DOI:
10.1039/C6RA12422D
(Paper)
RSC Adv., 2016,
6, 63940-63945
Coordinate-polymerized organic ligand towards an efficient catalyst for selective hydrocarbon oxidation†
Received
13th May 2016
, Accepted 27th June 2016
First published on 29th June 2016
Abstract
Here we show catalyst design based on an isoxazole derivative via a coordinate-polymerized strategy. Three new coordination polymers, [Co(bpp)(mpca)2] (1), [Zn(bpp)(mpca)2] (2), and [Cd(bpp)(mpca)2] (3) [bpp = 1,3-bis(4-pyridyl)propane, mpca = 5-methyl-3-phenylisoxazole-4-carboxylic acid], have been synthesized under hydrothermal conditions. Single crystal X-ray diffraction analysis reveals that, compounds 1–3 exhibit similar wave-like chains, with mpca functioning as pendant phenyl-isoxazole arms. Catalytic experiments show that all three compounds can be used as efficient heterogeneous catalysts for the selective oxidation of cyclooctene.
Introduction
The selective catalytic oxidation of hydrocarbons is a significant synthetic method for important chemical intermediates in the manufacture of high-value fine chemicals, high-tonnage commodities and pharmaceuticals.1–4 However, these reactions still suffer from low conversion and unsatisfactory selectivity.3,5,6 Crystal engineering allows the combination of multiple well defined moieties and the integration of different functional units in one system.7–11 Notably, coordination polymers (CPs) with designable structural features provide a platform for catalyst design at molecular and atomic levels.12–15 From the viewpoint of catalyst design,16 an isoxazole derivative (5-methyl-3-phenylisoxazole-4-carboxylic acid, mpca; shown in Scheme 1a) caught our attention. The isoxazole group has a weak N–O bond that can be easily cleaved to form an active diradical (Scheme S1†),17–20 which is favorable for the catalytic process. However, in organic reaction systems, mpca (as catalyst) would suffer from stability and isolation difficulties. While, the coordinate-polymerized design could offer the desired advantages, such as additional thermal stability, and change from homogeneous to heterogeneous catalysis.21–23 With regard to the above aspects, the mpca as organic ligand was introduced into the hybrid system with the purpose of coordinate-polymerization. On the other hand, to extend the structure, another flexible ligand 1,3-bis(4-pyridyl)propane (bpp) with no catalytic activity was employed (shown in Scheme 1b).24–27 In this work, three new coordination polymers, [Co(bpp)(mpca)2] (1), [Zn(bpp)(mpca)2] (2), [Cd(bpp)(mpca)2] (3) have been successfully synthesized under hydrothermal conditions. Compounds 1–3 exhibit similar one-dimensional (1D) structures with mpca functioning as pendant phenyl-isoxazole arms. Catalytic experiments show that all of the three title compounds exhibit excellent catalytic activity for selective oxidation of cyclooctene with air as oxidant directly. Compared with homogeneous catalyst of mpca with low conversion (19.98%), compounds 1–3 can be used as efficient heterogeneous catalysts, which exhibits high conversion based on cyclooctene owing to the enhanced catalytic activity (45.77, 37.78 and 39.08% for 1, 2, and 3, respectively).
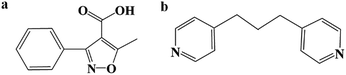 |
| Scheme 1 Representation of ligands mpca (a) and bpp (b). | |
Experimental section
Materials and measurements
All reagents and solvents were used as received from commercial sources without further purification. Elemental analyses were performed on an EA-1110 elemental analyzer. The FT-IR spectra were recorded on a HYPERION spectrometer with a pressed KBr pellet in the 4000–400 cm−1 region. The X-ray power diffraction (XRPD) data were collected by an X'Pert-ProMPD (Holand) D/max-γA X-ray diffractometer in a flat plate geometry with Cu Kα radiation (λ = 1.5406 Å). Thermogravimetric (TG) analysis was carried out with a Pyris-Diamond TG/DTA instrument in flowing N2 with a heating rate of 10 °C min−1. All samples of catalytic experiments were determined by a gas chromatography (Agilent GC 7890A) and a gas chromatography-mass spectroscopy (Trace ISQ).
