DOI:
10.1039/C6RA12686C
(Paper)
RSC Adv., 2016,
6, 67102-67112
Ethylene diamine mediated cobalt nanoparticle studded graphene oxide quantum dots with tunable photoluminescence properties†
Received
16th May 2016
, Accepted 2nd July 2016
First published on 4th July 2016
Abstract
Preparation of graphene oxide quantum dot-inorganic nanoparticle hybrids is still an unexplored area of graphene-centred research. The present study demonstrates the ethylene diamine mediated in situ synthesis of cobalt oxide nanoparticles (Co3O4 NPs) studded on graphene oxide quantum dots (GOQDs). Ethylene diamine(en) is known to form a stable coordination complex with Co2+. CoCl2 was used as a precursor material for Co-nanoparticle formation as small sized Co2+ ions can enter the interlayer spacing between the graphene sheets. Ethylene diamine gets attached to the GOQDs via ring opening of epoxy groups on the GOQDs and pulls the Co2+ ions from the medium onto the GOQDs. On treating this system with hydrogen peroxide, GOQDs-en-Co3O4 nanoparticle composites with enhanced optical properties could be successfully prepared. We also tried to explore the potential of our system as a reversible on/off fluorescence switching material based on oxidation–reduction chemistry and could find satisfactory results. Such systems we believe can prove to be model systems for quantum level graphene-inorganic nanoparticle hybrid nanocomposites with potential for application in optoelectronic devices.
Introduction
Graphene, a “wonder material” with the potential to revolutionize the existing field of technology, is presently the most promising material for the future.1 Recently, graphene quantum dots (GQDs) (first reported in 2008) have attracted much attention after their sister material i.e., carbon dots.2 GQDs are single or few-layered graphene sheets with lateral dimensions less than 100 nm depending on the structure of the graphenic nanosheet precursors.3 They are defined as quantum dots smaller than 100 nm in size and less than 10 layers in thickness and possess graphene lattices inside the dots irrespective of the dot size.4 However, carbon dots are defined as quasi-spherical carbon nanoparticles with sizes below 10 nm.5 The chemical structure of GQDs is composed of a regular hexagonal lattice of sp2 carbon atoms edged with heteroatomic functional groups6 decorated with oxygenated groups including hydroxyl, epoxide and carboxyl at their edges similar to graphene oxide.7 GQDs exhibit excellent properties both of graphene materials as well as new and interesting properties resulting from their quantum confinement and edge effects.5 GQDs are non-zero band gap graphene materials8 endowed with a number of interesting properties such as UV-visible fluorescence, luminescence upconversion, hot-carrier generation, and so forth6 in addition to high photostability against photobleaching and blinking, biocompatibility, and low toxicity.2 Advances in solution phase synthesis of GQDs have served as a platform for investigation of optical and optoelectronic properties for novel applications. Consequently, they have a wide and varied range of applications such as in biological imaging and labeling, light emitting diodes, electroluminescence, organic photovoltaic devices, catalysis, and sensors etc.5,6,9 Solution based synthetic approach of preparation of GQDs has potential for producing large quantities of GQDs thereby opening up new opportunities for chemical functionalization. Surface modification via introduction of different chemical functionalities is referred to as functionalization which allow for favorable solubility, enhanced interaction of graphene sheets with polar polymer matrices, various biomolecules, or inorganic precursors in order to be applicable as potential materials in the field of bio-medical science, polymer composites, catalysis,1,10 nanoelectronics, sensors, batteries and supercapacitors.11 Although many surfactant based methods are available, the presence of stabilizers is often undesirable and since practical applications require the presence of functional groups, therefore development of easier ways for better dispersibility and proper functionalization of graphene materials is the need of the hour.10 In order to compensate for the drawbacks arising from graphene, oxidation and reduction techniques can act as potential pathways. On the other hand a major disadvantage of GQDs is their usually low quantum yield (QY). Therefore, continuous efforts for the preparation of GQDs with high QY is demanding and important. Several methods such as chemical reduction and surface passivation are the most common pathways to improve the QY of GQDs and for tuning the fluorescence emission spectra of GQDs. For instance, Li et al.12 used NaBH4 as reducing agent to convert greenish-yellow luminescent GQDs (gGQDs) synthesized by microwave assisted method, to bright blue luminescent GQDs with higher QY. Shen et al.13 prepared the GQDs passivated by poly(ethylene glycol) PEG, and found the QY of as-prepared GQDs-PEG with 360 nm emission was up to 28%, which was two times higher than the pristine GQDs. Sun et al. used alcohols as the reducing agent assisted with UV irradiation to prepare bright blue luminescent GQDs with high QY through photo-reducing pathway.2 Many other techniques include amidation of carboxyl groups, ring-opening reaction of epoxide, nitrogen doping, etc. For example, GQDs surface-passivated by poly(ethylene glycol) (PEG1500N) possess bright blue emission13 The modification of alkylamine or amino groups onto GQDs tuned their emission with a blue or a red shift.14 GQDs grafted with aniline or methylene blue moieties showed efficient photovoltaic properties. The GQDs amidated with a specific recognition moiety can be applied for the optical detection of Cu2+. Luo et al.7 modified GQDs with different aryl groups by Gomberg–Bachmann reaction. Upon the resonance effects between aryl groups and graphene basal planes, the photoluminescence (PL) properties of GQDs are tuned systematically. Moreover, the aryl-modified GQDs showed greatly improved fluorescence properties both in their QYs and pH tolerance. All the chemical functionalization methods however, are based on the use of oxygen functionalities of GQDs as reactive sites for chemical reactions.7 Therefore facile and solution based methods for increasing the photoluminescence properties of GQDs is still being explored. Photoluminescence tuning of GQDs by various other techniques tailoring interesting applications of GQDs in optoelectronics include cutting of fluorinated graphene to fluorinated GQDs with bright blue PL and clear upconversion luminescence properties as reported by Feng et al.15 By a facile hydrothermal approach for cutting preoxidized N-doped graphene into N-doped GQDs which possess excellent upconversion photoluminescent (PL) properties in addition to bright blue PL as reported by Li et al.16 Or by amino functionalization of graphene quantum dots which lead to excitation wavelength dependent tunable heterogeneous PL as reported by Kumar et al.17 On the other hand although a great deal of work has been done on GQDs, there