DOI:
10.1039/C6RA12491G
(Paper)
RSC Adv., 2016,
6, 73210-73221
Oral delivery of quercetin to diabetic animals using novel pH responsive carboxypropionylated chitosan/alginate microparticles†
Received
13th May 2016
, Accepted 23rd July 2016
First published on 27th July 2016
Abstract
This study aims at the development of efficient, biocompatible, biodegradable and bio-safe polymeric carriers for investigating the pharmaceutical potentialities of bioflavonoid quercetin in antidiabetic research. In this article, two cost effective polymers, sodium alginate and a modified chitosan derivative (succinyl chitosan) were used for encapsulating quercetin through ionic crosslinking. In vitro biophysical characterizations like Fourier Transforms Infrared Spectroscopy (FT-IR), X-ray Diffraction (XRD), Scanning Electron Microscopy (SEM), swelling index, drug entrapment and loading efficiency were investigated. The prepared micro-formulations could efficiently encapsulate 94% quercetin and showed pH sensitive and self-sustained release of encapsulated quercetin, protecting it from the harsh environment and enzymatic deactivation in the gastrointestinal tract. An in vivo pharmacological study revealed a pronounced hypoglycemia effect in a diabetic rat model after peroral delivery of the quercetin microparticles in comparison to free quercetin. No acute systemic toxicity is evident following its oral administration in rats, ensuring it as an efficient carrier for oral quercetin carrier system in animal models.
1. Introduction
The global prevalence of diabetes mellitus is increasing at an alarming rate, affecting almost 285 million people and the number is estimated to double by 2030.1 This major socio-economic burden has a direct impact on developing countries due to poor control of glycemia and hypertension, trend of urbanization, lifestyle changes and most importantly the “Western-style” diet etc.2 To date, insulin therapy is the most effective treatment of diabetes although other oral hypoglycemic drugs such as sulfonylureas, α-glucosidase inhibitors, biguanides, and glinides,3 which are generally used as monotherapy or in combination to achieve better glycemic control and regulation. However, the cost of insulin therapy and adverse side effects of other treatments are not desirable. So, scientists are engaged in development of an alternative treatment with natural flavonoids to combat diabetes and its complications without compromising the overall health of the patients. Treatment of blood glucose abnormality with the use of ethnobotanicals has been in long practice since, the time of Charaka and Sushruta.4 Bio-flavonoids, (group of phenolic secondary plant metabolites) with multi-directional biological activities are widespread in nature (medicinal plants, fruits, vegetables) and have been explored for potentialities in the treatment of diabetes.5–8
In particular, quercetin (3,5,7-trihydroxy-2-(3,4-dihydroxyphenyl)-4H-chromen-4-one) (QUE) has gained considerable attention due to its beneficial properties, like antidiabetic, antioxidant, anticarcinogenic, antiradical, antiinflammatory, antimutagenic, antiangiogenic, antiviral, antibacterial, and antiaging effects.9,10 The oral delivery of QUE could be favourable to the diabetics, as it can minimize the inconvenience of regular injection insulin.10 However, being sparingly water soluble, chemically unstable in aqueous alkaline media, QUE is poorly absorbed in the intestine, with extensive fast-pass metabolism in gut and liver and its metabolites retain little biological activities following rapid elimination11,12 Therefore, to facilitate successful oral delivery of quercetin, development of polymeric carriers13 providing controlled release and prolong residence time within the intestinal lumen is required.
Recently, natural polymers (chitosan, pectin, galactomannan, alginate, guar gum, xanthan gum etc.) have become popular for myriad biomedical applications.14–16 Chitosan and alginate are very promising for cost effectiveness and have been widely subjected for controlling drug release.14 Chitosan (linear and partly acetylated (1 → 4)-2-amino-2-deoxy-β-D-glucan) and alginate have gained special attention due to their biocompatible, biodegradable, low-toxic, non-immunogenic and mucoadhesive nature.14,17–19 Presence of several functional groups (amino and hydroxyl groups) on chitosan makes it chemically active, facilitating effective encapsulation of bio-molecules like proteins and drugs.20 Again, alginate is also used for cell encapsulation, protein and drug delivery.9,21 Moreover, these polymers have the ability to adhere to the intestinal mucosa favouring perfect specific site localization of the dosage forms, leading to elevated oral bioavailability by reducing local irritation.22 However, poor water solubility of chitosan may limit its wide application in the biomedical field. Therefore, variety of water soluble pH responsive chitosan derivatives are investigated such as poly ethylene glycol modified chitosan (PEGylated chitosan),23 quaternized chitosan,24 oligoamine-conjugated-chitosan,25 trimethylated chitosan,26 polyamidoamine (PAMAM) conjugated chitosan16 etc. to evade the said problem.
In the present investigation, we have focused in the preparation of carboxypropionylated chitosan by introducing succinyl groups at the N-position of the glucosamine units of chitosan and development of pH responsive microparticles for sustained oral quercetin delivery, to evaluate its better efficiency in lowering blood glucose level as compared to free oral quercetin in diabetics. Carboxypropionylated chitosan has unique properties both in vitro and in vivo such as low toxicity, biocompatibility and long-term retention time in the body. It is an excellent drug carrier that can conjugates with many bio-molecules due to possessing –NH2 and –COOH groups. Moreover, due to presence of carboxylic group it exhibits pH sensitive swelling behaviour. In acidic pH drug molecules are trapped within the shrunken particles and get well protection from enzymatic reactions. Again, alginate is used for producing better pH sensitivity of the micro-formulations. All the advantages endowed this polymeric material with huge potential for successful site-specific or controlled-release drug delivery application. The flavonoid (quercetin) loading, encapsulation efficiency, swelling–deswelling behavior, release kinetics in simulated gastric and intestinal fluids and ex vivo mucoadhesion were also examined. The morphological observation under SEM and finally, in vivo hypoglycemic effects and systemic toxicity studies have been carried out to get an idea of the safety of the novel oral quercetin carrier system.
