, 713 RSC;
(h) D. L. Reger, A. Debreczeni, J. J. Horger and M. D. Smith, Cryst. Growth Des., 2011, 11, 4068 CrossRef CAS;
(i) P. Kucheryavy, G. Li, S. Vyas, C. Hadad and K. D. Glusac, J. Phys. Chem. A, 2009, 113, 6453 CrossRef CAS PubMed;
(j) M. S. Alexiou, V. Tychopoulos, S. Ghorbanian, J. H. P. Tyman, R. G. Brown and P. I. Brittain, J. Chem. Soc. Perkin Trans. 2, 1990, 837 RSC;
(k) S. Jinnai, Y. Le, M. Karakawa, T. Aernouts, Y. Nakajima, S. Mori and Y. Aso, Chem. Mater., 2016, 28, 1705 CrossRef CAS;
(l) P. Gautam, R. Misra, S. Biswas and G. D. Sharma, Phys. Chem. Chem. Phys., 2016, 18, 13918–13926 RSC;
(m) J. Zhang, H. Xiao, X. Zhang, Y. Wu, G. Li, C. Li, X. Chen, W. Ma and J. Bo, J. Mater. Chem. C, 2016, 4, 5656–5663 RSC.
-
(a) G. Chen, J. Song, H. Zhang, Y. Jiang, W. Liu, W. Zhang and B. Wang, Nanoscale, 2015, 7, 14393 RSC;
(b) S. Banerjee, E. B. Veale, C. M. Phelan, S. A. Murphy, G. M. Tocci, L. J. Gillespie, D. O. Frimannsson, J. M. Kelly and T. Gunnlaugsson, Chem. Soc. Rev., 2013, 42, 1601 RSC;
(c) M. H. Lee, J. Y. Kim, J. H. Han, S. Bhuniya, J. L. Sessler, C. Kang and J. S. Kim, J. Am. Chem. Soc., 2012, 134, 12668 CrossRef CAS PubMed;
(d) L. Ingrassia, F. Lefranc, R. Kiss and T. Mijatovic, Curr. Med. Chem., 2009, 16, 1192 CrossRef CAS PubMed.
-
(a) M. Kumar, N. Kumar and V. Bhalla, Chem. Commun., 2013, 49, 877 RSC;
(b) R. M. Duke, E. B. Veale, F. M. Pfeffer, P. E. Krugerc and T. Gunnlaugsson, Chem. Soc. Rev., 2010, 39, 3936 RSC.
-
(a) J. Zhang, X. Zhang, G. Li, H. Xiao, W. Li, S. Xie, C. Li and Z. Bo, Chem. Commun., 2016, 52, 469 RSC;
(b) X. Zhang, J. Zhang, H. Lu, J. Wu, G. Li, C. Li, S. Li and Z. Bo, J. Mater. Chem. C, 2015, 3, 6979 RSC;
(c) P. Gopikrishna, D. Das and P. K. Iyer, J. Mater. Chem. C, 2015, 3, 9318 RSC;
(d) H.-C. Wu, J. Zhang, Z. Bo and W.-C. Chen, Chem. Commun., 2015, 51, 14179 RSC;
(e) R.-Q. Lu, Y. Q. Zheng, Y. N. Zhou, X. Y. Yan, T. Lu, K. Shi, Y. Zhou, J. Pei, L. Zoopi, K. K. Baldridge, J. S. Seigel and X. Y. Cao, J. Mater. Chem. A, 2014, 2, 20515 RSC;
(f) G. Wang, S. Miao, Q. Zhang, H. Liu, H. Li, N. Li, Q. Xu, J. Lu and L. Wang, Chem. Commun., 2013, 49, 9470 RSC;
(g) O. K. Kwon, M. A. Uddin, J.-H. Park, S. K. Park, T. L. Nguyen, H. Y. Woo and S. Y. Park, Adv. Mater., 2016, 28, 910–916 CrossRef CAS PubMed;
(h) J. Zhang, X. Zhang, H. Xiao, G. Li, Y. Liu, C. Li, H. Huang, X. Chen and Z. Bo, ACS Appl. Mater. Interfaces, 2016, 8, 5475 CrossRef CAS PubMed.
- H. G. Rule and S. B. Thompson, J. Chem. Soc., 1937, 1764 RSC.
-
(a) P. Singh, A. Baheti and K. R. J. Thomas, J. Org. Chem., 2011, 76, 6134 CrossRef CAS PubMed;
(b) E. K. Unver, S. Tarkuc, Y. A. Udum, C. Tanyeli and L. Toppare, J. Polym. Sci., Part A: Polym. Chem., 2010, 48, 1714 CrossRef CAS;
(c) X. Zhang, J.-B. Han, P.-F. Li, X. Ji and Z. Zhang, Synth. Commun., 2009, 39, 3804 CrossRef CAS;
(d) K. R. J. Thomas, M. Velusamy, J. T. Lin, C.-H. Chuen and Y.-T. Tao, Chem. Mater., 2005, 17, 1860 CrossRef CAS;
(e) K. Pilgram, M. Zupan and R. Skiles, J. Heterocycl. Chem., 1970, 7, 629 CrossRef CAS.