are only a few reports available on synthesis of Graphene Oxide Quantum Dots (GOQDs)18,19 GOQDs have diameter in the range 3–20 nm exhibit zero-dimensional (0 D) distinctive electronic and optical properties owing to their large edge effects and quantum confinement. Zeng et al. reported synthesis of GOQDs from carbon black via a hydrothermal method covalent-surface functionalized PVDF membrane possessing attractive combined features of hydrophilicity, stability, antibiofouling, and antibacterial properties.20 Interesting PL properties were observed by Liu et al. simply by the reduction of GOQDs at different annealing temperatures (300–900 °C) tailoring the PL of reduced GOQDs from blue to purple enabling its potential application in future graphene based optoelectronic devices.21 Recently graphene based composites with inorganic nanostructures, organic crystals, polymers, metal–organic frameworks (MOFs), biomaterials, and carbon nanotubes (CNTs), have been extensively explored in a wide range of applications starting from batteries, supercapacitors, fuel cells, and photovoltaic devices, to photocatalysis, sensing platforms, Raman enhancement and so on. Graphene-inorganic nanohybrids have already been prepared with metals nanoparticles like Au, Ag, Pd, Pt, Ni, Cu, Ru and Rh oxides like TiO2, ZnO, SnO2, MnO2, Co3O4, Fe3O4, NiO, Cu2O, RuO2, and SiO2; and chalcogenides like CdS and CdSe. The fabrication methods for such composites fall into two categories viz. ex situ hybridization, and in situ crystallization. Ex situ hybridization involves the mixing of pre-modified graphene nanosheets with commercially available nanocrystals via non-covalent interactions or chemical bonding. However, it sometimes suffers from the drawback of low density and non-uniform coverage of the nanostructures on the Graphene Oxide (GO)/Reduced Graphene Oxide (rGO) surfaces. On the contrary the in situ crystallization leads to uniform surface coverage of nanocrystals by controlling the nucleation sites on GO/rGO via surface functionalization thereby giving rise to a continuous film of nanoparticles (NPs) on graphene surfaces. In situ method involves the reduction of metal precursors using various reducing agents like amines, NaBH4, and ascorbic acid. Few commonly used techniques for in situ synthesis of metal nanoparticles on graphene surfaces include microwave irradiation, sol–gel process, hydrothermal process, electrochemical deposition etc.22
Although a great deal of work has been done regarding the formation of graphene-inorganic metal nanoparticle composites, as far as our knowledge is concerned the area of GOQDs-inorganic nanoparticle hybrid via in situ formation of metal nanoparticles on GOQDs is yet to be explored. In the present study we report a simple method for one step reduction cum functionalization of GOQDs using ethylene diamine followed by ethylene diamine mediated immobilization of Co2+ within the graphene layers and also at the surface and formation of cobalt oxide nanoparticles (Co3O4 NPs). We chose cobalt chloride as the precursor for nanoparticle synthesis with a view that Co2+ ions can easily enter the interlayer spacings between the graphene layers. Moreover, as we chose ethylene diamine as the reducing agent and it is well known to form stable complex with Co2+, therefore, this acts as the driving force for immobilization of Co2+ on the GOQDs. Further the changes observed upon successful functionalization and in situ nanoparticle formation were monitored using different analytical techniques with special inference from fluorescence spectroscopy, the aspect which has not been much studied for graphene-based inorganic metal nanoparticle composites. Insight into the reversible on/off fluorescence switching property of the functionalized GOQDs is also being provided with quite satisfactory results upto three consecutive oxidation–reduction cycles.
Experimental section
Materials
Commercially available graphene platelet nanopowder (GPN Type 1, thickness 6–8 nm) was purchased from Sisco Research Laboratories Pvt. Ltd., ethylene diamine (≥98%), hydrogen peroxide solution (50%), concentrated sulfuric acid (98%), concentrated nitric acid (70%), cobalt(II) chloride hexahydrate, nickel(II) chloride hexahydrate and copper(II) sulphate pentahydrate were purchased from Merck Specialities Pvt. Ltd. All chemicals were used as received without further purification. The water used throughout the experiments was from a Milli-Q water purification system.
Methods
One step reduction and functionalization of GOQDs with ethylene diamine. For the reduction and functionalization typically 10 mL of the as-prepared GOQDs solution was taken in a round bottom flask and to it 15 mL of 33% ethylene diamine solution was added with continuous stirring. The pH of the reaction mixture was adjusted at around 10 and was refluxed for 8 hours at 80 °C. The color of the reaction mixture gradually turned bright yellow. The mechanism of reduction and functionalization of GOQDs by ethylene diamine via ring opening of epoxy groups has already been reported earlier.24 The proposed mechanism for the reduction of GOQDs by ethylene diamine occurs via reaction of diamine with epoxy groups by a ring opening addition reaction; the driving force for the reaction being the strain on the three-membered epoxy ring which always has a tendency to open up. Further some of the amine groups will also react with the carboxylic acid groups on the GOQDs to form covalent amide linkages thereby leading to covalent functionalization. The content of GOQDs-en in solution was found to be approximately 0.44 μg mL−1. The GOQDs solution reduced and functionalized with ethylene diamine was termed as GOQDs-en.
Ethylene diamine mediated in situ synthesis of Co3O4 NPs on GOQDs. The GOQDs reduced and functionalized by ethylene diamine (GOQDs-en) was used as a medium for the in situ synthesis of cobalt nanoparticles. Typically, 8 mL of the reduced GOQDs solution was taken in a round bottom flask and to it 2 mL of 2 mM cobalt chloride hexahydrate solution (CoCl2·6H2O) was added and stirred magnetically at room temperature for 30 minutes. The color of the solution turned slightly brown. To this solution 4 mL of 30% H2O2 solution was added drop wise with continuous stirring and the reaction mixture was refluxed for 8 hours at 80 °C. This sample was termed as GOQDs-en-Co3O4NPs.
Characterization
Particle size and zeta potential measurements were carried out on a Malvern Zetasizer Nanoseries, Nano-ZS90. Transmission Electron Microscopy (TEM) was carried out on a JEOL, JEM 2100 instrument. Scanning Electron Microscope (SEM) images were collected on a Carl Zeiss ∑igma VP instrument. Powder X-ray diffraction (XRD) spectrum was collected on a Bruker D8 Advance diffractometer. Raman spectra were collected on a STR Series Raman Spectrometer (STR 300) with a 532 nm excitation laser. UV-visible absorption spectra were collected on a Shimadzu UV-VIS Spectrophotometer, UV-2600; fluorescence spectra were collected on a Varian Cary Eclipse Spectrofluorometer. Fourier Transform Infrared Spectra were collected on a Nicolet-6700 FT-IR Spectrophotometer.