Syntheses
The title compounds 1–3 were synthesized under similar hydrothermal conditions from a mixture of mpca, bpp, and M(Ac)2 (1: M = Co; 2: M = Zn; 3: M = Cd) in a molar ratio of 1
:
1
:
2. The suspension was sealed into a 15 mL Teflon-lined autoclave and kept under autogenous pressure at 120 °C for 3 days. After cooling to room temperature slowly, purple block crystals of 1, colorless block crystals of 2 and 3 suitable for X-ray diffraction analysis were isolated. Yield: 35% for 1, 20% for 2, and 40% for 3 with respect to M(Ac)2. Elemental analyses calc. for 1: C, 63.49; H, 4.53; N, 8.46. Found: C, 63.52; H, 4.56; N, 8.42. For 2: C, 62.8; H, 4.49; N, 10.18. Found: C, 62.81; H, 4.52; N, 10.14. For 3: C, 57.29; H, 4.37; N, 9.28. Found: C, 57.33; H, 4.39; N, 9.25.
Selective oxidation experiments
The selective oxidation of cyclooctene was carried out in the absence of solvents. The cyclooctene (5 mL, 95%) and different catalysts (50 mg) were added to 20 mL round bottom flask with water condenser and continuous magnetic stirring at 80 °C using tert-butyl hydroperoxide (TBHP, 200 μL, 70%) as initiator and air as oxidant. After filtering off the catalyst, the oxidation products were analyzed by gas chromatography (GC) and gas chromatography-mass spectroscopy (GC-MS).
Recycle test
For the recycle test of the compounds, the catalyst (compound 1/2/3, 50 mg) was separated from the reaction solution after the first catalytic cycle, washed several times with distilled water to remove the physisorbed molecules, and dried. Then the catalyst was reused in a new catalytic experiment. The repeated catalysis experiments were carried out five times using the recycled catalyst. In contrast, the recycle test of the physical mixture materials of compounds was not performed. Because some components in the physical mixture materials system dissolve in the cyclooctene, which means it can't be reused as heterogeneous catalysts in another catalytic system.
X-ray crystallographic study
Crystal data for compounds 1–3 were collected by a Bruker APEX-II CCD diffractometer with Mo Kα radiation (λ = 0.71073 Å) at 296 K. The structures were solved by the direct methods and refined on F2 by full-matrix least-squares using the SHELXTL crystallographic software package.28,29 For all the compounds, all non-hydrogen atoms were refined anisotropically, and all the hydrogen atoms attached to carbon atoms were generated geometrically and refined isotropically with fixed thermal factors. A summary of the crystallographic data and structure refinement details is given in Table 1. Selected bond distances (Å) and angles (°) are listed in Table S1.† Hydrogen-bonding parameters are summarized in Table S2.† CCDC numbers are 1478067–1478069† for 1–3, respectively.
Table 1 Crystal data and structure refinements for compounds 1–3
|
1 |
2 |
3 |
R1 = ∑||F0| − |Fc||/∑|F0|. wR2 = ∑[w(F02 − Fc2)2]/∑[w(F02)2]1/2. |
Formula |
C35H30CoN4O6 |
C35H30ZnN4O6 |
C35H32CdN4O7 |
Fw |
661.56 |
668.00 |
733.05 |
Cryst. syst. |
Monoclinic |
Monoclinic |
Triclinic |
Space group |
P21/c |
P21/c |
P![[1 with combining macron]](https://www.rsc.org/images/entities/char_0031_0304.gif) |
a (Å) |
12.4940(17) |
12.2829(7) |
11.5100(11) |
b (Å) |
15.187(2) |
14.9256(8) |
12.0412(12) |
c (Å) |
20.2212(19) |
20.7045(9) |
13.0266(12) |
α (°) |
90.00 |
90.00 |
97.693(2) |
β (°) |
126.581(6) |
125.874(2) |
107.578(2) |
γ (°) |
90.00 |
90.00 |
100.029(2) |
V (Å3) |
3081.1(7) |
3075.7(3) |
1661.0(3) |
Z |
4 |
4 |
2 |
Dc (g cm−3) |
1.426 |
1.443 |
1.466 |
μ (mm−1) |
0.611 |
0.852 |
0.711 |
F(000) |
1372.0 |
1384.0 |
748.0 |
Collcd reflns |
15 813 |
16 101 |
8842 |
Unique reflns |
5433 |
5410 |
5754 |
Parameters |
415 |
415 |
424 |
Rint |
0.0433 |
0.0372 |
0.0227 |
GOF on F2 |
1.009 |
1.031 |
1.013 |
R1a [I > 2σ(I)] |
0.0421 |
0.0486 |
0.0260 |
wR2b (all data) |
0.1228 |
0.1298 |
0.0666 |
TG and PXRD analyses
Thermogravimetric (TG) analyses of 1–3 were performed on polycrystalline samples under a nitrogen atmosphere to check their thermal stability (Fig. S12†). For 1, the weight loses 60.74% for the decomposition of mpca in the temperature range of 215 to 335 °C (calcd 60.82%). In the temperature range of 335 to 800 °C, the 1D structure of 1 decomposes completely. The remaining weight of 8.92% is in accord with the mass of Co residue (8.92%). For 2, in the temperature range of 220 to 315 °C, the weight loss of 60.48% corresponds to the loss of mpca ligands (60.24%). The bpp ligands do not decompose completely at 800 °C. For 3, the structure began to decompose at about 180 °C, and ended at about 500 °C. For mpca, it decomposes completely in the range of 165 to 272 °C.