-
(a) J. Shi, J. Chen, Z. Chai, H. Wang, R. Tang, K. Fan, M. Wu, H. Han, J. Qin, T. Peng, Q. Li and Z. Li, J. Mater. Chem., 2012, 22, 18830 RSC;
(b) L. A. Estrada and D. C. Neckers, Org. Lett., 2011, 13, 3304 CrossRef CAS PubMed.
-
(a) J. R. Lakowicz, Principles of Fluorescence Spectroscopy, Springer Science + Business Media, New York, 3rd edn, 2006 Search PubMed;
(b) H.-T. Feng, J.-B. Xiong, Y.-S. Zheng, B. Pan, C. Zhang, L. Wang and Y. Xie, Chem. Mater., 2015, 27, 7812 CrossRef CAS.
-
(a) Z. Chen, U. Baumeister, C. Tschierske and F. Würthner, Chem.–Eur. J., 2007, 13, 450 CrossRef CAS PubMed;
(b) K. Balakrishnan, A. Datar, R. Oitker, H. Chen, J. Zuo and L. Zang, J. Am. Chem. Soc., 2005, 127, 10496 CrossRef CAS PubMed.
-
(a) B. M. Krasovitskii and B. M. Bolotin, Organic Luminescent Materials, VCH: Weinheim, Germany, 2002 Search PubMed;
(b) E. A. Silinsh, Organic Molecular Crystals, Springer-Verlag, Berlin, 1980 Search PubMed;
(c) J. B. Birks, Photophysics of Aromatic Molecules, Wiley, London, 1970 Search PubMed.
- A. Sillen and Y. Engelborghs, Photochem. Photobiol., 1998, 67, 475 CAS.
- F. C. Spano, J. Am. Chem. Soc., 2009, 131, 4267 CrossRef CAS PubMed.
-
(a) F. Würthner, T. E. Kaiser and C. R. Saha-Müller, Angew. Chem., Int. Ed., 2011, 50, 3376 CrossRef PubMed;
(b) T. Kobayashi, J-Aggregates, World Scientific, Singapore, 1996 Search PubMed.
-
(a) S. S. Babu, S. P. Kumar and A. Ajayaghosh, Angew. Chem., Int. Ed., 2012, 51, 1766 CrossRef CAS PubMed;
(b) X. Q. Li, V. Stepanenko, Z. J. Chen, P. Prins, L. D. A. Siebbeles and F. Würthner, Chem. Commun., 2006, 3871 RSC;
(c) P. Terech and R. G. Weiss, Chem. Rev., 1997, 97, 3133 CrossRef CAS PubMed.
- A. K. Mandal, S. Sreejith, T. He, S. K. Maji, X.-J. Wang, S. L. Ong, J. Joseph, H. Sun and Y. Zhao, ACS Nano, 2015, 9, 4796 CrossRef CAS PubMed.
- Y. S. Zhao, H. Fu, F. Hu, A. D. Peng and J. Yao, Adv. Mater., 2007, 19, 3554 CrossRef CAS.
- M. J. Frisch, G. W. Trucks, H. B. Schlegel, G. E. Scuseria, M. A. Robb, J. R. Cheeseman, G. Scalmani, V. Barone, B. Mennucci, G. A. Petersson, H. Nakatsuji, M. Caricato, X. Li, H. P. Hratchian, A. F. Izmaylov, J. Bloino, G. Zheng, J. L. Sonnenberg, M. Hada, M. Ehara, K. Toyota, R. Fukuda, J. Hasegawa, M. Ishida, T. Nakajima, Y. Honda, O. Kitao, H. Nakai, T. Vreven, J. A. Montgomery, J. E. Peralta, F. Ogliaro, M. Bearpark, J. J. Heyd, E. Brothers, K. N. Kudin, V. N. Staroverov, R. Kobayashi, J. Normand, K. Raghavachari, A. Rendell, J. C. Burant, S. S. Iyengar, J. Tomasi, M. Cossi, N. Rega, N. J. Millam, M. Klene, J. E. Knox, J. B. Cross, V. Bakken, C. Adamo, J. Jaramillo, R. Gomperts, R. E. Stratmann, O. Yazyev, A. J. Austin, R. Cammi, C. Pomelli, J. W. Ochterski, R. L. Martin, K. Morokuma, V. G. Zakrzewski, G. A. Voth, P. Salvador, J. J. Dannenberg, S. Dapprich, A. D. Daniels, O. Farkas, J. B. Foresman, J. V. Ortiz, J. Cioslowski and D. J. Fox, Gaussian 09, Revision A.02, Gaussian, Inc., Wallingford, CT, 2009 Search PubMed.
- R. G. Parr and W. Yang, Annu. Rev. Phys. Chem., 1995, 46, 701 CrossRef CAS PubMed.
Footnote |
† Electronic supplementary information (ESI) available: Thin film absorption spectra of dyes, THF : H2O titration spectra, fluorescence lifetime spectroscopy measurements, CV recorded in DCM, optimized geometries of the compounds, FESEM images of samples, optical microscopy images, compiled tabular data for absorption and emission of dyes, DLS, PXRD, thermal analysis, and Cartesian coordinates of the optimized compounds, 1H and 13C NMR spectra of dyes. See DOI: 10.1039/c6ra12776b |
|
This journal is © The Royal Society of Chemistry 2016 |