DOI:
10.1039/C6RA12507G
(Communication)
RSC Adv., 2016,
6, 77431-77439
Functionalised dihydroazo pyrimidine derivatives from Morita–Baylis–Hillman acetates: synthesis and studies against acetylcholinesterase as its inhibitors†
Received
13th May 2016
, Accepted 3rd August 2016
First published on 5th August 2016
Abstract
A wide array of dihydro[1,5]azo[1,2-a]pyrimidine 2-esters have been synthesised at room temperature in moderate to good yields through one-pot reaction between nitrostyrene derived MBH acetates and aminoazole derivatives with γ-α cyclisation. The reaction involves a cascade SN2 reaction followed by intramolecular Michael addition–cyclisation with the generation of two new carbon–nitrogen bonds. The structure was further confirmed by single X-ray crystal analysis. Docking and in vitro studies of dihydrobenzimidazo pyrimidine derivatives against acetylcholinesterase (AChE) have been performed and the compounds 3d and 3e exhibit potent inhibitory activities with an IC50 of 46.8 nM and 42.5 nM respectively.
Introduction
Diversely functionalised heterocyclic rings exist in nature and in many biologically relevant compounds, such as nucleic acids, antibiotics and hormones. A wide range of pharmacological properties shown by heterocyclic units inspired synthetic organic and medicinal chemists to pursue the synthesis of novel molecules and study their biological properties. Among them, benzimidazoles, imidazoles, triazoles and tetrazoles have exhibited biological properties such as anticancer and antimicrobial, and serve as antitubercular agents, benzodiazepine receptor agonists, calcium channel blockers and are also present as core structures in several currently marketed drugs.1,2 The various biological properties of imidazole and fused imidazole derivatives, such as antibacterial, antifungal, analgesic, antitubercular, anticancer, anti-HIV, antiarthritic and antitumor, have been extensively investigated.3 Imidazo[4,5-b]pyridines, which are structurally related to benzimidazoles have shown diverse biological activities such as cytotoxic activities depending on the substituents of the heterocyclic ring.4 Several anxiolytic drugs such as fasiplon, taniplon, and divaplon holding imidazopyrimidine fragments are currently used as drugs in market5 (Fig. 1).
 |
| Fig. 1 Imidazopyrimidine containing drugs. | |
The main concept for the synthesis of novel dihydroimidazo pyrimidine ester derivatives originated from biological importance of dihydropyrimidines and imidazole derivatives. They exhibit pharmacological properties like anticancer activity, antiplatelet activity,6 HIV-1 integrase inhibitors7a and anti-Alzheimer's disease.7b–d Moreover, these pyrimidines and imidazole/benzimidazole derivatives are reported to have AChE inhibitory properties.7a,e–k Also the structure activity studies proved that pyrimidine moieties plays an important role in the inhibition of AChE7b,h and the benzimidazole scaffold is the ring isoster of indanone moiety of donepezil, one of the most potent AChE inhibitor. Hence it was assumed that combining the two inhibitory moieties may further enhance the inhibitory potency towards AChE. More over expansion of aromatic plane may improve stacking and hydrophobic interaction with the enzyme as these interactions plays a crucial role in the binding of inhibitors to AChE.
Acetylcholinesterase inhibitors (AChEIs) temporarily restore native levels of the neurotransmitter acetylcholine (Ach) and prevents the loss of cholinergic transmission in brain areas. However, cholinergic deficit is one of the hallmarks of Alzheimer's disease (AD).8a,b AD, is associated with selective loss of cholinergic neurons and reduce the levels of acetylcholine neurotransmitter and it is characterised by progressive loss of memory, attention and depression.8c According to the Alzheimer's Association, AD is the one of the top 10 causes of death in high income countries. In recent decades, researchers focused on the increase of cholinergic neurotransmission by inhibiting the enzyme AChE as one of the main stream option for AD. Currently, the drugs launched in market for cholinergic approach for AD such as tacrine, donepezil, rivastigmine and galantamine (Fig. 2) increase neurotransmission at cholinergic synapses in the brain and improving cognition.
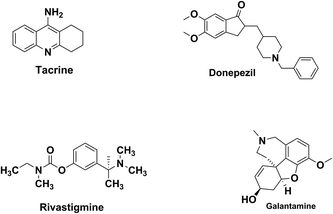 |
| Fig. 2 AChE inhibitors clinically used for the treatment of AD. | |
Therefore, the desirable protocols that would generate various heterocyclic compounds for the synthesis of natural and bio-active compounds is of great demand. Recently, cascade reactions gained tremendous potential in the area of organic synthesis to maximise the results. We envisioned that synthesis of dihydro[1,5]azo[1,2-a] pyrimidine 2-ester derivatives could be possible by reaction of nitrostyrene derived MBH acetates and aminoazole derivatives (Scheme 1).