2. Experimental
For details please see the ESI.†30–40
3. Results and discussion
3.1. Characterization of polymeric samples
N-Succinyl chitosan was prepared via ring-opening reactions with succinic anhydride in dimethyl sulphoxide. The percentage of yield and degree of substitution were determined to be 82.5% and 0.67 using chitosan and succinic anhydride w/w ratio of 1
:
1. The polymer structure of chitosan and N-succinyl chitosan has been shown in Fig. 1. Free amino group of a representative chitosan reacts with the carbonyl carbon of succinic anhydride to produce a representative succinyl chitosan. In each reaction centre, a free amine group of D-glucosamine (repeating monomer unit of chitosan) reacts with succinic anhydride to produce succinyl chitosan and the possibility of such a reaction has been explored using quantum mechanical (QM) calculation. Interestingly, in the energy minimized D-glucosamine structure, the amine group orients perpendicularly to the plane framed by the sugar moiety. Thus, two possible reaction pathways are possible, first one is the pathway where chitosan attacks through above the plane (pathway 1) and the second one is through the reaction below the plane (pathway 2). Energetics of each of the reaction pathways were calculated from two different quantum chemical methods (AM1 and RM1) and are is tabulated in Table 1. As evident from the table, pathway 1 is more favourable compared to pathway 2, since compound 1 is sterically favoured over compound 2.
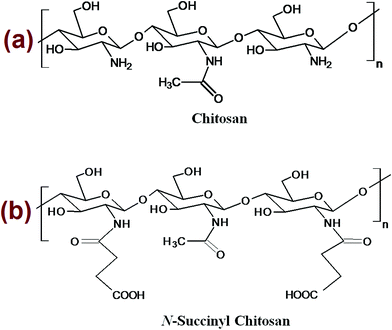 |
| Fig. 1 The polymer structures of (a) chitosan and (b) N-succinyl chitosan. | |
Table 1 Calculation of formation energy of the two possible reaction products during the reaction of succinic anhydride and chitosan
Comp name |
Method |
E (kcal mol−1) |
ΔE (kcal mol−1) |
Succinic anhydride |
AM1 |
−35 254.66 |
|
RM1 |
−34 803.21 |
|
Chitosan |
AM1 |
−63 610.99 |
|
RM1 |
−62 996.53 |
|
Compound 1 |
AM1 |
−98 885.28 |
−19.63 |
RM1 |
−97 818.21 |
−18.47 |
Compound 2 |
AM1 |
−98 882.63 |
−16.98 |
RM1 |
−97 815.27 |
−15.53 |
FT-IR analysis of the samples was carried out and the spectra are shown in Fig. 2. The basic characteristic peaks of chitosan are obtained at 3424 cm−1 (O–H stretch and N–H stretch, overlap), 2913 and 2856 cm−1 (C–H stretch), 1652 cm−1 (NH2 deformation), 1154 cm−1 (bridge-O-stretch) and 1068 cm−1 (C–O stretch) are shown in Fig. 2a.27 Fig. 2b shows the IR spectrum of N-succinyl chitosan (NSC), where after introduction of succinyl groups into native chitosan, the absorption bands of stretching vibration of the –OH and –NH2 (3300–3500 cm−1) have became narrower and were shifted to lower wave number. A new absorption band observed at 1408 cm−1 could be attributed to symmetric stretching of the –COO group indicating successful modification of native chitosan with succinic anhydride. Moreover, the peak at 1652 cm−1 (Amide I) of native chitosan increased and no absorption bands obtained at 1720–1750 cm−1, providing clear indication towards successful succinyl derivation at the N-position of chitosan and formation of –NH–CO– groups.28 Again, the peak at 1566 cm−1 (–NH2 bending) found to be decreased greatly, and a new signal appeared at 1552 cm−1 (assigned to the secondary amines) suggested substitution of the amino groups of chitosan. The spectrum of alginate (ALG) (Fig. 2c) presents the basic characteristic peaks at 1611 and 1428 cm−1; this could be assigned to the asymmetric and symmetric stretching of carboxylate salt groups. Additionally, a band around 1014 cm−1 (C–O–C stretching) could be denoted as its saccharide structure.41 For quercetin (QUE), the FT-IR spectrum (Fig. 2d) shows the characteristic intensities of –OH groups at 3408 cm−1, C
O absorption band at 1665 cm−1, C–C stretching bands at 1611 cm−1, C–H bending at 1450, 1382, and 823 cm−1; a band at 1262 cm−1 could be attributed to the C–O stretching of the oxygen in the ring, and the region for C–O stretching is found to be at 1014–1168 cm−1. All these results are quite consistent with previous investigations.13,42 From the IR spectrum of QUE-loaded NSC/ALG microparticles, it is observed that the asymmetric and symmetric stretching vibrations of quercetin either disappeared or became weak. This has occurred due to multi-interactions by between the carbonyl and the carboxyl groups of the bioflavonoid and the polymer, supporting the earlier investigations.13
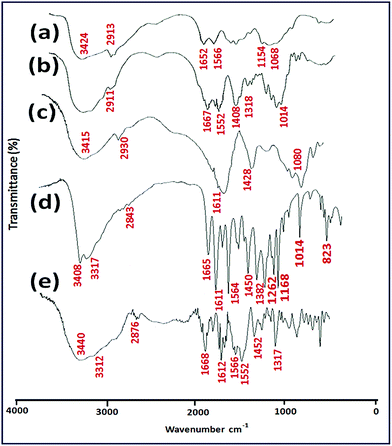 |
| Fig. 2 FT-IR spectra of (a) CS, (b) NSC, (c) ALG, (d) QUE and (e) QUE loaded NSC/ALG microparticles. | |
The X-ray diffraction (XRD) study was conducted to confirm the changes of chitosan structure before and after modification with succinic anhydride. The XRD profiles of the test samples are shown in Fig. 3. Chitosan has two distinct crystalline peaks, observed at around 10° and 20° compared to NSC (Fig. 3a). This can be explained by the fact that strong intermolecular and intramolecular hydrogen bonds between hydroxyl and amino groups of chitosan might support certain structural regularity of chitosan to form crystalline regions easily.29 However, in case of NSC, the peak at 10° disappeared and the peak at 20° significantly weakened as depicted in Fig. 3a. The results suggested that the destruction of the intermolecular hydrogen bonds and the crystalline regions of chitosan have occurred with successful derivation with succinic anhydride.28 The diffractogram of pure quercetin alone revealed its characteristic crystalline structure, showing multiple sharp peaks at different intensities between 2θ = 8° and 32° (Fig. 3b), which is consistent with previous reports.22,43
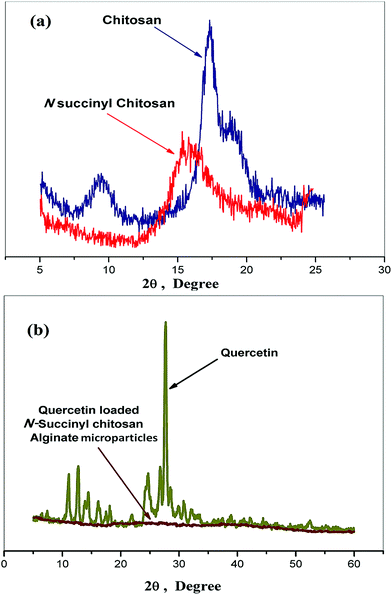 |
| Fig. 3 X-ray diffraction spectrum of (a) chitosan and its succinyl derivative, (b) quercetin and quercetin loaded microparticles. | |
On the other hand, from Fig. 3b the diffractogram of quercetin loaded microparticles was mostly dominated by the amorphous characteristics, indicating nearly complete disappearance of the crystalline peaks of quercetin. One explanation, it can inferred that quercetin has been either encapsulated or dispersed into carboxypropionylated chitosan/alginate polymeric microformulations when undergoing the physical crosslinking thus forming an amorphous complex with intermolecular interaction occurring within the polymer matrix. Our present study is also in close accordance with the similar phenomenon observed by Xing et al. (2007) for oridonin dispersed in poly (D,L-lactic acid) (PLA), where the XRD pattern of oridonin-loaded PLA nanoparticles showed that the drug was in its amorphous state.44 Hence, it can be confirmed that the quercetin was successfully loaded and it might exist dispersed in a non-crystalline state within the polymeric matrix.