The PXRD patterns of compounds 1–3 were recorded at room temperature. As shown in Fig. S13,† all of the major diffraction peaks of experimental patterns match well with their theoretical patterns, indicating the phase purities of these compounds.
Results and discussion
Structure of compounds 1–3
Structure analysis reveals that compound 1 crystallizes in a monoclinic system with space group P21/c. The asymmetric unit consists of one Co2+ ion, one bpp and two mpca molecules, as shown in Fig. 1a. In 1, the Co atom is six-coordinated by two N atoms from two bpp ligands and four O atoms from two mpca ligands, forming a [CoN2O4] octahedron (Fig. S1a†). In 1, all adjacent [CoN2O4] octahedrons are linked by bpp ligands to form a wave-like chain, with mpca functioning as pendant phenyl-isoxazole arms (Fig. 1b). Furthermore, the adjacent chains are parallel arranged via the hydrogen bonds between the pendant arms of mpca, forming a two dimensional (2D) supramolecular layer (Fig. 1c). Note that two adjacent supramolecular layers could join together via hydrogen-bonding interactions to form a double layer structure (Fig. S2†).
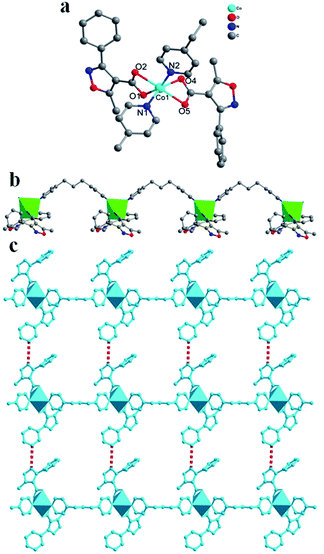 |
| Fig. 1 (a) The coordination environment view of compound 1. All hydrogen atoms are omitted for clarity. (b) The 1D wave-like chain in 1. (c) The 2D supramolecular layer. Hydrogen bonds were replaced by red dotted line. | |
As for compounds 2 and 3 (Fig. S3 and S4†), wave-like chain structures, similar to that of 1, are also observed (Table 2). As is known, different metal ions have preferential coordination geometry due to their electronic configurations. In detail, each Zn2+ ion in 2 is four-coordinated by two O atoms from two mpca ligands and two N atoms from two bpp ligands in a [ZnO2N2] tetrahedron (Fig. S1b†). In 3, each Cd2+ ion is seven-coordinated by four chelating O atoms from two mpca ligands, two N atoms from two bpp ligands and one O atom from a coordinated water molecule, thus, the coordination polyhedron around Cd2+ ion is a [CdN2O5] decahedron (Fig. S1c†). Bond lengths around metal centres are normal (Table S1†), except weak Zn–O interactions that are much longer according to the literature.30–32
Table 2 A summary of structural information of compounds 1–3
Compounds |
1 |
2 |
3 |
Different TM ions |
 |
 |
 |
Coordination modes of TM |
 |
 |
 |
Coordination modes of mpca |
 |
 |
 |
Similar 2D supramolecular structure |
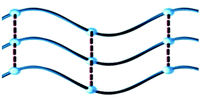 |
From the structural descriptions above, we can see that different choice of the transition metals apparently induce various coordination and conformation modes of ligands. For bpp, different conformations with similar bridging mode have been observed in 1–3 (Fig. S5†). For mpca, when Co2+ was introduced to the system in 1, the mpca adopts the simple chelate mode which acts as terminal ligand by its two carboxyl oxygens. When Zn2+ was used in 2, mpca acted as a monodentate ligand by one carboxyl oxygen, hanging on both sides of the chain. In 3, mpca utilize a chelate mode, similar to that in 1. Arrangements of mpca hanging up and down the chain are also different. In 1 and 2, the mpca ligands arrange in a head-to-head mode, while in 3, a shoulder-by-shoulder mode is observed (Fig. S6†). Despite the slight difference, the main structural feature of mpca relies on the terminal role that functioning as pendant phenyl-isoxazole arms within all of the three title compounds.