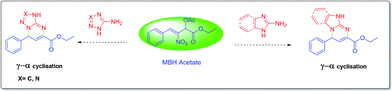 |
| Scheme 1 Strategy towards construction of dihydroazo pyrimidine derivatives. | |
The Morita–Baylis–Hillman (MBH) reaction9 has an attractive strategy for the synthesis of multifunctional heterocyclic molecules in recent years. The MBH reaction is a key step in the synthesis of bioactive and designed molecules.10 Earlier, Baylis and Hillman reported the synthesis of α-hydroxyethyl nitroethylenes from MBH reaction.11 Later, Namboothiri and Chen made further investigation on this reaction and emphasized the use of electrophilic ethyl glyoxylate.12 The MBH reaction of nitroalkenes have been utilised for the synthesis of several heterocycles and carbocycles.13 Further acetylation on hydroxyl group of these conjugated nitroalkene afforded MBH acetates as excellent Michael acceptors containing four potential electrophilic sites (α, β, γ, δ). All four electrophilic sites are highly reactive and used as efficient synthons to synthesise the diversified molecules (Scheme 2). The MBH adducts, can act as dielectrophilic reagents to undergo cascade reactions with various bifunctional nucleophiles.14
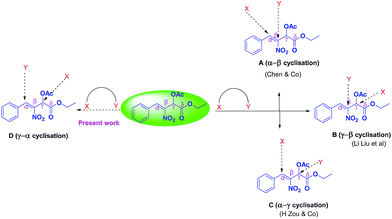 |
| Scheme 2 Potential electrophilic sites of MBH acetates. | |
Recently Namboothiri and Chen demonstrated that the potential electrophilic site at α-position and further Michael addition at β-position in the synthesis of fused furans,15 imidazopyridines,16 and 2-substituted imidazoles.17 Li Liu demonstrated the formation of γ–α and γ–β cyclic products using 2-mercapto benzimidazoles for the synthesis of benzimidazo[2,1-b]-1,3-thiazine and thiazolo[3,2-a]benzimidazole respectively.18 Nair, reported the synthesis of fused pyrans using MBH acetates with cyclopentanedione as α–γ cyclic product.19 Recently, Shao reported the cascade reaction of MBH acetates with N-substituted hydrazine for the synthesis of pyrazoles, in which the reaction was initiated by a SN2 reaction at the electrophilic γ-position followed by Michael addition at α-position.20 Zou demonstrated the reactivity of all four subsequent potential electrophilic sites (α, β, γ, δ) of MBHAs to form imidazo[1,2-a]pyridines (α–α), indolizines (α–β), pyrroles (α–γ) and benzo[b][1,6]oxazocin-2-ones (α–δ).21 Herein, we firstly report a facile regioselective attack at γ position of MBH acetates using various bifunctional nucleophiles such as 2-aminobenzimidazole, 2-aminoimidazoles and 2-amino-1,3,5 triazole followed by another at α-position to give rise to functionalised dihydroazo pyrimidine derivatives with γ–α cyclisation.
Results and discussion
Chemistry
Our initial efforts were focused on optimising the reaction parameters with respect to base, solvent and temperature. Accordingly, the reaction was studied with MBH acetate of nitro alkene 1 and 2-amino benzimidazole 2 as a model substrate. The reaction was performed in THF at room temperature without base. Unfortunately no product formation was observed by LCMS even after heating at 70 °C (Table 1, entry 1 and 2). While adding organic bases like triethyl amine and DIPEA, we observed the formation of 4-phenyl-1,4-dihydrobenzo[4,5]imidazo[1,2-a]pyrimidine-2-carboxylic ester 3 in lower yields (Table 1, entry 3 and 4). Our sub-sequent efforts were focused on use of bases such as pyridine, 2,6-lutidine, DABCO, DBU which delivered 3 in 40–50% yield (Table 1, entry 5–8) and inorganic bases like K2CO3, Cs2CO3 gave compound 3 in moderate to good yields (Table 1, entry 9 and 10). Among all the bases screened, Cs2CO3 was found to give a superior yield (80%) in THF over 12 h at RT. Among the solvent screens (CH2Cl2, CHCl3, MeOH, EtOH, CH3CN, and 1,4-dioxane) (Table 1, entry 11–16), CH3CN and 1,4-dioxane shows the formation of compound 3 in good yield (∼81%). Finally we optimised the condition is Cs2CO3 (2.0 eq.) in acetonitrile/1,4-dioxane as a solvent of choice to yield compound 3 (82%) at RT.
Table 1 Screening of reaction conditionsa

|
S. no |
Base |
Solvent |
Temp |
Yieldb (%) |
All reactions were performed with 0.34 mmol of 1 and 2 with 4 mL of solvent for 12 h. Isolated yield by column chromatography. |
1 |
— |
THF |
RT |
0 |
2 |
— |
THF |
70 °C |
0 |
3 |
Et3N |
THF |
RT |
20 |
4 |
DIPEA |
THF |
RT |
22 |
5 |
Pyridine |
THF |
RT |
40 |
6 |
2,6-Lutidine |
THF |
RT |
40 |
7 |
DABCO |
THF |
RT |
44 |
8 |
DBU |
THF |
RT |
49 |
9 |
K2CO3 |
THF |
RT |
60 |
10 |
Cs2CO3 |
THF |
RT |
80 |
11 |
Cs2CO3 |
CH2Cl2 |
RT |
45 |
12 |
Cs2CO3 |
CHCl3 |
RT |
48 |
13 |
Cs2CO3 |
MeOH |
RT |
60 |
14 |
Cs2CO3 |
EtOH |
RT |
68 |
15 |
Cs2CO3 |
CH3CN |
RT |
82 |
16 |
Cs2CO3 |
1,4-Dioxane |
RT |
81 |
Having these optimised conditions in hand, we explored the scope of 2 with different MBH acetates 1. All the reactions were completed in 12 h at room temperature to give the desired product in moderate to good yields. The yields were good with MBH acetates possessing electron donating groups (3b, 3c, 3h and 3l) to afford the corresponding products in excellent yields (73–82%). On other hand, MBH acetates bearing hindered aryl groups at ortho position (3d, 3e, 3i) delivered moderate yields (60–70%). The yields were low in case of MBH acetates having strong electron withdrawing groups (3j, 3k) and pyridine substrates (3f, 3g). We next carried the scope of this optimised conditions with 2-aminoimidazoles for the synthesis of 5-phenyl-5,8-dihydroimidazo[1,2-a]pyrimidine-7-carboxilic ester derivatives (3m–3r). It was observed that, compared to 2-aminobenzimidazole, 2-aminoimidazole required 15 h to obtain the desired product in moderate yields (45–66%) (Scheme 3).
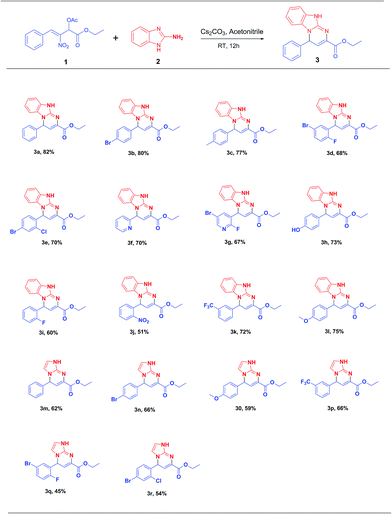 |
| Scheme 3 Substrate scope of MBHAs towards reaction with 2-aminobenzimidazoles and 2-aminoimidazoles. | |
Our studies were extended to use of 3-amino 1,2,4-triazoles for the synthesis of 7-phenyl-4,7-dihydro-[1,2,4]triazolo[1,5-a]pyrimidine-5-carboxylicesters with MBHAs using the optimised conditions. In case of 3-amino 1,2,4-triazoles, we observed two regio isomers in the ratio of 7
:
3 by LCMS and NMR. To improve the regioselectivity, different solvents (MeOH, THF, CH3CN, 1,4-dioxane and DMF) were tried. To our delight, DMF provided the excellent regioselectivity (9
:
1) at 60 °C by using Cs2CO3 as a base (Scheme 4). The yields are good in case of MBHAs possessing substituents at meta and para position (4b–4f) compared to MBHAs having substitutions at ortho positions (4g and 4h).