3.2. Preparation of NSC/alginate microparticles and SEM study
The NSC/alginate polymeric microparticles were prepared by addition of polymeric blend of NSC and alginate into 2% calcium chloride (crosslinking solution). It is well known that microencapsulated microparticles can be prepared with alginate because it immediately gets ionically crosslinked in Ca2+ solution.29 Likewise, aqueous N-succinyl chitosan also forms gel like structure in calcium chloride solution.45 The gel formation suggested ionic crosslinking between the carboxylate ions (–COO−) on NSC established by Ca2+. Therefore, during the formation of NSC/alginate hydrogel microparticles, alginate gets entangled through the NSC polymeric network, resulting in interpenetrating polymeric network. These microparticles were further studied for oral quercetin delivery to diabetic animals.
Morphological study of blank and quercetin loaded NSC/alginate microparticles were investigated in this section. It can be observed in Fig. 4a–h, that the shape of microparticles was almost spherical with average particle size around 500 μm and the color of the test microparticles appeared to be stramineous (NSC
:
ALG, 1
:
1). After drying, the test samples had a rough surface with large wrinkles especially at a lower weight ratio of N-succinyl chitosan and alginate (0.5
:
1, 0.25
:
1). (The micrographs are not shown because of its irregular shape). Therefore, it can be confirmed that the weight ratio of N-succinyl chitosan and alginate could influence the morphology and the shape of the microparticles. The SEM images of blank hydrogel microparticles are illustrated in Fig. 4a–d and quercetin loaded particles are shown in Fig. 4e–h. As can be seen, the shape is apparently regular with rough surface morphology and cracks. Detailed surface examination (Fig. 4d and h) revealed cracks, wrinkles and somewhere inward dents that might be caused by partly collapsing the polymer network during in situ dehydration process.
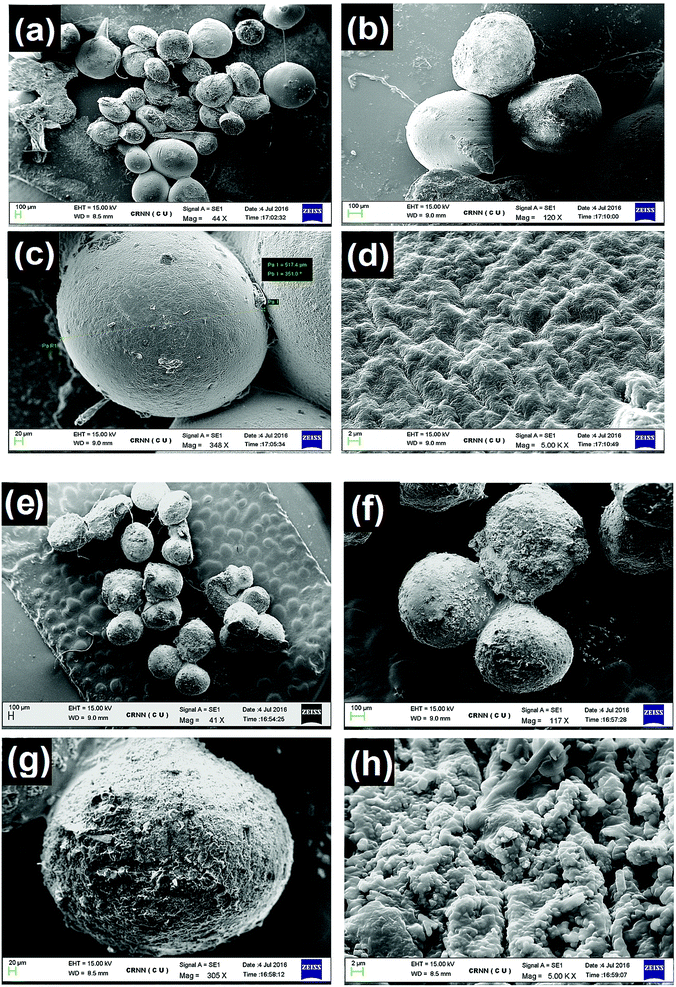 |
| Fig. 4 (a) and (b) SEM micrographs of NSC/ALG blank microparticles (low magnification), (c) SEM image showing a single blank microparticles, (d) SEM image of the surface of blank microparticles, (e) and (f) SEM micrographs of quercetin loaded NSC/ALG microparticles (low magnification), (g) SEM image showing a single quercetin loaded NSC/ALG microparticles, (h) SEM image of showing the surface morphology of quercetin loaded NSC/ALG microparticles. | |
The surface morphology of QUE loaded NSC/alginate microparticles showed more rough and irregular wrinkles and pores as compared to blank microparticles under scanning electron microscopy (Fig. 4h). Blending of NSC with alginate might have some molecular interaction in between them that could have influenced this type of surface morphology. Hence, it can be summarised from the SEM observation that the porous surface might help in better quercetin release from the microparticles after oral injection.