Results and discussion
Commercially available graphene nanoplatelets were exfoliated and oxidized in a single step using an acid mixture oxidant i.e., a 3
:
1 mixture of H2SO4/HNO3 by using a simple sonication and centrifugation technique at room temperature as described earlier. This procedure has already been reported to produce oxygen functionalities on all kinds of nanocarbon materials.25,26 The reactive species of the process responsible for the oxidation is nitronium ion (NO2+).14
H2SO4 + HNO3 = HSO4− + NO2+ + H2O |
The dispersion of GOQDs prepared by the above procedure is yellow in color. GOQDs prepared by the above procedure were then both reduced and functionalized in a single step with ethylene diamine (GOQDs-en) and finally the GOQDs-en was used as a medium for the in situ synthesis of cobalt nanoparticles. The schematic representation of the complete process of oxidation-exfoliation of graphene nanoplatelets to form GOQDs, reduction-functionalization of GOQDs to form GOQDs-en and in situ formation of Co3O4 NPs to give GOQDs-en-Co3O4NPs nanocomposite is shown in Scheme 1. The materials synthesized were characterized after each step by UV-visible absorption spectroscopy, fluorescence spectroscopy, Fourier transform infrared spectroscopy, particle size and zeta potential measurements, transmission electron microscopy, scanning electron microscopy, powder XRD and Raman spectroscopy.
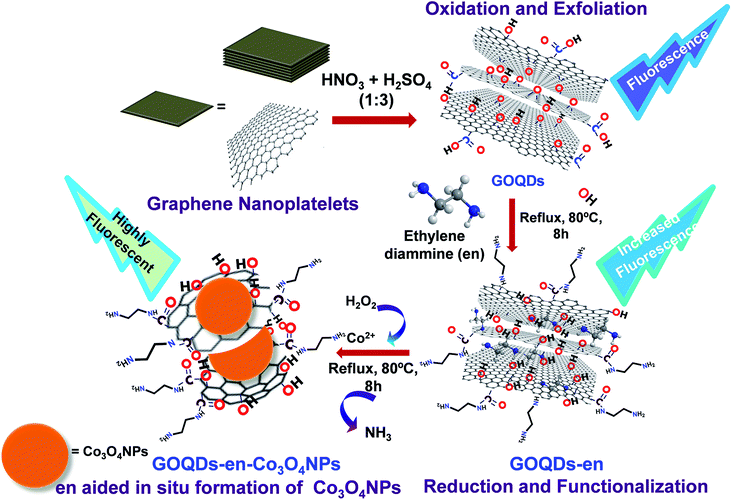 |
| Scheme 1 The schematic representation of the complete process of oxidation-exfoliation of graphene nanoplatelets to form GOQDs, reduction-functionalization of GOQDs to form GOQDs-en and ethylene diammine aided in situ formation of Co3O4 NPs to give GOQDs-en-Co3O4NPs. | |
UV-visible spectroscopy
GOQDs, ethylene diamine reduced GOQDs (GOQDs-en) and that after formation of cobalt oxide nanoparticles (GOQDs-en-Co3O4NPs) were characterized by UV-visible spectroscopy. The stacked UV-visible spectra of GOQDs, GOQDs-en, GOQDs-en-Co3O4NPs and pure cobalt chloride (CoCl2) is shown in Fig. 1. The UV-visible spectrum of GOQDs shows two distinct peaks viz. at ∼230 nm and ∼300 nm. The peak near 230 nm could be assigned to π–π* transition of the C
C bond. The other peak near 300 nm could be assigned to n–σ* transition of the –OH, epoxy and –COO− groups arising due to oxidation. The presence of these two peaks for the oxidized samples indicated the successful oxidation of graphene nanoplatelets. Again for ethylene diamine reduced GOQDs, the absorbance of the 300 nm peak is considerably reduced with a tail extending into the visible region which indicate the successful reduction of GOQDs by ethylene diamine. Further upon titration with Co2+ ions the peak at ∼230 nm was found to be shifted to 224 nm and also the peak ∼300 nm observed for ethylene diamine reduced GOQDs was completely disappeared indicating successful coordination of amine groups to Co2+ ions. However, upon reduction and Co3O4 NPs formation, this peak was found to be reduced in intensity. This can be attributed to the fact that upon reduction the –COO− and epoxy groups are replaced by –NHR groups thereby reducing the number of possible n–σ* transitions and resulting in reduced intensity. After Co3O4 NPs formation the intensity of the peak was found to be further reduced due to the fact that some of the –NH2 groups of ethylene diamine are being liberated as ammonia upon addition of H2O2 thus further decreasing the number of possible n–σ* transitions. The coordination between the amine groups of ethylene diamine and Co2+ ions results in color change, from light pink (CoCl2 solution) to light brown (CoCl2/en solution).
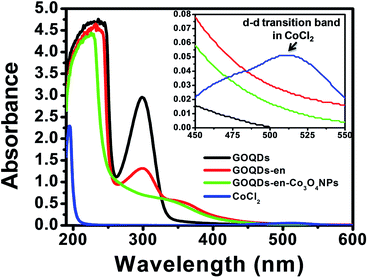 |
| Fig. 1 UV-visible absorption spectra of GOQDs, ethylene diamine reduced GOQDs (GOQDs-en), that after formation of cobalt oxide nanoparticles (GOQDs-en-Co3O4NPs) and of pure cobalt chloride (CoCl2). Inset shows the d–d transition band in CoCl2 which does not appear for GOQDs-en-Co3O4NPs. | |
The UV-visible spectrum of cobalt chloride hexahydrate shows a d–d absorption band at 520 nm as shown in inset of Fig. 1. However, this characteristic band was absent in the spectrum of GOQDs-en-Co3O4NPs nanocomposite indicating that there are no free CoCl2 in the solution. Additionally, in order to confirm the formation of complex between Co2+ and en reduced GOQDs i.e., GOQDs-en, titration experiments were carried out and monitored via UV-Vis spectroscopy. The UV-visible titration curves for Co2+, Ni2+ and Cu2+ with GOQDs-en are shown in ESI Fig. S1(A)–(C)† respectively. The titration curve with Co2+ shows that the intensity of the peaks ∼300 nm and 350 nm gradually increases in intensity as the concentration of Co2+ increases and reaches saturation at a concentration of 460 μM Co2+. However, no such increase was observed for Ni2+ and Cu2+. This is a clear indication of the successful coordination of GOQDs-en with Co2+.