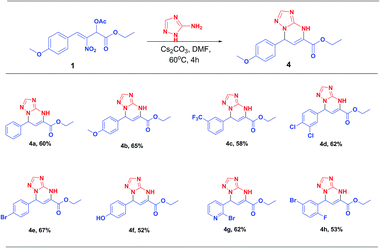 |
| Scheme 4 Synthesis of dihydro triazopyrimidine derivatives. | |
After the successful demonstration of the reactivity of different MBHAs with 3-amino-1,2,4-triazole, we focused our attention for the synthesis of 7-phenyl-4,7-dihydrotetrazolo[1,5-a]pyrimidine-5-carboxylic esters (5a and 5b) by reacting 5-amino tetrazoles with different MBHAs and are summarised in Scheme 5. The above conditions were, not suitable for reacting MBHAs with guanidine hydrochloride. Thus, there is no reaction was observed even after 12 h reflux when guanidine hydrochloride was treated with MBH acetates.
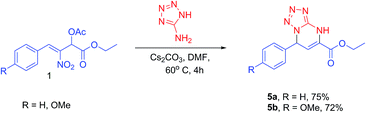 |
| Scheme 5 Synthesis of dihydro tetrazolopyrimidine derivatives follows. | |
The structures of all compounds were confirmed by LCMS, 1H NMR and 13C NMR spectral data. The compounds 3e (CCDC 1446736) and 4b (CCDC 1456725) were confirmed by single crystal X-ray diffraction studies and were shown in Fig. 3.
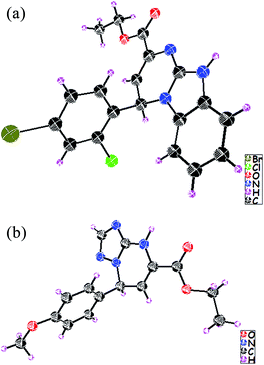 |
| Fig. 3 ORTEP diagram of (a) compound 3e and (b) compound 4b. | |
A possible mechanism for the synthesis is described in Scheme 6. The primary amine of 2-amino benzimidazole attacks the γ electrophilic site of MBH acetate through SN2 reaction to afford intermediate I. Intramolecular Michael addition occurs at α electrophilic site of MBH acetate to generate intermediate II via 6-endo trig process. The intermediate II results in formation of III by loss of hydrogen atom, which on tautomerisation gives IV. The excess base facilitates the elimination of HNO2 to give dihydro-benzo[4,5]imidazo[1,2-a]pyrimidine-2-carboxylic ester as 3. Thus the reaction is completed by formal γ–α cyclisation on MBH acetate.18,20 Reaction of N-methyl 2-amino-benzimidazole with MBH acetate shows very low conversion for the desired product with nearly 10% product formation. Reaction with N-benzyl and N-Boc derivative of amino-benzimidazole resulted in recovery of the starting material, with no trace of product formation even at 70 °C. This can be easily anticipated due to steric hindrance by the nucleophilic nitrogen to attack at the elctrophilic sp3 carbon atom of MBH acetate.
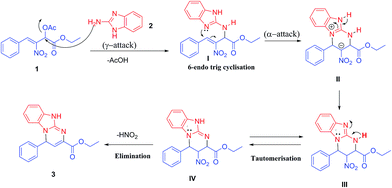 |
| Scheme 6 Proposed mechanism. | |
Biology
The in vitro inhibitory effect of newly synthesized ligands were assessed by Ellman's method22 using AMPLITE™ AChE assay kit (AAT Bioquest, Inc., Sunnyvale, CA). In order to check the inhibitory activity of the compounds towards the AChE an initial screening has been carried out with a ligand concentration of 208 nM. Enzyme and ligands were incubated for 15 minutes and checked the enzyme activity towards the substrate. A control experiment has also been carried out without ligand. Finally the optical density was plotted against time (velocity time graph) (Fig. 4) and deduced the percentage of inhibition of the compounds (Table 2). Since all the compounds were displayed strong inhibitory activity, the half maximal inhibitory activity (IC50) value for all compounds were deduced. Enzyme inhibition studies revealed that the newly synthesized compounds exhibit inhibitory activity against AChE in nM range. All of these compounds displayed enhanced inhibitory profile than tacrine and reported IC50 of galantamine (two known AD drugs).23 The maximum and minimum IC50 values obtained are 91.8 and 42.52 nM respectively. The compound 3a exhibited an IC50 value 70.78 nM. It was also noticed that the phenyl group baring any substitutions are found to have lower IC50 value except 3c. The presence of methyl group in the phenyl ring of the compound 3c′ has drastically increased the IC50 value to 91.8 nM. With the substitution of polar groups such as –OH (3h) and –NO2 (3j), the AChE inhibitory effects was better as compared with 3a. Introduction of halogens in phenyl moiety exerted prominent AChE inhibition (IC50 values in the range of 42–53 nM) of the compounds such as 3b, 3d, 3e and 3i. It was already reported that the substitution of halogen is an important method in drug design since it can increase the binding affinity of the compounds through halogen bonding and alters the physico chemical properties.24 Substitution of an additional halogen in the phenyl ring of the compounds 3d and 3e make it more effective against AChE with IC50 values 46.86 and 42.52 nM respectively (Fig. 5). Whereas the substitution of phenyl ring with pyridine ring (3g) has markedly decreased the inhibitory effect towards AChE. The IC50 values of all compounds are shown in Table 2.