3.3. Swelling–deswelling behavior of quercetin loaded NSC/alginate microparticles and its modelling
The quercetin loaded NSC/alginate microparticles showed excellent reversible swelling in pH dependent manner. It is observed that in the simulated stomach pH 1.2, minimal swelling occurred whereas polymeric microparticles significantly swelled in simulated intestinal buffer, pH 7.4 as shown in Fig. 5a. The reversible swelling–deswelling behavior of these polymeric formulations can be attributed to the ionization of functional groups present on the polymeric chains. Remarkable swelling of the microparticles at intestinal pH (7.4) might occur due to generation of electrostatic repulsion between the ionized acid groups (–COO−) of the polymeric chains as depicted in Fig. 5b. Simultaneously, when the swollen microparticle samples were transferred to a buffer solution (pH 1.2), strong hydrogen bond formation started to occur between the (–COOH and –OH) of NSC and (–OH) of alginate; this might led to a gradual deswelling of the polymeric formulations (Fig. 5b). These results are quite comparable to earlier reports.29,46 Therefore, the evidence of progressive swelling–deswelling behavior of quercetin loaded NSC/alginate microparticles could facilitate the delivery of the bioflavonoid in a pH-responsive manner and protect it from enzymatic degradation through the gastrointestinal (GI) tract passage.
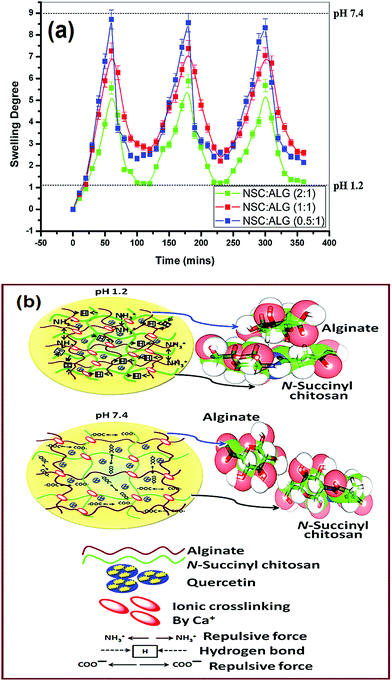 |
| Fig. 5 (a) Swelling–deswelling profile of the NSC/ALG microparticles at pH 1.2 and pH 7.4. (b) Schematic presentation of swelling and quercetin release mechanism of the microparticles. | |
To provide atomistic insight into the pH dependent swelling mechanism, modelling studies were carried out further as presented in Fig. 5b. At low pH 1.2, both the carboxyl group of the succinyl chitosan and alginate are protonated, thus the quantum mechanical optimized structure of the succinyl chitosan–alginate complex shows close association between succinyl chitosan and alginate, indicating a strong cross-linking possibilities (Fig. 5b). In fact, strong surface complementarities are observed in this complex driven primarily by van der Waals interactions. Formation energy of this succinyl chitosan–alginate complex is energetically favourable, evident from both RM1 and AM1 calculations (Table 2). Interestingly, when the carboxylic acidic groups of both the succinyl chitosan and alginate are deprotonated, i.e., exist as COO− form, the association between the succinyl chitosan and alginate are disrupted due to strong repulsion between the two COO− groups. Two COO− groups move opposite to each other such that the van der Waals interaction between succinyl chitosan and alginate is completely diminished as shown in Fig. 5b and energy of formation of that complex is highly unfavourable (Table 2).
Table 2 Calculation of formation energy of the Alginate-N-succinyl chitosan complex in pH 1.2 and pH 7.4 are summarized
Compound name |
Method |
E (kcal mol−1) |
ΔE (kcal mol−1) |
Alginate |
AM1 |
−72 666.71 |
|
RM1 |
−71 790.11 |
|
N-Succinyl chitosan |
AM1 |
−98 885.28![[thin space (1/6-em)]](https://www.rsc.org/images/entities/char_2009.gif) |
|
RM1 |
−97 818.21 |
|
Alginate-N succinyl chitosan complex (pH 1.2) |
AM1 |
−1 71 559.77 |
−7.78 |
RM1 |
−1 69 611.79 |
−3.47 |
Alginate-N succinyl chitosan complex (pH 7.4) |
AM1 |
−1 70 965.63 |
586.36 |
RM1 |
−1 68 981.13 |
627.19 |
3.4. Quercetin (QUE) loading and encapsulation efficiency
Successful oral drug delivery demands ideal carrier system with significant drug loading and encapsulation efficiency.29,47 After entrapment within the polymeric carriers, bioactive molecules (like enzymes, peptides, proteins and drugs) must remain biologically active to generate desired effects in animal system. Therefore, poor drug loading or encapsulation efficiency could effectively hinder the use of the polymeric formulations for oral drug delivery as it would result in wastage of expensive drug (QUE). The percentage of quercetin encapsulation and the loading within the polymeric microformulations are shown in Fig. 6a. It has been observed that the maximum quercetin loading and encapsulation efficiency were ∼55% and ∼94% respectively. Microparticles formulated with alginate alone have poor quercetin loading (∼17%) as well as encapsulation efficiency (∼37%). However, addition of carboxypropionylated chitosan with alginate in the formulation seemed to improve both the drug load and entrapment efficiency significantly (Fig. 6a). The quercetin encapsulation efficiency of N-succinyl chitosan/alginate microparticles revealed ∼94%. Since quercetin is not soluble in water drug, it was not dissolved in solution during the crosslinking process; in turn the loss of quercetin was minimal during the washing process. Again, the loading efficiency seemed to be significantly improved at various weight ratios of drug (quercetin) to polymer. As the weight ratio of polymer to drug increased (i.e. N-succinyl chitosan was added with alginate in the microparticle formulation), the contact probability of polymer and drug also increased significantly; which resulted in decreased amount of free drug and an increased amount of drug within the microparticle formulation. The result showed that the weight ratio of drug to polymer had an evident influence on the microparticle preparation method. Apart from the amount of polymer used in the preparation of microparticles, the concentration of the crosslinker has a pivotal role in influencing the drug encapsulation and loading. It is observed that increasing concentration of calcium chloride resulted in better quercetin entrapment but less QUE release from the particles owing to higher crosslinking density. In this study, we found significant encapsulation of quercetin and sustained release of the flavonoid from the particles using 2% calcium chloride solution as a crosslinking agent.