Fluorescence spectroscopy
GOQDs synthesized by the above procedure exhibit a broad excitation dependent PL emission spectra with a maximum emission at ∼530 nm for λexc = 360 nm. The PL from GOQDs has been reported to originate from the combined effect of intrinsic and defect emission although it is still a matter of debate.27 Successful coordination of amine groups of ethylene diamine to Co2+ was also monitored by fluorescence spectroscopy. Fig. 2(A) shows the stacked photoluminescence spectra of GOQDs, GOQDs-en and that after titration of GOQDs-en with cobalt chloride solution.
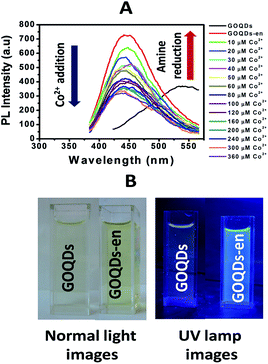 |
| Fig. 2 (A) Stacked photoluminescence spectra of GOQDs, en reduced GOQDs (GOQDs-en) and that after titration with cobalt chloride solution (B) normal light and UV lamp images of GOQDs and GOQDs-en showing enhanced fluorescence intensity upon functionalization. | |
It is to be observed that the GOQDs solution upon excitation at 360 nm exhibits maximum emission at ∼530 nm. This emission was found to be considerably enhanced upon reduction and functionalization with ethylene diamine accompanied with a huge blue shift in the emission maxima. For λexc = 360 nm, the GOQDs-en solution showed maximum emission at ∼450 nm. This significant blue shift in emission maxima of GOQDs after reduction and functionalization with ethylene diamine as has been reported earlier for similar cases28,29 can be attributed to radiative recombination of localized electron–hole pairs within isolated sp3 clusters which in addition to size, surface effects and presence of O-containing groups; contribute to the observed blue shift of PL emission upon functionalization. Further, this emission was found to be quenched gradually upon titration with Co2+ solution in the concentration range 10–360 μM. The PL quenching upon Co2+ addition to GOQDs-en may be attributed to electron transfer from –NH2 groups of ethylene diamine to Co2+ as has been reported earlier for similar systems.30 The photoinduced charge transfer may probably lead to a perturbation of electronic states of GOQDs-en thereby facilitating non-radiative transitions.31 This quenching in fluorescence intensity can be considered as an indication of successful coordination of amine groups with Co2+. Fig. 2(B) shows the normal light and UV lamp images of GOQDs and ethylene diamine (en) reduced GOQDs (GOQDs-en) showing enhanced fluorescence intensity of GOQDs upon functionalization. To check the selectivity of the ethylene diamine reduced GOQDs towards Co2+ complexation, we performed similar titration experiments with Ni2+ and Cu2+. Whereas upon Co2+ addition, the fluorescence intensity of the ethylene diamine-reduced GOQDs was found to be quenched, no such quenching was found with either Ni2+ or Cu2+. The corresponding stacked photoluminescence spectra of GOQDs, en reduced GOQDs and that after titration with Ni2+ and Cu2+ solution is shown in Fig. S2(A) and (B) respectively (ESI†). As evident from the figure there is no considerable change in the PL intensity of GOQDs-en upon titration with Ni2+ or Cu2+ which suggests that there is probably no complexation between en and either of Ni2+ or Cu2+. However it is still not clear why only Co2+ shows such a phenomenon.
In view of the off-on-off fluorescence behavior of the GOQDs:GOQDs-en:GOQDs-en-Co systems, we decided to study the fluorescence properties of the systems again after formation of cobalt oxide nanoparticles. Fig. 3(A) shows the stacked PL spectra of GOQDs, GOQDs-en and GOQDs-en-Co3O4NPs. It was observed that PL intensity was further enhanced upon formation of cobalt oxide nanoparticles along with a marked blue shift in the emission maximum at the same excitation wavelength i.e., 360 nm. The emission maxima for GOQDs, GOQDs-en and GOQDS-en-Co3O4NPs was found to be ∼525 nm, 450 nm and 380 nm respectively for λexc = 360 nm. Fig. 3(B) shows the normal and UV lamp images of GOQDs, GOQDs-en and GOQDS-en-Co3O4NPs. From the images it is quite clear that the fluorescence intensity of the samples increases accompanied with a considerable amount of blue shift.
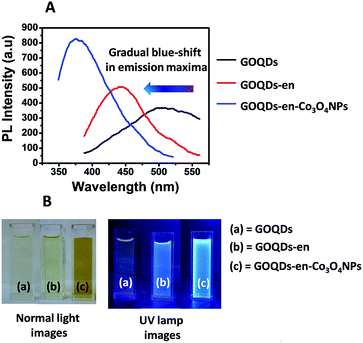 |
| Fig. 3 (A) Stacked PL spectra of GOQDs, GOQDs-en and GOQDs-en-Co3O4NPs showing a gradual blue-shift in the emission maxima for excitation at 360 nm. (B) The normal light and UV lamp images of (a) GOQDs, (b) GOQDs-en and (c) GOQDS-en-Co3O4NPs showing the fluorescence intensity increase of the samples from left to right accompanied with a considerable amount of blue shift. | |
PL evolution of GOQDs can be attributed to the reduction of intrinsic state emissions and increase in the defect state emission. The size of sp2 clusters and defect state density in GOQDs may be altered via functionalization. As the band gap of GOQDs is proposed to increase as size decreases32 amine functionalization is proposed to tune the band gap structure of GOQDs via charge transfer or by forming an inter-band state resulting in shift of PL emission. The blue shift in the PL properties may be attributed to the change of isolated sp2 clusters within the C–O sp3 matrix due to delocalization of electron–hole pairs, the smaller size and higher concentration of sp2 clusters contributing to the energy blue-shift and intensity enhancement.15,21 Further the enhancement of PL intensity is due to the replacement of the –COO− and epoxy groups by –NHR which would otherwise induce non-radiative recombinations.33 Upon formation of Co3O4 NPs there is a further increase in the amine density on GOQDs which arises due to setting free of the cobalt co-ordinated amine groups leading to further enhancement in PL intensity and blue-shift of PL energy.32
To investigate the selectivity of the GOQDs-en towards formation of Co3O4 NPs we carried out similar experiments with Ni2+ and Cu2+ ions to check if there is a change in the fluorescence property of GOQDs-en. The corresponding comparative PL emission spectra after reaction with Ni2+ and Cu2+ are shown in Fig. S3(A) and (B) respectively (ESI†).