In present system, the coordinate-polymerized design also offer some advantages. Compared with mpca ligand, which suffers from a low thermal stability (decomposition at 165 °C), the mpca-based hybrid material of 1 exhibits a higher stability (decomposition at 215 °C), as illustrated in Fig. 2. Additional stabilization arises from the geometrical constraints of coordinate-polymerized structure, which contains a cascade of coordinate and hydrogen bonds. Similar phenomenon has also been observed for compounds 2 and 3. On the other hand, the solution processes of mpca and 1 soaked in cyclooctene at different time periods are shown in Fig. 3. The mpca is entirely dissolved within 10 min, while compound 1 is indissoluble within at least 10 days. The results implied that, when coordinate-polymerized, the hybrid material of 1 could be used as heterogeneous catalyst.
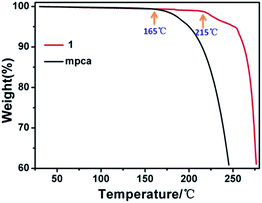 |
| Fig. 2 TG spectra of 1 and mpca, showing the decomposition temperature. | |
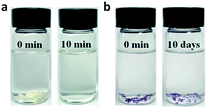 |
| Fig. 3 (a) Photographs of ligand mpca soaked in cyclooctene at different time periods. It shows the total dissolution of mpca in cyclooctene within 10 min. (b) Photographs of compound 1 immersed in cyclooctene for 10 days. | |
Selective oxidation of cyclooctene
The catalytic activities of mpca (31 mg), bpp (15 mg), metal ion (Co2+, 19 mg Co(Ac)2) and compounds 1–3 were investigated over the selective oxidation of cyclooctene in the molar ratio of 2
:
1
:
1
:
1 with absence of solvents, using TBHP as initiator and air as oxidant at 80 °C (Fig. S7†). Fig. 4a shows the relationship between the conversion of cyclooctene and reaction time with mpca, bpp and Co2+ ion as catalysts, respectively. Detailed conversion of cyclooctene and selectivity of oxidation products using mpca, bpp and Co2+ ion as catalysts are shown in Table S3.† As is shown, the catalytic activities are obviously different. For mpca and Co2+ ion, the conversion of cyclooctene reached 19.98 and 17.27%, respectively. While for bpp, it was just about 2.20%. Selectivity of epoxycyclooctane with mpca, bpp and Co2+ ions are shown in Fig. 4b. The selectivity was up to 86.26, 94.96 and 94.18%, respectively. The results indicate the comparative catalytic activities of mpca and Co2+ ions, while bpp exhibits no obvious catalytic activity.