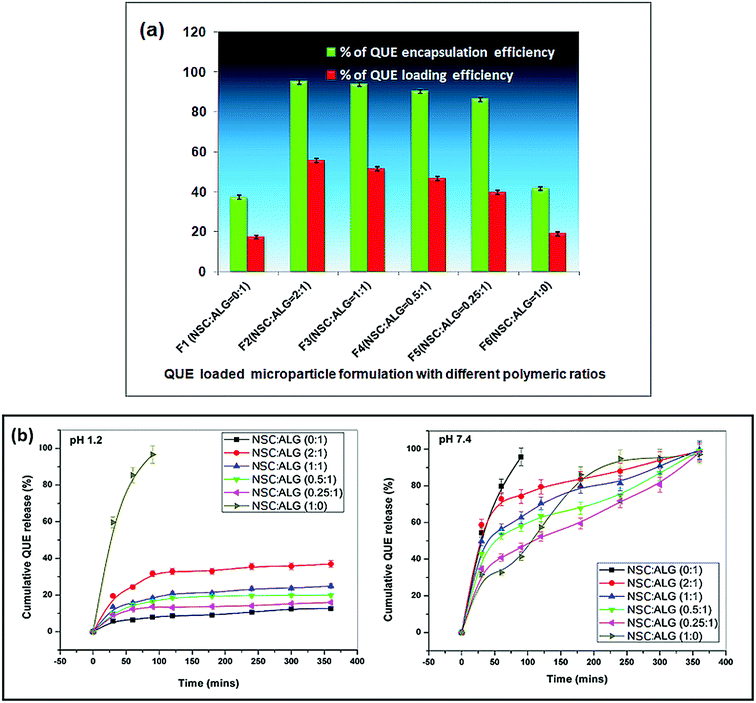 |
| Fig. 6 Comparative study of (a) the quercetin loading capacity and percentage of quercetin encapsulation of microparticles of different polymeric ratio (b) cumulative quercetin release profile of the microparticles samples at different pH values. | |
3.5. Quercetin release profile and kinetic modelling
The quercetin release profile from NSC/alginate microparticles follows the same trend as of the swelling behaviour of those formulations. All the samples efficiently retarded quercetin release at pH 1.2, retaining almost entire loaded amount of the drug as shown in Fig. 6b. Only ∼24% of encapsulated quercetin found to be released from microparticle formulations of NSC/alginate at this pH. Formation of strong hydrogen bond between the alginate (–COOH and –OH) and NSC (–COOH and –OH) might have retarded quercetin release at pH 1.2.29 However, samples with maximum NSC content released comparatively more quercetin (∼36%) at pH 1.2 which could be assigned to protonation of primary amino groups (–NH3+) on NSC structure (Fig. 6b).
On the contrary, significant quercetin release was observed at pH 7.4. A sustained release pattern of encapsulated quercetin was evident with progressive increase in swelling leading to disintegration of microformulations at alkaline pH. At this alkaline pH, microparticles of native sodium alginate showed burst quercetin release within 90 min of incubation. Almost entire entrapped quercetin (∼95%) was released due to high electrostatic repulsion force between the ionized carboxylic groups on polymeric chain. But all other polymer microformulations released quercetin following sustained and controlled manner. Considerable swelling and slow sustained release of almost total encapsulated quercetin was observed within 6 h of incubation in pH 7.4 (Fig. 6b).
To deduce the actual mechanism of quercetin release from the microparticles, the parameters ‘n’ of the Ritger–Peppas equation was computed and in vitro release data are presented in Table 3. The correlation coefficient values (R ≥ 0.99) of all the formulations were fitted well to the empirical equation. Furthermore, the release exponent ‘n’ ranges between 0.88–0.89 in case of all the formulations, indicating an anomalous non-Fickian (0.45 < n < 0.89) diffusion mechanism of quercetin release from the NSC/alginate microparticles.
Table 3 Estimated parameters and drug release mechanism of quercetin microparticles
Samples |
Ritger—Peppas |
Mechanism of drug transport |
r2 |
n |
pH 1.2 |
F1(NSC : ALG = 0 : 1) |
0.9808 |
0.4690 |
Non-Fickian |
F2(NSC : ALG = 2 : 1) |
0.9169 |
0.6125 |
Non-Fickian |
F3(NSC : ALG = 1 : 1) |
0.9334 |
0.5419 |
Non-Fickian |
F4(NSC : ALG = 0.5 : 1) |
0.9347 |
0.5175 |
Non-Fickian |
F5(NSC : ALG = 0.25 : 1) |
0.9176 |
0.4646 |
Non-Fickian |
F6(NSC : ALG = 1 : 0) |
0.8961 |
0.7848 |
Non-Fickian |
![[thin space (1/6-em)]](https://www.rsc.org/images/entities/char_2009.gif) |
pH 7.4 |
F1(NSC : ALG = 0 : 1) |
0.8770 |
0.7896 |
Non-Fickian |
F2(NSC : ALG = 2 : 1) |
0.8998 |
0.8028 |
Non-Fickian |
F3(NSC : ALG = 1 : 1) |
0.9109 |
0.7601 |
Non-Fickian |
F4(NSC : ALG = 0.5 : 1) |
0.9212 |
0.7521 |
Non-Fickian |
F5(NSC : ALG = 0.25 : 1) |
0.9489 |
0.7468 |
Non-Fickian |
F6(NSC : ALG = 1 : 0) |
0.9708 |
0.7852 |
Non-Fickian |
pH sensitive release of the drug is a prerequisite for successful oral administration. It is reported that fasting pH of the stomach is about 2.0–6.0 and the presence of food stimulates HCl secretion which in turn alters the pH to 1.2–2.0. On the other hand, intestinal pH ranges between 6.8–7.4.29,48 Therefore, orally administered drug loaded polymeric formulations must go through different pH environments during its passage through gastrointestinal tract and finally the drug is absorbed from the small intestine. However, small intestine transit time also varies from 3–4 h in healthy person.29 In the present study, formulated polymeric microparticles maintained excellent pH sensitive quercetin release, limiting its fast dissolution in acidic stomach pH followed by slow sustained release in alkaline intestinal pH. The formulations with NSC/alginate weight ratio of 1
:
1 and 0.5
:
1 showed the best result in vitro release studies and further used for in vivo experiments.
3.6. Assessment of mucoadhesion study and in vivo pharmacological response
Mucoadhesion is also an important criterion in successful oral drug delivery. The strong interaction of quercetin loaded microparticles with the mucous lining of the intestinal lumen and prolonged attachment with the intestinal wall would provide sustained release and better absorption in the animal body. The microparticles are observed to be firmly attached to the rat intestinal lumen, even after continuous washing with buffer (pH 7.4) for 30–45 min as shown in Fig. 7a. Both NSC and ALG possess mucoadhesive nature9 and adsorption-enhancing properties, which contributed to this prolonged attachment. Chitosan and its derivates (NSC) showed excellent mucoadhesion owing to flexibility of their structure and polar functional groups. The free carboxyl groups of NSC forms strong hydrogen bonding with the hydroxyl groups of mucin and this could be attributed to the better mucoadhesion between the intestine mucous lining and these polymeric materials.28 Again, some of the chitosan monomer residues retained their protonated structure even after reaction with succinic anhydride, offering strong electrostatic interaction with the negatively charged mucous layer of the intestine. This in turn may also enhance the paracellular drug absorption through the tight junctions existing between intestinal epithelial cells. Therefore, obtained results suggested that NSC/alginate microparticles significantly elevated mucoadhesion and could serve as efficient oral quercetin carrier.