From the spectra it was clear that there was no further enhancement in PL intensity of GOQDs-en system after formation of Ni or Cu nanoparticles by the same procedure as adopted for Co3O4 NPs formation. Rather there was observed quenching of PL intensity at the same value of λexc = 360 nm and also there was no considerable blue shift observed. This suggests that probably Ni and Cu nanoparticles are not formed on GOQDs-en by this process.
Fourier transformed infrared spectroscopy, powder XRD and zeta potential analysis
The FTIR spectra further points towards successful ethylene diamine mediated formation of Co3O4 NPs on GOQDs-en.
Fig. 4(A) shows the stacked FTIR spectra of (a) graphene before oxidation (b) GOQDs (c) en (d) GOQDs-en and (e) GOQDs-en-Co3O4NPs. The FTIR spectrum of graphene shows peaks at 2924, 2855 cm−1 (–C–H stretching vibration) and at 1507 cm−1 (–C–C stretch; aromatics). The spectrum for GOQDs shows peaks at 3191 cm−1 (O–H stretch), 1748 cm−1 (C
O stretch), 1508 cm−1 (C–C (in ring) aromatics), 1455 (C–H bend), 1293, 1171 cm−1 (C–O stretch; alcohol, carboxylic acid, ether), 1004 cm−1 (
C–H bend) and 550 cm−1 (C–H aromatics). The spectrum of GOQDs when compared with that of graphene shows the successful oxidation and incorporation of different functional groups into the graphene nanoplatelets. On the other hand peaks for ethylene diamine (en) occur at 3290 cm−1 (N–H stretch 1° amine), 3043 cm−1 (C–H stretch alkanes), 1667 cm−1 (N–H bend 1° amine), 1329 cm−1 (C–H rock alkanes), 1179, 1063 cm−1 (C–N stretch aliphatic amine) and 947 cm−1 (N–H wag 1° amine). Upon reduction cum functionalization of GOQDs with ethylene diamine, the peak positions appear at 3040 cm−1 (C–H stretch alkanes), 1570 cm−1 (–NH2 bending), 1380 cm−1 (C
C) and 1100 cm−1 (C–N stretch). The absence of the peak at 1748 cm−1 (C
O stretch for carboxylic acid), and 1171 cm−1 (C–O stretch) for GOQDs-en is a clear indication of the interaction of en with –COOH groups to form amide linkages and ring opening of epoxy groups to form –OH and –NHR groups on GOQDs-en. Further upon formation of Co3O4NPs on GOQDs-en as proposed, the peak positions appear at 3040 cm−1 (C–H stretch alkanes), 1670 (stretching mode of amide), 1570 cm−1 (–NH2 bending), 1380 cm−1 (C
C) and the peak at 1100 cm−1 (C–N stretch) is shifted to 1120 cm−1 after formation of Co3O4 NPs.
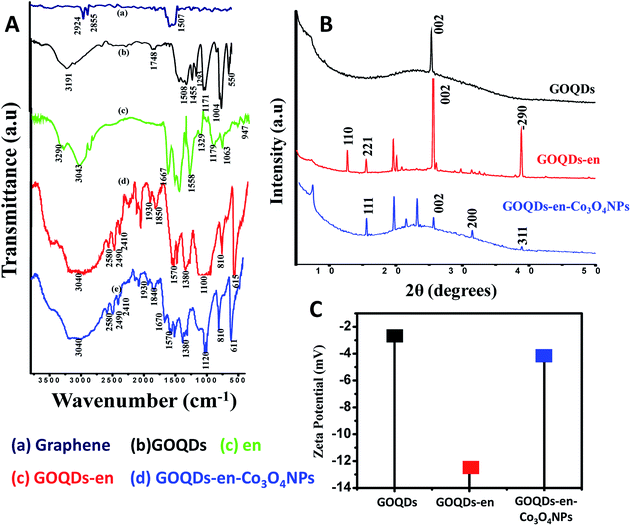 |
| Fig. 4 (A) The stacked FTIR spectra of (a) graphene before oxidation (b) GOQDs (c) en (d) GOQDs-en and (e) GOQDs-en-Co3O4NPs. (B) Powder XRD spectra of GOQDs, GOQDs-en and GOQDs-en-Co3O4NPs. (C) The comparative zeta potential plot for GOQDs, GOQDs-en and GOQDs-en-Co3O4NPs. | |
Further support of ethylene diamine mediated successful in situ formation of Co3O4 NPs on GOQDs-en can be drawn from powder XRD analysis of GOQDs, GOQDs-en and GOQDs-en-Co3O4NPs as shown in Fig. 4(B). The diffractogram for GOQDs shows diffraction peak at 2θ = 25.5°(002) corresponding to the disorderedly stacked graphene sheets34 upon oxidation to form GOQDs (the GOQDs prepared are not monolayer and might possess a few layers of stacked graphene with a well-defined lattice structure). The spectrum of GOQDs-en shows sharp and well-defined peaks at 2θ values of 12.76(110), 15.6(221), 25.5(002) and 38.9(−290) degrees. These peaks were probably found to occur due to successful incorporation of –NH2 groups into the planar structure of the GOQDs (details of peak assignment in ESI†). On the other hand diffraction peaks appearing at 2θ = 19.7° (111), 31.5° (220) and 38.8° (311) along with the (002) peak for GOQD-en-Co3O4NPs can be ascribed to the well-crystallized Co3O4 with a face-centered cubic structure.35
Evidence in support of reduction and functionalization of GOQDs and in situ formation of Co3O4 NPs on GOQDs-en can be drawn from the zeta potential results. Fig. 4(C) shows the comparative zeta potential plot for GOQDs, GOQDs-en and GOQDs-en-Co3O4NPs. From the plot the variation of zeta potentials at every step is very clear. The zeta potential value for GOQDs observed to be −2.6 mV was subsequently changed to −12.8 mV upon formation of GOQDs-en due to increased electron density on formation of –OH groups via ring opening of epoxy groups and formation of –NHCH2–CH2–NH2 linkages. Formation of Co3O4 NPs again leads to an increase in the zeta potential value to −4.51 mV, indicating a decrease in the electron density due to elimination of some –NH2 groups as ammonia on addition of H2O2 to form Co3O4 NPs.
Raman spectroscopy
In order to investigate the successful oxidation, amine reduction with functionalization of GOQDs and cobalt coordination to form nanoparticles we recorded the Raman spectra of all the samples. Fig. 5 shows the stacked Raman spectra of GOQDs, GOQDs-en and GOQDs-en-Co3O4NPs.