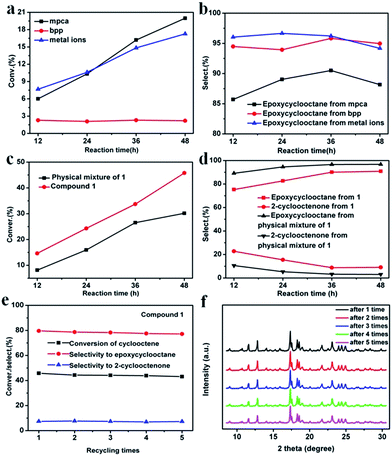 |
| Fig. 4 (a) The relationship between the conversion and reaction time with mpca, bpp and metal ion (Co2+) as catalysts. (b) The relationship between the selectivity and reaction time with mpca, bpp and metal ion (Co2+) as catalysts. (c) The relationship between the conversion and reaction time with compound 1 and physical mixture materials of 1 as catalysts. (d) The relationship between the selectivity and reaction time with compound 1 and physical mixture materials of 1 as catalysts. (e) The relationship between the conversion/selectivity and recycling times with compound 1 as catalyst. (f) The XRPD patterns of compound 1: after 1, 2, 3, 4 and 5 times recycling. | |
In the following experiments, the catalytic activities of compound 1 and physical mixture materials of 1 (mixture of bpp, mpca and Co(Ac)2) were performed. Fig. 4c shows conversion of cyclooctene achieved by compound 1 and physical mixture materials of 1. Detailed data of the catalytic experiments for 1 and physical mixture materials of 1 is shown in Table S4.† It can be seen that the conversion increased with the increasing reaction time. After 48 h, the conversion was up to 45.77% with catalyst 1, while for physical mixture materials of 1, it was 30.15%. Fig. 4d exhibits the selectivity of different oxidation products achieved by compound 1 and physical mixture materials of 1. We can see that, the selectivity of epoxycyclooctane with catalyst 1 increased from 75.28 to 90.85% after 48 h, while the selectivity of 2-cyclooctenone decreased from 22.76 to 9.08%. The total selectivities of epoxycyclooctane and 2-cyclooctenone are less than 100%, which may owe to the further oxidation of some C8 products to other carbon oxides. With catalyst of the physical mixture materials, the selectivity of epoxycyclooctane was up to 96.72% after 48 h, which is higher than that of compound 1. However, the selectivity of 2-cyclooctenone was about 3.05%, which is lower than that of compound 1. These results demonstrate that, after coordinate-polymerized, the hybrid structure of compound 1 as catalyst exhibits higher catalytic activities than that of the physical mixture materials of 1, and the catalytic activity can be remarkably enhanced.
In order to study the stability of compound 1 as catalyst in the catalytic system, the repeated experiments were designed and performed. The catalyst (compound 1) was separated from the reaction solution after the first catalytic cycle, washed several times with distilled water to remove the physisorbed molecules, and dried. Then the catalyst was reused in a new catalytic experiment. The repeated catalysis experiments were carried out five times using the recycled catalyst. The relationship between the conversion of cyclooctene/selectivity of different products and recycling times is shown in Fig. 4e. The catalytic cycle results show that the conversion of the cyclooctene decreases a little with increasing the cyclic number of catalytic cycles. And the selectivity of epoxycyclooctane and 2-cyclooctenone is similar to those of the first catalytic run. Fig. 4f shows the XRPD patterns of compound 1 as catalyst after each recycling time. And the position of peaks is almost no change during 5 recycling times. The results confirm compound 1 still retains its catalytic activities and is stable enough after five catalytic cycles.
Next, the catalytic activities of 2 and 3 were also investigated for oxidation of cyclooctene under the similar conditions. The high conversion of cyclooctene achieved with catalysts 2 (37.78%) and 3 (39.08%) demonstrate their catalytic activities (Fig. 5a). As is shown, the conversion of cyclooctene achieved by 2 is a little higher than that of 3 before 24 h, after that, it is lower than that of 3. For 2, the conversion increased gradually before 36 h and then grew quickly. For 3, the conversion increased quickly from 24 to 36 h, and then grew gradually. The selectivity of epoxycyclooctane achieved by 2 and 3 is shown in Fig. 5b. Note that the selectivity achieved by 3 is always higher than that of 2. The detailed conversion/selectivity for 2 and 3 are shown in Table S5 and S6.† As the control experiments, the physical mixtures of each component for compounds 2 and 3 were used as catalysts. As is shown, the catalytic activities of 2 and 3 are much higher than that of corresponding physical mixtures.
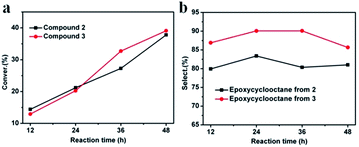 |
| Fig. 5 (a) The relationship between the conversion of cyclooctene and reaction time with catalyst 2 and 3 respectively. (b) The relationship between the selectivity of epoxycyclooctane and reaction time with catalyst 2 and 3, respectively. | |
To demonstrate that compounds 2 and 3 are stable enough for catalytic cycles, a series of catalytic experiments were performed, and detailed results are shown in Fig. S8.† It's clearly that, after 5 times of catalytic experiments, the conversion and selectivity are almost the same. From the XRPD patterns, we can see that the position of peaks is almost no change during 5 recycling times. These results demonstrate that 2 and 3 as catalysts are stable enough for catalytic cycles. Further blank reactions (i.e., without catalysts) do not result in any epoxide and/or other products. Moreover, the filtrate tests (additional 48 h reaction after removing the catalysts from the reaction system) show no catalytic activities (the conversion was 0), indicating that the catalytic activities originated from the addition of compounds 1–3 and is not caused by molecular species in solution. All the catalytic experiments above demonstrate that compounds 1–3 indeed act as heterogeneous catalysts in the present catalytic systems.