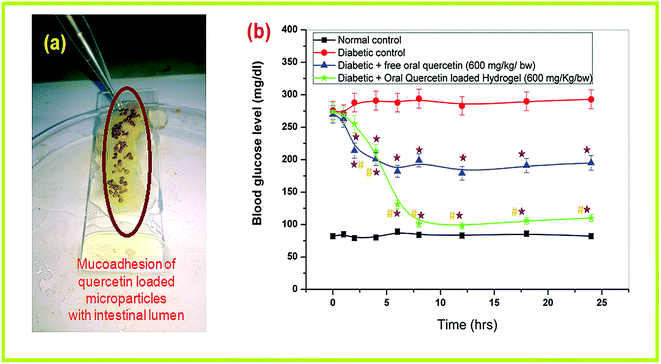 |
| Fig. 7 (a) Mucoadhesion study in excised rat intestinal lumen (b) in vivo pharmacological response of quercetin loaded polymeric micro-formulation in hypoglycemic study. | |
The oral hypoglycemic responses of QUE loaded polymeric microparticles were investigated in diabetic rats in comparison to the free oral QUE at a similar single dose of 600 mg kg−1 body weight. Blood glucose level in different groups of rats was monitored at different time intervals to evaluate the efficacy of orally administered QUE loaded microparticles (Fig. 7b). It is observed that STZ treatment caused a high rise in blood sugar level in comparison to that the control animal group. Both free oral QUE (600 mg kg−1 b.w) and orally administered QUE loaded polymeric microparticles (600 mg kg−1 b.w) treated animal groups showed significant reduction in glycemia in comparison to the diabetic group. However, orally treated QUE loaded polymeric microparticles exhibited highly significant down regulation of blood glucose levels as compared to free oral QUE treated group (Fig. 7b). The QUE loaded polymeric microparticles showed hypoglycemic effects after 2–3 h of oral delivery but the effect was sustained up to 24 h of oral delivery. The in vivo hypoglycemic results are quite consistent with in vitro QUE release. This may be explained by the fact that the microparticles initially retard QUE release in stomach and after 3 h of oral delivery; the microformulations might reach the intestine and upon releasing QUE in sustained manner helped in significant glycemic control in diabetics.
3.7. Toxicity study
No significant changes in body weight, food intake, water intake, hair loss or hair colour difference and behavior were noticed in polymeric microparticle treated rats as compared to control animals. No considerable differences were found between the animal groups regarding fasting blood glucose level, cholesterol, and triglyceride levels as shown in Table 4.
Table 4 Estimation of blood glucose, cholesterol and triglyceride in toxicity study
Animal group |
Fasting blood glucose level (mg dL−1) |
Cholesterol (mg dL−1) |
Triglyceride (mg dL−1) |
NC (normal control) |
85.4 ± 0.6 |
77.4 ± 0.835 |
61.38 ± 2.09 |
NTBM (treated with blank microparticles) |
87.3 ± 0.8 |
81.11 ± 0.72 |
58.3 ± 1.74 |
NTQM (treated with quercetin loaded microparticles) |
81.2 ± 0.7 |
75.55 ± 1.12 |
60.21 ± 2.06 |
3.7.1. Analysis of liver marker enzymes. ALT, AST and ALP are liver specific enzymes which are responsible for proper functioning of the liver. Due to toxicity hepatocellular damages may lead to excessive leakage of these enzymes into the blood stream. ALP is considered as biliary function and cholestasis marker.49 On hepatic tissue damages the activity of this enzyme significantly increases. However, there is no significant change in serum ALP activities of animal groups (Fig. 8a), indicating no hepatic tissue damage. Furthermore, elevated AST and ALT activities in the serum are the indicator of hepatocellular damages.50 It is observed from Fig. 8b that ALT activity of control group, blank microparticles treated group and QUE loaded microparticles treated groups have no significant differences. With respect to serum AST activity, blank microparticles treated group and QUE loaded microparticles treated groups showed significant difference in comparison to control; however the values were within the normal range suggesting no considerable damage to the liver after microparticle treatment.
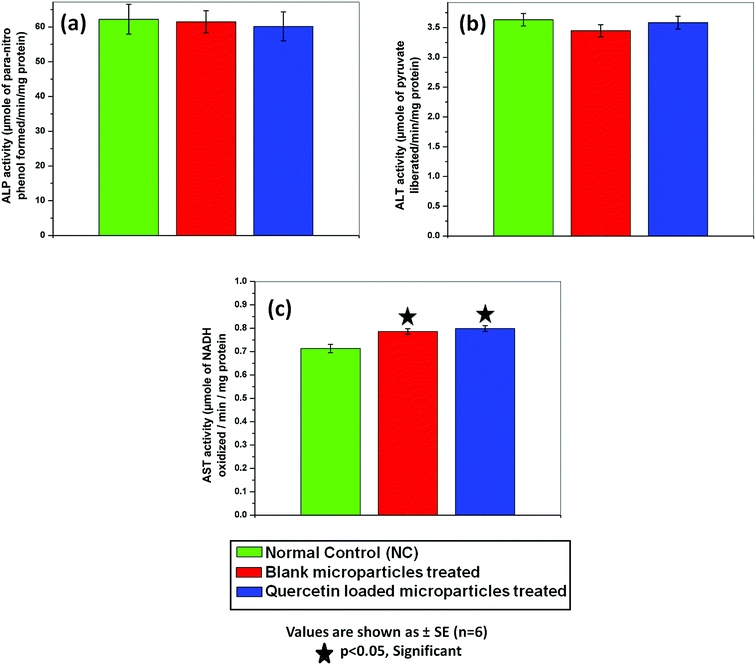 |
| Fig. 8 Analysis of liver marker enzymes (a) ALP, (b) ALT, (c) AST in toxicity study. | |
3.7.2. Antioxidant status evaluation. Antioxidant defence is an important aspect of the normal health maintenance. An imbalance between reactive oxygen species (ROS) and poor anti-oxidant activity may lead to develop several health complications.49 Therefore, antioxidant status after oral polymeric microparticle treatment was measured by SOD, catalase enzymatic markers as presented in Table 5. The results showed no significant alteration in SOD, catalase activities in blank microparticles and QUE loaded microparticles treated groups compared to the control one, suggesting no remarkable oxidative stress after oral delivery of NSC/alginate micro-formulations.