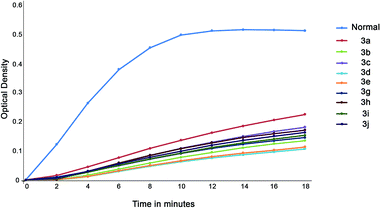 |
| Fig. 4 Velocity time graph obtained for native enzyme and in presence of compounds (208 nM). | |
Table 2 The in vitro AChE inhibitory profile of compounds 3a–3j
Compound |
% of inhibition at 208 nM |
hAChE IC50 (nM) + SD |
3a |
53 |
70.78 ± 10.01 |
3b |
70 |
52.64 ± 1.07 |
3c |
62 |
91.8 ± 3.6 |
3d |
76 |
46.86 ± 1.16 |
3e |
74 |
42.52 ± 5.17 |
3g |
69 |
71.48 ± 5.04 |
3h |
61 |
67.32 ± 4.94 |
3i |
67 |
52.58 ± 15.65 |
3j |
65 |
68.4 ± 7.94 |
Tacrine |
66 |
551.58 ± 19.17 |
Galanthamine |
|
360 ± 10 |
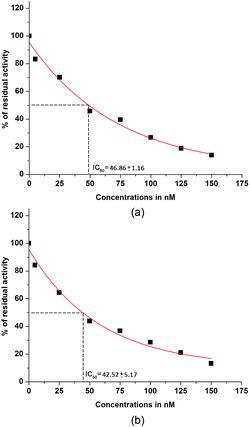 |
| Fig. 5 Relative residual activity of AChE plotted against the different concentrations of the most potent compounds 3d (a) and 3e (b). | |
The newly synthesized compounds were evaluated for their drug like behavior through in silico predictions. The ADME profiles of the compounds are given in Table 3. Some of the important parameters [molecular weight (MW) and volume (V), CNS activity, partition coefficient (log
P), number of hydrogen bond donors and acceptors (HBD and HBA), polar surface area (PSA), number of rotatable groups (rotor), number of N and O (N and O), BBB permeability (log
BB), permeability across Mandin–Darby canine kidney, (MDCK) and intestinal epithelial cells (Caco-2), solvent accessible surface area (SASA) and % of human oral absorption (% HOA)] that are crucial for CNS activity were identified and displayed in Table 3 along with the reference values.25,26
Table 3 ADME profile of the compounds predicted by QikProp program
Compound |
MW (≤450 Da) |
HBD (≤3) |
HBA (≤7) |
log P (≤5) |
CNS (≥0) |
Rotor (≤7) |
N and O (≤7) |
3a |
319 |
1 |
3 |
4.35 |
0 |
2 |
5 |
3b |
398 |
1 |
3 |
4.92 |
0 |
2 |
5 |
3c |
333 |
1 |
3 |
4.66 |
0 |
2 |
5 |
3d |
416 |
1 |
3 |
5.01 |
0 |
2 |
5 |
3e |
433 |
1 |
3 |
5.32 |
0 |
2 |
5 |
3g |
417 |
1 |
4 |
4.41 |
0 |
2 |
6 |
3h |
335 |
2 |
4 |
3.58 |
−2 |
3 |
6 |
3i |
337 |
1 |
3 |
4.53 |
0 |
2 |
5 |
3j |
348 |
1 |
5 |
3.51 |
−2 |
3 |
7 |
Compound |
BBB (−1.2 to 1.2) |
SASA (320–735 Å2) |
Volume (≤1250 Å3) |
PSA (60–70 Å2) |
Caco (≥500 nm s−1) |
MDCK (≥500 nm s−1) |
% HOA |
3a |
−0.51 |
605 |
1052 |
64 |
1171 |
587 |
100 |
3b |
−0.35 |
634 |
1105 |
64 |
1171 |
1554 |
100 |
3c |
−0.54 |
637 |
1112 |
64 |
1171 |
587 |
100 |
3d |
−0.27 |
641 |
1118 |
64 |
1170 |
2391 |
100 |
3e |
−0.22 |
649 |
1141 |
63 |
1174 |
3126 |
100 |
3g |
−0.51 |
634 |
1106 |
76 |
675 |
1362 |
100 |
3h |
−1.12 |
618 |
1077 |
86 |
355 |
161 |
94 |
3i |
−0.43 |
612 |
1065 |
64 |
1169 |
899 |
100 |
3j |
−1.16 |
620 |
1090 |
96 |
319 |
144 |
92 |
Using these physicochemical properties it is possible to differentiate the CNS drugs from a group of molecules. It was found that the values for MW, volume, N and O, rotor, HBD and HBA were in the preferred range for all the compounds. The lipophilicity (derived from log
P) of molecules are in the allowed limits except 3d and 3e. It was already reported that CNS acting agents may have slightly higher log
P value.8 PSA is always linked with BBB penetration and normally for CNS active molecules the PSA values will be low compared to other therapeutics.27 For majority of the compounds, the PSA value is optimal except 3g, 3h and 3j. The upper limit of PSA for the BBB permeability was reported as 90 Å2, and hence the two compounds such as 3g and 3h can also be taken into consideration.28 The SASA of all compounds are seems to be in the range of CNS acting agents. The polar groups containing compounds such as 3h and 3j has very poor BBB, MDCK cell permeability, Caco cell permeability and CNS activity. MDCK and Caco cell lines are used as in vitro models to study the BBB permeability.29 The online BBB prediction tool also gave the similar results. It showed all compounds except 3h and 3j have the ability to cross BBB.