To confirm the air activation by the catalyst 1–3, the spin-trapping electron spin resonance (ESR) characterization was carried out. The radicals of superoxide radicals (˙O2−) are captured in methanol solution. In Fig. 6, the signal of six typical characteristic peaks of DMPO–˙O2− adducts are clearly exhibited. For 2 and 3, similar characteristic peaks of ˙O2− were also observed (Fig. S9 and S10†).
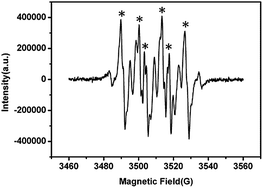 |
| Fig. 6 The ESR signals of the DMPO–˙O2− adducts (compound 1 in methanol solution). DMPO (dimethyl pyridine N-oxide) works as the free radical trapping agent. | |
Based on the ESR analysis and above catalytic results, it can be inferred that the possible mechanism for the oxidation reaction can be described as follows. Firstly, molecular chemisorption of O2 occurred on the surface of 1-dimensional coordination polymers, where O2 was formed active oxygen species (˙O2−) with the action of initiator and catalyst. Then, the active oxygen species would be quickly consumed by the cyclooctene to form a primary epoxide epoxycyclooctane, and then epoxycyclooctane was converted into 1,2-cyclooctanediol, but 1,2-cyclooctanediol was unstable and further converted into 2-cyclooctenone in the sequential oxidation process. The repetition of such reactions constituted the catalytic process, as illustrated in Scheme 2.
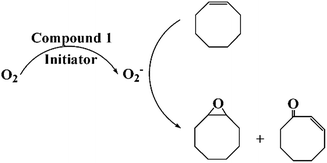 |
| Scheme 2 Possible selective oxidation mechanism based on compound 1. | |
Conclusion
We demonstrated the catalyst design based on an isoxazole derivative via a coordinate-polymerized strategy. Three new coordination polymers have been synthesized under hydrothermal conditions. Compounds 1–3 exhibit similar wave-like chains, with isoxazole-based ligand mpca functioning as pendant arms. Catalytic experiments show that all of the three title compounds can be used as efficient heterogeneous catalysts for selective oxidation of cyclooctene. Compared with homogeneous catalyst of mpca with low conversion, heterogeneous catalysts 1–3 exhibit high conversion owing to the enhanced catalytic activity. The results presented here may open a promising route for the design and fabrication of more CPs with specific catalytic properties.
Acknowledgements
This work is supported by Collaborative Innovation Center of Suzhou Nano Science and Technology, the National Basic Research Program of China (973 Program) (2012CB825803, 2013CB932702), the National Natural Science Foundation of China (51422207, 51132006, 51572179, 21471106, 21501126), the Specialized Research Fund for the Doctoral Program of Higher Education (20123201110018), the Natural Science Foundation of Jiangsu Province of China (BK20140310), China Postdoctoral Science Foundation (2014M560445, 2015T80581) and a project funded by the Priority Academic Program Development of Jiangsu Higher Education Institutions (PAPD).
Notes and references
- S. Caron, R. W. Dugger, S. G. Ruggeri, J. A. Ragan and D. H. B. Ripin, Chem. Rev., 2006, 106, 2943 CrossRef CAS PubMed.
- A. K. Suresh, M. M. Sharma and T. Sridhar, Ind. Eng. Chem. Res., 2000, 39, 3958 CrossRef CAS.
- M. D. Hughes, Y. J. Xu, P. Jenkins, P. McMorn, P. Landon, D. I. Enache, A. F. Carley, G. A. Attard, G. J. Hutchings, F. King, E. H. Stitt, P. Johnston, K. Griffin and C. J. Kiely, Nature, 2005, 437, 1132 CrossRef CAS PubMed.
- F. E. Kühn, E. Herdtweck, J. J. Haider, W. A. Herrmann, I. S. Goncalves, A. D. Lopes and C. C. Romão, J. Organomet. Chem., 1999, 583, 3 CrossRef.
- O. P. H. Vaughan, G. Kyriakou, N. Macleod, M. Tikhov and R. M. Lambert, J. Catal., 2005, 236, 401 CrossRef CAS.