Table 5 Effects of polymeric microparticles on antioxidant status of treated rats
Animal groups |
Serum SOD (unit per mg of protein content) |
Serum catalase activity (unit per mg of protein content) |
NC (normal control) |
0.4173 ± 0.003 |
0.2458 ± 0.004 |
NTBM (treated with blank microparticles) |
0.4158 ± 0.005 |
0.2198 ± 0.002 |
NTQM (treated with quercetin loaded microparticles) |
0.4345 ± 0.011 |
0.2340 ± 0.003 |
3.7.3. Pathohistological and haematological parameters. The pathohistological observation showed no damage to liver, kidney and intestine of treated rats, after H&E (hematoxylin and eosin) staining (Fig. 9a). Overall, the appearance of liver section of blank microparticles and QUE loaded microparticles treated group is almost similar to that of the control one; central vein with radiating hepatic cells were observed, suggesting no apparent hepato-toxicity after treatment. Furthermore, the sections of the kidney, in both treated animal and the control one, show the presence of the renal corpuscle surrounded by Bowman's capsule; kidney tubules are lined by simple cuboidal epithelium and the urinary space (appears as a clear space) is also clearly visible on histological slides. The glomerulus, (a tuft of capillaries), also appears as a large cellular mass. The intestinal sections of the treated animals showed normal architecture, similar to the control tissue. However, a little alteration in the intestinal epithelial cells and outer lining was observed. These findings from histology imply that no toxicity is documented after oral treatment of the polymeric microparticles in animal model.
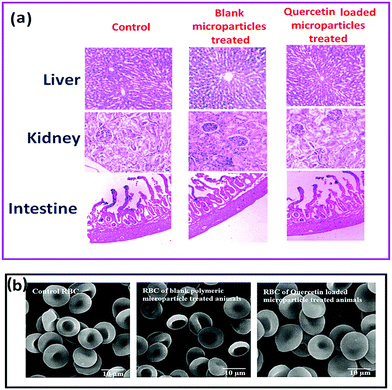 |
| Fig. 9 (a) Internal vital organs of a treated animal and sections of the liver, kidney and intestine (H&E staining, magnification 40×) of the control and treated rats (b) red blood cell under SEM of treated and control animals. | |
Furthermore no morphological alteration of red blood cells (RBC) in NSC/alginate microparticles treated groups were observed in comparison to control animal RBC (Fig. 9b). So, there was no toxic effect of polymeric formulations in rat models.
All animal experiments in this study were approved by animal ethics committee (IAEC) of University of Calcutta (Registration no. 935/c/06/CPCSEA, 30.06.2009) as per guidance of the Committee for the Purpose of Control and Supervision of Experiments on Animals (CPCSEA), Government of India.
4. Conclusions
Water soluble chitosan derivative N-succinyl chitosan was synthesized and characterized by FT-IR and XRD. The reaction has been also explored using quantum mechanical (QM) calculation. Overall, the results suggested successful preparation of pH responsive, porous microparticles for oral delivery of quercetin in diabetics. Indeed, the microformulations were able to produce better controlled release of quercetin following a non-fickian anomalous diffusion mechanism, retarding quercetin release in acidic stomach and facilitating almost complete release in alkaline intestine. The quercetin loaded NSC/alginate microparticles showed excellent quercetin encapsulation efficiency of 94% and produced significant hypoglycemic response following oral delivery in diabetic rats. Moreover, toxicity study with these polymeric particles ensured a new safe and efficient polymeric vehicle for oral quercetin administration.
Acknowledgements
Dr Piyasi Mukhopadhyay greatly appreciates and acknowledges financial support under Dr D. S. Kothari Postdoctoral fellowship [No. F.4-2/2006(BSR)/CH/14-15/0082, Dated: March, 2015] funded by the University Grants Commission (UGC), New Delhi, India. Mr Subhajit Maity acknowledges University Grants Commission (UGC), Government of India for financial assistance (UGC/58/Jr.Fellow(Sc) dated 25.01.2012). The authors are highly thankful to Prof N. D. Kulkarni, Head, Dept. of Chemistry, The M. S. University of Baroda, Vadodara for providing laboratory and instrumental facilities. Authors thank Dr Soumalee Basu, coordinator, Centre for High Performance Computing for Modern Biology, Ballygunge Science College, University of Calcutta, India, for kindly providing the computational facility. Authors wish to thank Mr Pratyush Sengupta, University of Calcutta for conducting SEM imaging in this study.