The conclusion made from the enzyme kinetics studies was further explored with the help of molecular docking followed by binding energy calculations. The close analysis of the AChE–ligand complexes revealed a common binding pattern for all the newly synthesized ligands. They binds at the bottom of the active site, spanning along the acyl binding pocket and makes contacts with peripheral anionic site residues also. In all complexes, the aromatic residues such as F297, F295, F338, Y337, Y341 and H447 are oriented perpendicular to the dihydrobenzimidazo pyrimidine ring and arrests the rotational and translational movement of the ligands in the active site. Apart from these, hydroxyl group of Y337 and Y124 are acting as an anchor to hold the compounds at its current positions through a series of hydrogen bonds with keto group and ring nitrogens. A classical π–π stacking interaction was also observed between the phenyl ring of the compounds and the indole ring of W86. Furthermore, π–π stacking between dihydrobenzimidazo pyrimidine ring system and H447 an F338 was also observed. Docking studies also showed that the compounds 3d and 3e were more potent inhibitors than other compounds with binding energies −87.03 and −92.26 kcal mol−1 respectively. These compounds forms additional hydrogen bonds with keto group and Y341. They also exhibit halogen bonds with the polar atoms of the following residues such as Y119, G120, G121, S125 and Y133. Apart from that a series of van der Waals interactions and hydrophobic interactions are also found to stabilize the interactions. The residue such as Y72, V73, W86, Y124, Y133, F295, F297, Y337, F338, Y341 and I451 seems to be involved in the hydrophobic interactions. Whereas in case of tacrine, the interactions are mainly favoured by stacking formed against Y337 and W86. A hydrogen bond with N atom and main chain carbonyl oxygen of H447 is also seen. It also forms a series of hydrophobic interactions like the compounds 3d/3e. But it lacks halogen bonding and other hydrogen bonding interactions as seen in case of 3d and 3e. These interactions may be partly contributing to the highest inhibitory potency of the compounds over tacrine. The binding mode of the top scored ligands (3d and 3e) was displayed in Fig. 6. The binding energies of all the compounds are mentioned in Table 4.
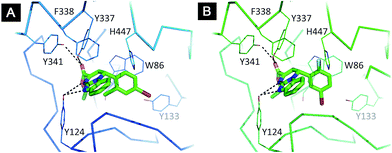 |
| Fig. 6 Binding pattern of compounds 3e (A) and compound 3d (B) at the active site of hAChE. The protein residues are shown in lines and the compounds are shown in stick. The hydrogen bonds are indicated by dotted lines. | |
Table 4 The binding energetics of the compounds at the active site of hAChE
Comp. |
Glide score |
Binding energy |
Interacting residues |
3a |
−8.24 |
−74.38 |
F338, H447, Y337 and W86 |
3b |
−8.62 |
−75.55 |
F338, H447, Y337, Y124 and W86 |
3c |
−8.09 |
−72.60 |
F338, H447, Y124, Y337 and W86 |
3d |
−9.15 |
−87.03 |
F338, H447, Y341, Y124, Y337 andW86 |
3e |
−9.79 |
−92.26 |
F338, H447, Y341, Y337, Y124 and W86 |
3g |
−8.70 |
−85.55 |
F338, Y124, Y337 and W86 |
3h |
−9.11 |
−75.07 |
F338, Y124, Y133, G121 and W86 |
3i |
−8.90 |
−76.65 |
F338, H447, Y124, Y337 and W86 |
3j |
−9.04 |
−74.13 |
F338, H447, Y124, Y337 and W86 |
Galanthamine |
−9.69 |
−81.51 |
F338, S203, E202 and Y337 |
In general, the effective AChE inhibition of the compounds are achieved by interaction with the residues in the peripheral anionic site (Y72, Y124 and Y341), anionic site (W86 and Y337), acyl binding site (F295 and F297) and active site (H447). Also the orientation of these compounds in the ligand binding site is in such a manner that it completely mask the entry of the substrate in to the active site. Also it can hinder H447 and make it unavailable for the catalytic activities. Stability of the compounds (3d and 3e) at their bound positions was investigated through 20 ns MD simulation. During the simulation, the intermediate structures were saved and superimposed to the native structure with respect to the ligand position. The RMSD indicates that none of the complex deviates much from their respective binding positions. The maximum deviation observed for ligand was 1.4 Å and the minimum was 0.6 Å. The studies indicate that the ligands are stable in their binding position. The RMSD of two top scored compounds 3d and 3e were displayed in Fig. 7.
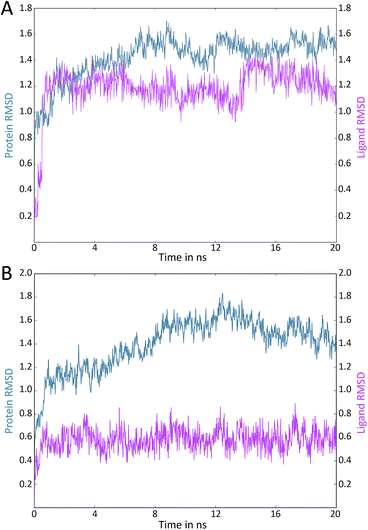 |
| Fig. 7 The RMSD graph of Cα (blue in colour), ligand displacement (pink in colour) of compound 3d (A) & compound 3e (B). | |
Conclusions
In summary, we have carried out a regioselective (γ–α) attack of bifunctional nucleophiles to MBH acetates. Various bifunctional nucleophiles such as 2-aminobenzimidaole, 2-aminoimidazole and 3-amino 1,2,4 triazole on reaction with MBH acetate gave moderate to excellent yields (45–82%) of dihydroazo pyrimidine derivatives. Interestingly, of all the potential elctrophilic sites (α, β, γ, δ) the first Michael addition occurs at γ position of MBH acetates in a SN2 followed by another nucleophilic attack at α position with a 6-endo trig cyclisation to give rise to functionalised dihydroazo pyrimidine derivatives. The in vitro enzyme inhibition studies of these derivatives indicate that they exhibits much higher potency than the known drugs such as tacrine and galantamine. The above results describes the importance of MBHAs in the synthesis of dihydroazo pyrimidine derivatives and their possible utilization against AD treatment as AChE inhibitors.
Experimental section
Experimental procedure for synthesis of compound (3a–3l)
To a solution of 2-aminobenzimidazole (0.15 mmol) in CH3CN (1.0 mL) was added MBH acetate of nitro alkene (0.18 mmol) and Cs2CO3 (0.3 mmol) at RT. The reaction mixture was stirred for 12 h at RT. After completion of reaction (monitored by LCMS), the reaction mixture was quenched with water (5 mL). The reaction mixture was extracted with ethyl acetate (3 × 30 mL). The combined organic layers were dried over anhydrous sodium sulphate, filtered and concentrated to give the crude product. To this crude product was added methanol (4 mL) and stirred for 30 min at RT. A white precipitate was obtained which was filtered to give the desired product (60–82%).