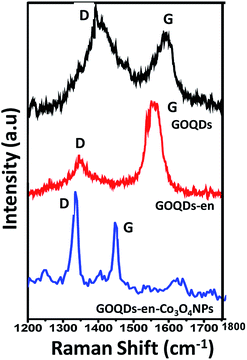 |
| Fig. 5 The stacked Raman spectra of GOQDs, GOQDs-en and GOQDS-en-Co3O4NPs. | |
Table 1 summarizes the respective bands and their positions for the different samples.
Table 1 The positions at which the respective D, G and 2D bands occur for GOQDs, GOQDs-en and GOQDS-en-Co3O4NPs along with the intensity ratios of the bands
Sample |
Peak position (cm−1) |
ID/IG |
Band |
D |
G |
GOQDs |
1344 |
1598 |
1.12 |
GOQDs-en |
1357 |
1587 |
0.55 |
GOQDs-en-Co3O4NPs |
1335 |
1548 |
1.23 |
From the figure it is quite evident that the Raman spectrum for GOQDs shows two distinct peaks viz. D and G at 1334 and 1598 cm−1 respectively. The appearance of the D peak at 1334 cm−1 for GOQDs is an indication of the disordered structure of graphene to form GOQDs due to oxidation by the acid mixture. However, after reduction cum functionalization by ethylene diamine i.e., for GOQDs-en the D and G peaks were found to appear at 1357 and 1587 cm−1 respectively. More interestingly, upon formation of cobalt oxide nanoparticles, i.e., for GOQDs-en-Co3O4NPs the two characteristic peaks were found to appear at 1335 and 1548 cm−1 respectively. Additionally, it is to be observed that there is a shift in the peak positions of all the three samples as compared to each other. This shifting of the D and G bands indicates successful functionalization and interaction of amine functionalized reduced GOQDs and Co3O4 NPs.36 Further, the ratio between the intensities of characteristic bands gives indication about the functional group insertion.37 Therefore as observed from the spectrum the decreased D/G ratio of GOQDs-en provides evidence for the reduction of GOQDs by ethylene diamine, which reduces the number of defects on the surface of the quantum dots33,38 as well as stabilizes the GOQDs by entering into the interlayer spaces via ring opening of the epoxy groups. The subsequent increase in the ID/IG ratio for GOQDs-en-Co3O4NPs indicates the creation of more defects after attachment of Co3O4 NPs to GOQDs-en.36
Transmission electron microscopy (TEM) and energy dispersive X-ray (EDX) analysis
The morphology and size of the GOQDs prepared was studied using TEM. Fig. 6(A) shows the TEM micrograph of GOQDs. The TEM image of GOQDs shows particles in the range 5–15 nm in size. This was also confirmed from DLS and SEM analysis as shown in the Fig. S4 (ESI†). In order to confirm the formation of Co3O4 NPs on the GOQDs-en, EDX analysis was carried out. Fig. 6(B) shows the EDX spectrum of GOQDs-en-Co3O4NPs. The spectrum clearly shows the presence of the elements C, N, O and Co and the corresponding weight percentages of these elements are shown in Table 2.
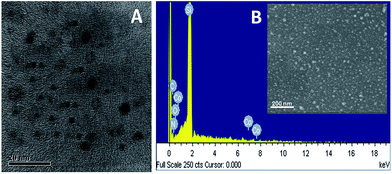 |
| Fig. 6 (A) TEM micrograph of GOQDs, (B) EDX spectrum of GOQDs-en-Co3O4NPs: inset shows the SEM image of GOQDs-en-Co3O4NPs. | |
Table 2 The various elements present in GOQDs, GOQDs-en and GOQDs-en-Co3O4NPs along with their weight percentages
Sample |
C (wt%) |
O (wt%) |
N (wt%) |
Co (wt%) |
Si (wt%) from substrate |
GOQDs |
7.65 |
3.94 |
— |
— |
88.41 |
GOQDs-en |
9.35 |
1.25 |
1.82 |
— |
87.58 |
GOQDs-en-Co3O4NPs |
15.31 |
1.09 |
0.59 |
0.04 |
82.96 |
From the table it is observed that the weight percentages of oxygen decreases upon reduction and functionalization with en which is consistent with the conversion of –COOH groups on the sheet surface to form –CONH2. Further after formation of Co3O4 NPs, the weight percentage of nitrogen was decreased to half and this is consistent with the liberation of N atoms as ammonia on addition of H2O2 to form Co3O4 NPs. The remaining 0.59% N indicates the presence of –CONH2 groups on the surface of GOQDs-en. The EDX spectra for GOQDs and GOQDs-en were also recorded and are shown in ESI Fig. S5(A) and (B)† respectively. Therefore EDX analysis provides evidence for the successful oxidation, reduction and functionalization and formation of Co3O4 NPs on GOQDs.
Owing to the PL switching behaviour of GOQDs-en and GOQDs-en-Co3O4NPs we decided to study the reversibility behaviour of this system in order to monitor the restoration of PL intensity. Fig. 7 shows the reversible switching cycle of GOQDs-en (a), GOQDs-en-Co3O4NPs (b) and after re-reduction with en to release Co2+ (c) upto three consecutive cycles. Re-reduction of GOQDs-en-Co3O4NPs results in decrease of PL intensity as represented by point (c) in Fig. 7. Further re-oxidation with H2O2 causes an enhancement in the PL intensity point (d) due to formation of Co3O4 NPs and so on (e)–(g). Therefore this system can be very well suited for reversible on/off fluorescence switching devices based on functionalized hybrid quantum systems.
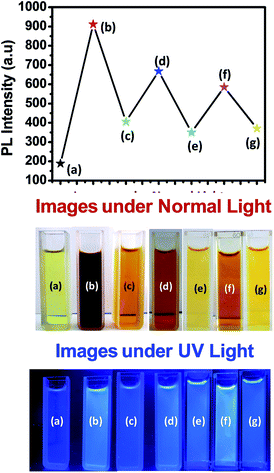 |
| Fig. 7 Reversibility graph for the consecutive oxidation–reduction of the functionalized GOQD hybrid system and the corresponding images of the samples under normal and UV-light. | |
Table 3 shows the corresponding increase in PL intensities upto three consecutive oxidation-reduction cycles.