Notes and references
- S. Wild, G. Roglic, A. Green, R. Sicree and H. King, Diabetes Care, 2004, 27, 1047 CrossRef PubMed
. - A. Ramachandran, R. C. W. Ma and C. Snehalatha, Lancet, 2010, 375, 408 CrossRef
. - A. Saxena and V. N. Kishore, Jpn. J. Compl. Alternative Med., 2004, 10, 369 CrossRef PubMed
. - J. K. Grover, V. Vats, S. S. Rathi and R. J. Dewar, J. Ethnopharmacol., 2001, 76, 233 CrossRef CAS PubMed
. - S. Mohan and L. Nandhakumar, J. Med. Hypotheses Ideas, 2014, 8, 1 CrossRef CAS
. - G. Brahmachari and D. Gorai, Curr. Org. Chem., 2006, 10, 873 CrossRef CAS
. - E. Nicolle, F. Souard, P. Faure and A. Boumendjel, Curr. Med. Chem., 2011, 18, 2661 CrossRef CAS PubMed
. - G. Brahmachari, Nat. Prod. Commun., 2008, 3, 1337 CAS
. - M. Hazra, D. Dasgupta Mandal, T. Mandal, S. Bhuniya and M. Ghosh, Saudi Pharm. J., 2015, 23, 429 CrossRef PubMed
. - P. Mukhopadhyay and A. K. Prajapati, RSC Adv., 2015, 5, 97547 RSC
. - D. V. Ratnam, D. D. Ankola, V. Bhardwaj, D. K. Sahana and M. N. Kumar, J. Controlled Release, 2006, 113, 189 CrossRef CAS PubMed
. - T. Pralhad and K. Rajendrakumar, J. Pharm. Biomed. Anal., 2004, 34, 333 CrossRef CAS PubMed
. - H. Pool, D. Quintanar, J. de Dios Figueroa, C. M. Mano, J. E. H. Bechara, L. A. Godínez and S. Mendoza, J. Nanomater., 2012, 86, 1 CrossRef
. - P. Mukhopadhyay, R. Mishra, D. Rana and P. P. Kundu, Prog. Polym. Sci., 2012, 37, 1457 CrossRef CAS
. - H. Gong, M. Liu, J. Chen, F. Han, C. Gao and B. Zhang, Carbohydr. Polym., 2012, 88, 1015 CrossRef CAS
. - P. Mukhopadhyay, K. Sarkar, S. Bhattacharya, R. Mishra and P. P. Kundu, RSC Adv., 2014, 4, 43890 RSC
. - J. Shi, N. M. Alves and J. F. Mano, J. Biomed. Mater. Res., Part B, 2008, 84, 595 CrossRef PubMed
. - P. Mukhopadhyay, S. Bhattacharya, A. Nandy, A. Bhattacharyya, R. Mishra and P. P. Kundu, J. Toxicol. Res., 2015, 4, 281 RSC
. - R. A. A. Muzzarelli, J. Boudrant, D. Meyer, N. Manno, M. DeMarchis and M. G. Paoletti, Carbohydr. Polym., 2012, 87, 995 CrossRef CAS
. - K. Nagpal, S. K. Singh and D. N. Mishra, Chem. Pharm. Bull., 2010, 58, 1423 CrossRef CAS PubMed
. - Y. Murata, D. Jinno, D. Liu, T. Isobe, K. Kofuji and S. Kawashima, Molecules, 2007, 12, 2559 CrossRef CAS PubMed
. - C. Caddeo, A. Nácher, O. Díez-Sales, M. Merino-Sanjuán, A. M. Fadda and M. Manconi, J. Microencapsulation, 2014, 31, 694 CrossRef CAS PubMed
. - X. Jiang, H. Dai, K. W. Leong, S. H. Goh, H. Q. Mao and Y. Y. Yang, J. Gene Med., 2006, 8, 477 CrossRef CAS PubMed
. - M. Thanou, B. I. Florea, M. Geldof, H. E. Junginger and G. Borchard, Biomaterials, 2002, 23, 153 CrossRef CAS PubMed
. - B. Lu, C. F. Wang, D. Q. Wu, C. Li, X. Z. Zhang and R. X. Zhuo, J. Controlled Release, 2009, 137, 54 CrossRef CAS PubMed
. - S. Mao, U. Bakowsky, A. Jintapattanakit and T. Kissel, J. Pharm. Sci., 2006, 95, 1035 CrossRef CAS PubMed
. - P. Mukhopadhyay, K. Sarkar, M. Chakraborty, S. Bhattacharya, R. Mishra and P. P. Kundu, Mater. Sci. Eng., C, 2013, 33, 376 CrossRef CAS PubMed
. - P. Mukhopadhyay, K. Sarkar, S. Bhattacharya, A. Bhattacharyya, R. Mishra and P. P. Kundu, Carbohydr. Polym., 2014, 112, 627 CrossRef CAS PubMed
. - P. Mukhopadhyay, K. Sarkar, S. Soam and P. P. Kundu, J. Appl. Polym. Sci., 2013, 129, 835 CrossRef CAS
. - R. Pal, M. Roy and A. S. Chakraborti, Adv. Sci. Lett., 2012, 10, 127 CrossRef CAS
. - P. L. Ritger and N. A. Peppas, J. Controlled Release, 1987, 5, 37 CrossRef CAS
. - P. Mukhopadhyay and P. P. Kundu, RSC Adv., 2015, 5, 93995 RSC
. - H. Malekinejad, A. Rezabakhsh, F. Rahmani and R. Hobbenaghi, Phytomedicine, 2012, 19, 583 CrossRef CAS PubMed
. - S. Reitman and S. Frankel, Am. J. Clin. Pathol., 1957, 28, 56 CrossRef CAS PubMed
. - D. Thambidorai and B. K. Bachawat, J. Neurochem., 1977, 29, 503 CrossRef
. - E. Amador and W. Wacker, Clin. Chem., 1962, 8, 343 CAS
. - O. H. Lowry, N. J. Rosebrough, A. L. Farr and R. J. Randal, J. Biol. Chem., 1951, 193, 265 CAS
. - S. Sakuljaitrong, S. Chomko, C. Talubmook and N. Buddhakala, ARPN Journal of Science and Technology, 2012, 2, 1049 Search PubMed
. - S. Marklund and G. Marklund, Eur. J. Biochem., 1974, 47, 469 CrossRef CAS PubMed
. - H. Aebi, Methods Enzymol., 1984, 105, 121 CAS
. - P. Mukhopadhyay, S. Chakraborty, S. Bhattacharya, R. Mishra and P. P. Kundu, Int. J. Biol. Macromol., 2015, 72, 640 CrossRef CAS PubMed
. - T. H. Wu, F. L. Yen, L. T. Lin, T. R. Tsai, C. C. Lin and T. M. Cham, Int. J. Pharm., 2008, 346, 160 CrossRef CAS PubMed
. - Y. Zhang, Y. Yang, K. Tang, X. Hu and G. Zou, J. Appl. Polym. Sci., 2008, 107, 891 CrossRef CAS
. - J. Xing, D. Zhang and T. Tan, Int. J. Biol. Macromol., 2007, 40, 153–158 CrossRef CAS PubMed
. - Y. N. Dai, P. Li, J. P. Zhang, A. Q. Wang and Q. Wei, Biopharm. Drug Dispos., 2008, 29, 173 CrossRef CAS PubMed
. - Y. H. Lin, H. F. Liang, C. K. Chung, M. C. Chen and H. W. Sung, Biomaterials, 2005, 26, 2105 CrossRef CAS PubMed
. - R. Gong, C. Li, S. Zhu, Y. Zhang, Y. Du and J. Jiang, Carbohydr. Polym., 2011, 85, 869 CrossRef CAS
. - S. C. Chen, Y. C. Wu, F. L. Mi, Y. H. Lin, L. C. Yu and H. W. Sung, J. Controlled Release, 2004, 96, 285 CrossRef CAS PubMed
. - S. Das, P. Roy, R. Pal, R. G. Auddy, A. S. Chakraborti and A. Mukherjee, PLoS One, 2014, 9, 101818 Search PubMed
. - R. Sil, D. Ray and A. S. Chakraborti, Mol. Cell. Biochem., 2015, 409, 177 CrossRef CAS PubMed
.
Footnote |
† Electronic supplementary information (ESI) available. See DOI: 10.1039/c6ra12491g |
|
This journal is © The Royal Society of Chemistry 2016 |