Enzyme inhibition studies
The in vitro inhibitory effect of newly synthesized ligands were assessed by Ellman's method using AMPLITE™ AChE assay kit (AAT Bioquest, Inc., Sunnyvale, CA). The assay system consists of AChE from electric eel (EC 3.1.1.7), 5,5-dithiobis-(2-nitrobenzoic acid) (DTNB, known as Ellman's reagent) and acetylcholine. The assay procedure is as follows, an aliquots (total volume 100 μL) was prepared by mixing 5.7 nM AChE (prepared in 0.1% of BSA containing distilled water) with acetylcholine (500 μM) and DTNB in a reaction buffer of pH 7.4. As a result of enzyme substrate interaction, the yellow colour formation was monitored and measured at 405 nm using a spectrophotometer at a time interval 2 minutes each for 20 minutes. Later the optical density was plotted against time. The same experiment (in triplicate) was repeated by incubating (15 minutes) the enzyme with different ligands. The relative activities of all ligands were expressed and compared with that of native enzyme activity. Finally the half maximal inhibitory concentration (IC50) was determined for each ligands and compared with a known inhibitor tacrine.
Molecular modelling studies
The physico chemical properties, atomic level of interactions and binding stability of newly synthesized compounds were predicted using different molecular modelling experiments. Initially the ligands (drawn using Chemsketch program) were prepared by correcting the bond lengths and bond angles followed by energy minimization using LigPrep module with OPLS-3 force field. Different tautomers of ligands were also generated and used for different studies. By using the module QikProp 4.7, the physico chemical, ADME (Absorption, Distribution, Metabolism and Excretion), lipinski rule of five, in silico BBB penetrability and CNS activity of newly synthesized ligands were predicted. The BBB permeability of the ligands were reasserted using a bayesian statistical model implemented in an online tool ‘B3PP 09’ (http://www.b3pp.lasige.di.fc.ul.pt). As a part of investigating the atomic level of interaction of newly synthesized ligands with human AChE a molecular modeling studies was carried out. Crystal structure of hAChE complex with galanthamine (4EY6) was downloaded from protein databank (PDB) and prepared by deleting all the crystallographic water molecules and adding hydrogen. The protonation states and partial charges were assigned for the charged residues during preparation. Finally the prepared structure was optimized and minimized. Later the ligand binding site was mapped by taking galanthamine as an axis point. Further the docking studies were performed between the prepared ligands and protein using extra precision (XP) mode of glide. The binding free energies of the ligand enzyme complex were also deduced using Prime MM-GBSA method.
The correlation between experimental IC50 values and theoretical binding energies were identified and highly active complex were selected for molecular dynamics (MD) simulation studies to predict the stability of the complex. The enzyme ligand complexes were prepared for MD simulation by soaking the complex in to an orthorhombic solvent box (volume 508
093 Å3) containing 13
100 TIP3P water molecules. The whole system was neutralized by adding 7 Na+ ions. Total simulation time lasts around 20 ns. The intermediate structures were saved at every 10 ps and superimposed to the native structure and deduced the RMS deviation. Finally a graph was plotted with RMS deviation against time.
Acknowledgements
SA thanks DST-SERB for financial support under young scientist scheme (SB/FT/CS-079/2014). EKR and AMS thanks Syngene. RC thanks Indian Council of Medical Research for the Senior Research Fellowship and KVD thanks IISER for the institute post-doctoral fellowship. The authors thanks IISc for providing single crystal X-ray diffraction data, and the authors gratefully acknowledge the ‘Bioinformatics Infrastructure Facility’ (supported by DBT, Govt. of India) located at the department of Biotechnology and Microbiology, Kannur University for providing the computational works. Authors RC and S.C acknowledge the financial support of Indian Council of Medical Research in the form of project (No. BIC/12(23)/2012).
References
-
(a) N. Margiotta, R. Ostuni, R. Ranaldo, N. Denora, V. Laquintana, G. Trapani, G. Liso and G. Natile, J. Med. Chem., 2007, 50, 1019 CrossRef CAS PubMed;
(b) J.-B. Veron, H. Allouchi, C. E. Gueiffier, R. Snoeck, G. Andrei, E. De Clercq and A. Gueiffier, Bioorg. Med. Chem., 2008, 16, 9536 CrossRef CAS PubMed;
(c) C. Enguehard-Gueiffier and A. Gueiffier, Mini-Rev. Med. Chem., 2007, 7, 888 CrossRef CAS PubMed.
-
(a) T. S. Harrison and G. M. Keating, CNS Drugs, 2005, 19, 65 CrossRef CAS;
(b) S. M. Hanson, E. V. Morlock, K. A. Satyshur and C. Czajkowski, J. Med. Chem., 2008, 51, 7243 CrossRef CAS PubMed;
(c) J. M. Monti, D. Warren Spence, S. R. Pandi-Perumal, S. Z. Langer and R. Hardeland, Clin. Med.: Ther., 2009, 1, 123 CAS.
-
(a) M. Masaki, T. Yamakawa, Y. Nomura and H. Matsukura, US Pat., US5576341, 1996;
(b) K. G. Desai and K. R. Desai, Bioorg. Med. Chem., 2006, 14, 8271 CrossRef CAS PubMed;
(c) Z. Kazimierczuk, M. Andrzejewska, J. Kaustova and V. Klimesova, Eur. J. Med. Chem., 2005, 40, 203 CrossRef CAS PubMed.
- A. M. Sajith, K. K. Abdul Khader, N. Joshi, M. Nageswar Reddy, M. Syed Ali Padusha, H. P. Nagaswarupa, M. Nibin Joy, Y. D. Bodke, R. P. Karuvalam, R. Banerjee, A. Muralidharan and P. Rajendra, Eur. J. Med. Chem., 2015, 89, 21 CrossRef CAS PubMed.
-
(a) W. R. Tully, C. R. Gardner, R. J. Gillespie and R. Westwood, J. Med. Chem., 1991, 34, 2060 CrossRef CAS PubMed;
(b) S. Clements-Jewery, G. Danswan, C. R. Gardner, S. S. Matharu, R. Murdoch, W. Roger Tully and R. Westwood, J. Med. Chem., 1988, 31, 1220 CrossRef CAS PubMed.