Table 3 The percentage restoration of PL intensities upto three consecutive oxidation-reduction cycles
Sl. No. |
Cycle no. |
% restoration of PL intensity |
1 |
Cycle 1 |
79.317 |
2 |
Cycle 2 |
71.760 |
3 |
Cycle 3 |
69.121 |
Therefore from experimental evidences discussed so far, we can summarize the process as successful oxidation, exfoliation of graphene platelet nanosheets into GOQDs by introducing different oxygen functionalities into their surface; successful reduction and functionalization of GOQDs by ethylene diamine via ring opening of epoxy groups on the surface of the different layers of GOQDs by formation of –NH linkages to form GOQDs-en and finally immomibilization of Co2+ ions by coordination to ethylene diamine within the layers and on the surface of GOQDs-en via in situ formation of Co3O4 NPs.
The mechanism of reduction and functionalization of GOQDs by ethylene diamine via ring opening of epoxy groups has already been reported earlier.24 The proposed mechanism for the reduction of GOQDs by ethylene diamine occurs via reaction of diamine with epoxy groups by a ring opening addition reaction; the driving force for the reaction being the strain on the three-membered epoxy ring which always has a tendency to open up. Further some of the amine groups will also react with the carboxylic acid groups on the GOQDs to form covalent amide linkages. The schematic representation of the reduction of GOQDs by ethylene diamine and covalent functionalization to form –NHR linkages is shown in Scheme 2(A). Schematic representation of immomibilization of Co2+ ions by coordination to ethylene diamine both within the layers and on the surface of GOQDs-en and in situ formation of Co3O4 NPs is shown in Scheme 2(B). The mechanism for formation of Co3O4 NPs is proposed to be the coordination between ethylene diamine attached to the GOQDs and Co2+ ions to form a stable complex which causes the immobilization of Co2+ within the graphene layers of GOQDs-en. Further addition of H2O2 leads to the formation of Co3O4 NPs with liberation of ammonia due to the oxidizing nature of H2O2 thereby indicating the successful breaking of Co–N bond to form Co3O4 NPs. The procedure adopted for formation of Co3O4 NPs has already been reported and is employed in our system with slight modifications.34
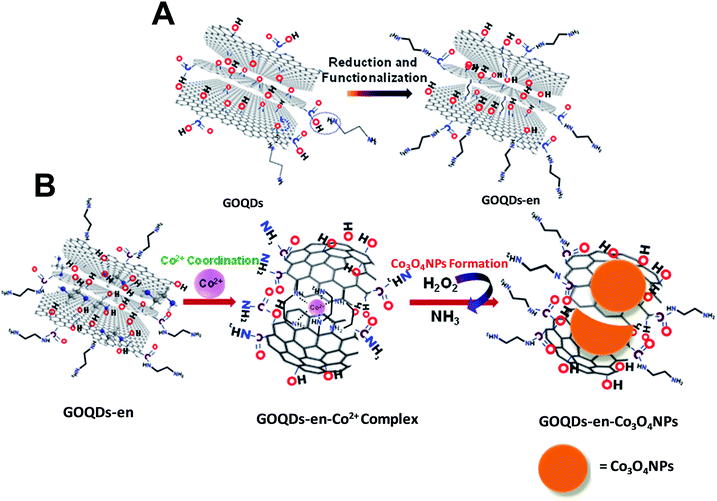 |
| Scheme 2 (A) The schematic representation of the reduction of GOQDs by ethylene diamine and covalent functionalization to form –NHR linkages on GOQDs. (B) Schematic representation of immomibilization of Co2+ ions by coordination to ethylene diamine within the layers of GOQDs-en and in situ formation of Co3O4 NPs. | |
Conclusions
In the present study we successfully synthesized graphene oxide quantum dots and report a simple method for one step reduction cum functionalization of GOQDs using ethylene diamine followed by ethylene diamine mediated immobilization of Co2+ on the GOQDs via in situ formation of cobalt oxide nanoparticles. Ethylene diamine gets attached to the layers of GOQDs via ring opening of epoxides and acts as the driving force to pull the Co2+ ions into the interlayer spacings such that the ions get immobilized onto the GOQDs. Functionalization of GOQDs with ethylene diamine led to an enhanced fluorescence intensity with a huge blue shift and this was tremendously increased upon in situ Co3O4 NPs formation on the GOQDs with further blue shift in PL emission. All the experimental evidences suggest the successful formation of GOQDs-en-Co3O4NPs nanocomposite. Moreover, in our work we tried to prepare quantum level GOQDs-inorganic hybrid material by using a very simple solution based strategy and we observed that the optical property i.e., fluorescence of GOQDs could be greatly enhanced at the same time. This study reports the synthesis of an organic–inorganic nanohybrid material for tuning the optical properties of native graphene quantum dots via functionalization thereby adding a new dimension to the possible applicability of graphene as optical switching materials. Functionalized carbon dots showing similar reversible optical properties have also been reported by us in our previous work.39 Since this aspect has not been highly explored, therefore we feel our system can prove to be a model for opening up new possibilities for synthesis and possible optoelectronic applications of such systems in future.
Acknowledgements
The authors thank SERB, New Delhi, Grant No. SB/S1/PC-69/2012 and BRNS, Mumbai, Grant No. 34/14/20/2014-BRNS. We would like to thank CIF, IIT Guwahati for Raman and TEM analysis. U.B wants to thank IASST for fellowship.
References
- M. Song and D. Cai, RSC Nanosci. Nanotechnol., 2012, 26, 1–51 CAS.
- H. Sun, L. Wu, N. Gao, J. Ren and X. Qu, ACS Appl. Mater. Interfaces, 2013, 5, 1174–1179 CAS.
- V. Georgakilas, J. A. Perman, J. Tucek and R. Zboril, Chem. Rev., 2015, 115, 4744–4822 CrossRef CAS PubMed.
- Z. Zhang, J. Zhang, N. Chen and L. Qu, Energy Environ. Sci., 2012, 5, 8869–8890 CAS.
- Y. Sun, S. Wang, C. Li, P. Luo, L. Tao, Y. Wei and G. Shi, Phys. Chem. Chem. Phys., 2013, 15, 9907–9913 RSC.
- W. Kwon, Y. H. Kim, C. L. Lee, M. Lee, H. C. Choi, T. W. Lee and S.-W. Rhee, Nano Lett., 2014, 14, 1306–1311 CrossRef CAS PubMed.
- P. Luo, Z. Ji, C. Li and G. Shi, Nanoscale, 2013, 5, 7361–7367 RSC.
- L. Lin and S. Zhang, Chem. Commun., 2012, 48, 10177–10179 RSC.
- L. M. Dong, D. Y. Shi, Z. Wu, Q. Li and Z. D. Han, Digest Journal of Nanomaterials and Biostructures, 2015, 10, 855–864 Search PubMed.
- E. D. Grayfer, A. S. Nazarov, V. G. Makotchenko, S. J. Kim and V. E. Fedorov, J. Mater. Chem., 2011, 21, 3410–3414 RSC.
- B. Jiang, C. Tian, L. Wang, Y. Xu, R. Wang, Y. Qiao, Y. Mac and H. Fu, Chem. Commun., 2010, 46, 4920–4922 RSC.
- L. L. Li, J. Ji, R. Fei, C. Z. Wang, Q. Lu, J. R. Zhang, L. P. Jiang and J. J. Zhu, Adv. Funct. Mater., 2012, 22, 2971–2979 CrossRef CAS.
- J. H. Shen, Y. H. Zhu, C. Chen, X. L. Yang and C. Z. Li, Chem. Commun., 2011, 47, 2580–2582 RSC.
- S. Zhu, J. Zhang, S. Tang, C. Qiao, L. Wang, H. Wang, X. Liu, B. Li, Y. Li, W. Yu, X. Wang, H. Sun and B. Yang, Adv. Funct. Mater., 2012, 22, 4732–4740 CrossRef CAS.
- Q. Feng, Q. Cao, M. Li, F. Liu, N. Tang and Y. Du, Appl. Phys. Lett., 2013, 102, 013111 CrossRef.
- M. Li, W. Wu, W. Ren, H.-M. Cheng, N. Tang, W. Zhong and Y. Du, Appl. Phys. Lett., 2012, 101, 103107 CrossRef.
- G. S. Kumar, R. Roy, D. Sen, U. K. Ghorai, R. Thapa, N. Mazumder, S. Saha and K. K. Chattopadhyay, Nanoscale, 2014, 6, 3384–3391 RSC.
- S. Chen, J. W. Liu, M. L. Chen, X. W. Chen and J. H. Wang, Chem. Commun., 2012, 48, 7637–7639 RSC.
- Y. Zhang, H. Gao, J. Niu and B. Liu, New J. Chem., 2014, 38, 4970–4974 RSC.
- Z. Zeng, D. Yu, Z. He, J. Liu, F.-X. Xiao, Y. Zhang, R. Wang, D. Bhattacharyya, T. Thatt and Y. Tan, Sci. Rep., 2016, 6, 20142 CrossRef CAS PubMed.
- F. Liu, T. Tang, Q. Feng, M. Li, Y. Liu, N. Tang, W. Zhong and Y. Du, J. Appl. Phys., 2014, 115, 164307 CrossRef.
- X. Huang, X. Qi, F. Boeyab and H. Zhang, Chem. Soc. Rev., 2012, 41, 666–686 RSC.
- U. Baruah and D. Chowdhury, Nanotechnology, 2016, 27, 145501 CrossRef PubMed.
- H. Liang, J. M. Raitano, L. Zhang and S. W. Chan, Chem. Commun., 2009, 48, 7569–7571 RSC.
- J. Zhang, H. Zou, Q. Qing, Y. Yang, Q. Li, Z. Liu and Z. D. Guo, J. Phys. Chem. B, 2003, 107, 3712–3718 CrossRef CAS.
- S. C. Hens, W. G. Lawrence, A. S. Kumbhar and O. Shenderova, J. Phys. Chem. C, 2012, 116, 20015–20022 Search PubMed.
- Y. Y. Zhao, H. Cheng, Y. Hu, G. Shi, L. Dai and L. Qu, J. Am. Chem. Soc., 2012, 134, 15–18 CrossRef PubMed.
- J. Shen, Y. Zhu, X. Yang and C. Li, Chem. Commun., 2012, 48, 3686–3699 RSC.
- Y. Wang, L. Zhang, R. P. Liang, J. M. Bai and J. D. Qiu, Anal. Chem., 2013, 85, 9148–9155 CrossRef CAS PubMed.
- H. Xu, S. Zhou, L. Xiao, H. Wang, S. Lia and Q. Yuan, J. Mater. Chem. C, 2015, 3, 291–297 RSC.
- J. Che, L. Shena and Y. Xiao, J. Mater. Chem., 2010, 20, 1722–1727 RSC.
- J. M. Yoo, J. H. Kang and B. H. Hong, Chem. Soc. Rev., 2015, 44, 4835–4852 RSC.
- Z. Wang, H. Zeng and L. Sun, J. Mater. Chem. C, 2015, 3, 1157–1165 RSC.
- Z. S. Wu, W. Ren, L. Wen, L. Gao, J. Zhao, Z. Chen, G. Zhou, F. Li and H. M. Cheng, ACS Nano, 2010, 4, 3187–3194 CrossRef CAS PubMed.
- C. H. Kuo, W. Li, W. Song, Z. Luo, A. S. Poyraz, Y. Guo, A. W. K. Ma, S. L. Suib and J. He, ACS Appl. Mater. Interfaces, 2014, 6, 11311–11317 CAS.
- M. S. A. S. Shah, W.-J. Kim, J. Park, D. K. Rhee, I.-H. Jang, N.-G. Park, J. Y. Lee and P. J. Yoo, ACS Appl. Mater. Interfaces, 2014, 6, 20819–20827 Search PubMed.
- C.-M. Damian, A. M. Pandele and H. Iovu, Sci. Bull.–"Politeh." Univ. Bucharest, Ser. B, 2010, 72, 165–174 Search PubMed.
- G. Luo, X. Jiang, M. Li, Q. Shen, L. Zhang and H. Yu, ACS Appl. Mater. Interfaces, 2013, 5, 2161–2168 CAS.
- U. Baruah, M. J. Deka and D. Chowdhury, RSC Adv., 2014, 4, 36917–36922 RSC.
Footnote |
† Electronic supplementary information (ESI) available: UV-visible titration curves for Co2+, Ni2+ and Cu2+ with GOQDs-en, stacked photoluminescence spectra of GOQDs, en reduced GOQDs (GOQDs-en) and that after titration with Ni2+ and Cu2+ solution, stacked PL emission spectra of GOQDs, GOQDs-en and that after reaction with Ni 2+ and Cu2+, the particle size graphs and zeta potential values of GOQDs, GOQDs-en and GOQDs-en-Co3O4NPs obtained from Dynamic Light Scattering (DLS) and SEM techniques and EDX spectra for GOQDs and GOQDs-en. See DOI: 10.1039/c6ra12686c |
|
This journal is © The Royal Society of Chemistry 2016 |