-
(a) K. B. Puttaraju, K. Shivashankar, C. M. Mahendra, V. P. Rasal, P. N. Venkata Vivek, K. Rai and M. B. Chanu, Eur. J. Med. Chem., 2013, 69, 316–322 CrossRef CAS PubMed;
(b) M. Di Braccio, G. Grossi, M. G. Signorello, G. Leoncini, E. Cichero, P. Fossa, S. Alfei and G. Damonte, Eur. J. Med. Chem., 2013, 62, 564 CrossRef CAS PubMed.
-
(a) E. D. Jones, N. Vandegraaff, G. Le, N. Choi, W. Issa, K. Macfarlane, N. Thienthong, L. J. Winfield, J. A. V. Coates, L. Lu, X. Li, X. Feng, C. Yu, D. I. Rhodes and J. J. Deadman, Bioorg. Med. Chem. Lett., 2010, 20(19), 5913–5917 CrossRef CAS PubMed;
(b) H. Zhi, L. M. Chen, L. L. Zhang, S. J. Liu, D. C. C. Wan, H. Q. Lin and C. Hu, ARKIVOC, 2008, 266–277 CAS;
(c) A. Rivkin, S. P. Ahearn, S. M. Chichetti, Y. R. Kim, C. M. Li, A. Rosenau, S. D. Kattar, J. Jung, S. Shah, B. L. Hughes, J. L. Crispino, R. E. Middleton, A. A. Szewczak, B. Munoz and M. S. Shearman, Bioorg. Med. Chem. Lett., 2010, 20, 1269–1271 CrossRef CAS PubMed;
(d) W. S. Messer Jr, W. G. Rajeswaran, Y. Cao, H. J. Zhang, A. A. el-Assadi, C. Dockery, J. Liske, J. O'Brien, F. E. Williams, X. P. Huang, M. E. Wroblewski, P. I. Nagy and S. M. Peseckis, Pharm. Acta Helv., 2000, 74, 135–140 CrossRef CAS;
(e) R. M. Kypta, Expert Opin. Ther. Pat., 2005, 15, 1315–1331 CrossRef CAS;
(f) K. R. Valasani, M. O. Chaney, V. W. Day and S. S. D. Yan, Pharmacophore, 2013, 53, 2033–2046 CAS;
(g) J. Liu, J. Qiu, M. Wang, L. Wanga, L. Su, J. Gaob, Q. Gua, J. Xua, S.-L. Huang, L.-Q. Gu, Z.-S. Huang and D. Li, Biochim. Biophys. Acta, 2014, 1840, 2886–2903 CrossRef CAS PubMed;
(h) H. Zhi, L. M. Chen, L. L. Zhang, S. J. Liu, D. C. C. Wan, H. Q. Lin and C. Hu, Chem. Res. Chin. Univ., 2009, 25, 332–337 CAS;
(i) A. S. Alpan, S. Parlar, L. Carlino, A. H. Tarikogullari, V. Alptuzun and H. S. Gunes, Bioorg. Med. Chem., 2013, 21, 4928–4937 CrossRef CAS PubMed;
(j) J. Zhu, C. F. Wu, X. Li, G. S. Wu, S. Xie, Q. N. Hu, Z. Deng, M. X. Zhu, H. R. Luo and X. Hong, Bioorg. Med. Chem., 2013, 21, 4218–4224 CrossRef CAS PubMed;
(k) Y. K. Yoon, M. A. Ali, A. C. Wei, T. S. Choon, K.-Y. Khaw, V. Murugaiyah, H. Osman and V. H. Masand, Bioorg. Chem., 2013, 49, 33–39 CrossRef CAS PubMed.
-
(a) Y.-H. Suh and F. Checler, Pharmacol. Rev., 2002, 54, 469–525 CrossRef CAS PubMed;
(b) V. Tumiatti, A. Minarini, M. L. Bolognesi, A. Milelli, M. Rosini and C. Melchiorre, Curr. Med. Chem., 2010, 17, 1825–1838 CrossRef CAS PubMed;
(c) D. M. Walsh and D. J. Selkoe, Neuron, 2004, 44, 181 CrossRef CAS PubMed.
-
(a) K. Morita, Z. Suzuki and H. Hirose, Bull. Chem. Soc. Jpn., 1968, 41, 2815 CrossRef CAS;
(b) A. B. Morita and M. E. D. Hillman, Ger. Offen., DE 2155113, 1972Chem. Abstr., 1972, 77, 434174.
- Reviews on MBH and related reactions:
(a) D. Basavaiah and G. Veeraraghavaiah, Chem. Soc. Rev., 2012, 41, 68 RSC;
(b) D. Basavaiah, B. S. Reddy and S. S. Badsara, Chem. Rev., 2010, 110, 5447 CrossRef CAS PubMed;
(c) R. Rios, Catal. Sci. Technol., 2012, 2, 267 RSC;
(d) V. Declerck, J. Martinez and F. Lamaty, Chem. Rev., 2009, 109, 1 CrossRef CAS PubMed;
(e) V. Singh and S. Batra, Tetrahedron, 2008, 64, 4511 CrossRef CAS;
(f) Y. Wei and M. Shi, Chem. Rev., 2013, 113, 6659 CrossRef CAS PubMed.
-
(a) K. Morita, Z. Suzuki and H. Hirose, Bull. Chem. Soc. Jpn., 1968, 41, 2815 CrossRef CAS;
(b) M. E. D. Hillman and A. B. Baylis, US Pat., US 4743669, 1973.
-
(a) I. Deb, M. Dadwal, S. M. Mobin and I. N. N. Namboothiri, Org. Lett., 2006, 8, 1201 CrossRef CAS PubMed;
(b) I. Deb, P. Shanbhag, S. M. Mobin and I. N. N. Namboothiri, Eur. J. Org. Chem., 2009, 4091 CrossRef CAS;
(c) H. Kuan, R. J. Reddy and K. Chen, Tetrahedron, 2010, 66, 9875 CrossRef CAS.
-
(a) E. Gopi and I. N. N. Namboothiri, J. Org. Chem., 2014, 79, 7468 CrossRef CAS PubMed;
(b) L. F. Yeh, S. Anwar and K. Chen, Tetrahedron, 2012, 68, 7317 CrossRef CAS;
(c) C. L. Cao, Y. Y. Zhou, J. Zhou, X. L. Sun, Y. Tang, Y. X. Li, G. Y. Li and J. Sun, Chem.–Eur. J., 2009, 15, 11384 CrossRef CAS PubMed.
-
(a) H. Cao, T. O. Vieira and H. Alper, Org. Lett., 2011, 13, 11 CrossRef CAS PubMed;
(b) S. Rajesh, B. Banerji and J. Iqbal, J. Org. Chem., 2002, 67, 7852 CrossRef CAS PubMed;
(c) B. Singh, A. Chandra and R. M. Singh, Tetrahedron, 2011, 67, 2441 CrossRef CAS;
(d) H. P. Deng, Y. Wei and M. Shi, Eur. J. Org. Chem., 2011, 10, 1956 CrossRef;
(e) Y. Guo, G. Shao, H. Zeng, L. Li, W. Wu, R. Li, J. Li, J. Song, L. Qiu, M. Prashad and F. Y. Kwong, Adv. Synth. Catal., 2010, 352, 1539 CrossRef CAS;
(f) S. Q. Ge, Y. Y. Hua and M. Xia, Synth. Commun., 2010, 40, 1954 CrossRef CAS.
-
(a) S. Anwar, W.-Y. Huang, C.-H. Chen, Y.-S. Cheng and K. Chen, Chem.–Eur. J., 2013, 19, 4344 CrossRef CAS PubMed;
(b) T. Kumar, S. M. Mobin and I. N. N. Namboothiri, Tetrahedron, 2013, 69, 4964 CrossRef CAS;
(c) V. Mane, T. Kumar, S. Pradhan, S. Katiyar and I. N. N. Namboothiri, RSC Adv., 2015, 5, 69990 RSC.
- D. K. Nair, S. M. Mobin and I. N. N. Namboothiri, Org. Lett., 2012, 14, 4580 CrossRef CAS PubMed.
-
(a) T. Kumar, D. Verma, R. F. S. Menna-Barreto, W. O. Valenca, E. N. da Silva Junior and I. N. N. Namboothiri, Org. Biomol. Chem., 2015, 13, 1996 RSC;
(b) E. Gopi, T. Kumar, R. F. S. Menna-Barreto, W. O. Valenca, E. N. D. Silva Junior and I. N. N. Namboothiri, Org. Biomol. Chem., 2015, 13, 9862 RSC.
- J.-Q. Zhang, J.-J. Liu, C.-L. Gu, D. Wang and L. Liu, Eur. J. Org. Chem., 2014, 5885 CrossRef CAS.
- D. K. Nair, S. M. Mobi and I. N. N. Namboothiri, Tetrahedron Lett., 2012, 53, 3349 CrossRef CAS.
- N. Shao, T. Chen, T. zhang, H. Zhu, Q. Zheng and H. Zou, Tetrahedron, 2014, 70, 795 CrossRef CAS.
- H. Zhu, N. Shao, T. Chen and H. Zou, Chem. Commun., 2013, 49, 7738 RSC.
- G. L. Ellman, K. D. Courtney, V. Andres Jr and R. M. Featherstone, Biochem. Pharmacol., 1961, 7, 88 CrossRef CAS PubMed.
- C. Guillou, A. Mary, D. Z. Renko, E. Gras and C. Thal, Bioorg. Med. Chem. Lett., 2000, 10(7), 637 CrossRef CAS PubMed.
- P. W. Codding, Halogen-substituted Drugs and their Intermolecular Interactions, Acta Crystallogr., Sect. A: Found. Crystallogr., 2005, 61, C329 Search PubMed.
-
(a) H. Pajouhesh and G. R. Lenz, Medicinal chemical properties of successful central nervous system drugs, NeuroRx, 2005, 2, 541 CrossRef PubMed;
(b) M. S. Alavijeh, M. Chishty, M. Z. Qaiser and A. M. Palmer, Drug metabolism and pharmacokinetics, the blood–brain barrier, and central nervous system drug discovery, NeuroRx, 2005, 2, 554 CrossRef PubMed;
(c) A. K. Ghose, T. Herbertz, R. L. Hudkins, B. D. Dorsey and P. J. Mallamo, Knowledge-Based, Central Nervous System (CNS) Lead Selection and Lead Optimization for CNS Drug Discovery, ACS Chem. Neurosci., 2012, 3, 50 CrossRef CAS PubMed.
-
(a) D. E. Clark, Rapid calculation of polar molecular surface area and its application to the prediction of transport phenomena. 2. Prediction of blood–brain barrier penetration, J. Pharm. Sci., 1999, 88, 815 CrossRef CAS PubMed;
(b) G. R. Lenz, Technical problems in getting results, in From data to drugs: strategies for benefiting from the new drug discovery technologies, ed. A. B. Haberman, G. R. Lenz and D. E. Vaccaro, Scrip Reports, London, 1999, p. 95 Search PubMed.
-
(a) R. M. Feng, Assessment of blood-brain barrier penetration: in silico, in vitro and in vivo, Curr. Drug Metab., 2002, 3, 647 CrossRef PubMed;
(b) T. Sakaeda, N. Okamura, S. Nagata, T. Yagami, M. Horinouchi, K. Okumura, F. Yamashita and M. Hashida, Molecular and pharmacokinetic properties of 222 commercially available oral drugs in humans, Biol. Pharm. Bull., 2001, 24, 935 CrossRef CAS PubMed;
(c) J. Kelder, P. D. J. Grootenhuis, D. M. Bayada, L. P. C. Delbressine and J.-P. Ploemen, Polar molecular surface as a dominating determinant for oral absorption and brain penetration of drugs, Pharm. Res., 1999, 16, 1514 CrossRef CAS PubMed.
- M. A. Deli, C. S. Abraham, Y. Kataoka and M. Niwa, Permeability studies on in vitro blood–brain barrier models: physiology, pathology, and pharmacology, Cell. Mol. Neurobiol., 2005, 25, 59 CrossRef PubMed.
- A. Reichel, D. J. Begley and N. J. Abbott, An overview of in vitro techniques for blood–brain barrier studies, Methods Mol. Med., 2003, 89, 307 CAS.
Footnote |
† Electronic supplementary information (ESI) available. CCDC 1446736 and 1456725. For ESI and crystallographic data in CIF or other electronic format see DOI: 10.1039/c6ra12507g |
|
This journal is © The Royal Society of Chemistry 2016 |