- F. E. Kühn, E. Herdtweck, J. J. Haider, W. A. Herrmann, I. S. Goncalves, A. D. Lopes and C. C. Romão, J. Organomet. Chem., 1999, 583, 3 CrossRef.
- J. P. Zhang, Y. B. Zhang, J. B. Lin and X. M. Chen, Chem. Rev., 2012, 112, 1001 CrossRef CAS PubMed.
- R. Breslow, Science, 1982, 218, 532 CAS.
- A. Stein, B. Melde and R. Schroden, Adv. Mater., 2000, 12, 1403 CrossRef CAS.
- Z. H. Peng, Angew. Chem., Int. Ed., 2004, 43, 930 CrossRef CAS PubMed.
- Y. F. Song, D. L. Long and L. Cronin, Angew. Chem., Int. Ed., 2007, 46, 3900 CrossRef CAS PubMed.
- N. Mizuno, S. Hikichi, K. Yamaguchi, S. Uchida, Y. Nakagawa, K. Uehara and K. Kamata, Catal. Today, 2006, 117, 32 CrossRef CAS.
- D. Hagrman, P. J. Hagrman and J. Zubieta, Angew. Chem., Int. Ed., 1999, 38, 3165 CrossRef CAS.
- C. Streb, C. Ritchie, D.-L. Long, P. Kögerler and L. Cronin, Angew. Chem., Int. Ed., 2007, 46, 7579 CrossRef CAS PubMed.
- C. Inman, J. M. Knaust and S. W. Keller, Chem. Commun., 2002, 156 RSC.
- Y. Ding, J. X. Meng, W. L. Chen and E. B. Wang, CrystEngComm, 2011, 13, 2687 RSC.
- C. M. Nunes, I. Reva and R. Fausto, J. Org. Chem., 2013, 78, 10657 CrossRef CAS PubMed.
- S. A. Lang and Y. I. Lin, Comprehensive Heterocyclic Chemistry, Oxford, Pergamon, U. K., 1984, vol. 6, Part 4B Search PubMed.
- P. Grünanger and P. Vita-Finzi, Chemistry of Heterocyclic Compounds, Wiley, New York, 1991, vol. 49, Part 1 Search PubMed.
- T. M. V. D. Pinhoe Melo, Curr. Org. Chem., 2005, 9, 925 CrossRef CAS.
- D. G. Sun, F. X. Sun, X. Y. Deng and Z. H. Li, Inorg. Chem., 2015, 54, 8639 CrossRef CAS PubMed.
- D. B. Shi, Y. W. Ren, H. F. Jiang, B. W. Cai and J. X. Lu, Inorg. Chem., 2012, 51, 6498 CrossRef CAS PubMed.
- L. Liu, C. Huang, L. Zhang, R. Ding, X. N. Xue, H. W. Hou and Y. T. Fan, Cryst. Growth Des., 2015, 15, 2712 CAS.
- X. S. Qu, L. Xu, G. G. Gao, F. Y. Li and Y. Y. Yang, Inorg. Chem., 2007, 46, 4775 CrossRef CAS PubMed.
- X. L. Wang, Y. F. Bi, B. K. Chen, H. Y. Lin and G. C. Liu, Inorg. Chem., 2008, 47, 2442 CrossRef CAS PubMed.
- H. H. Zou, Y. P. He, L. C. Gui and F. P. Liang, CrystEngComm, 2011, 13, 3325 RSC.
- R. Sibile, T. Mazet, E. Elkaïm, B. Malaman and M. François, Inorg. Chem., 2013, 52, 608 CrossRef PubMed.
- G. M. Sheldrick, SHELXS-97, Program for Crystal Structure Solution, University of Göttingen, Germany, 1997 Search PubMed.
- G. M. Sheldrick, SHELXL-97, Program for Crystal Structure Refinement, University of Göttingen, Germany, 1997 Search PubMed.
- A. Neels and H. Stoeckli-Evans, Inorg. Chem., 1999, 38, 6164 CrossRef CAS PubMed.
- Y. Z. Tang, X. F. Huang, Y. M. Song, H. Chan and R. G. Xiong, Inorg. Chem., 2006, 45, 4868 CrossRef PubMed.
- P. Chaudhuri, M. Hess, J. Muller, K. Hildenbrand, E. Bill, T. Weyhermuller and K. Wieghardt, J. Am. Chem. Soc., 1999, 121, 9599 CrossRef CAS.
Footnotes |
† Electronic supplementary information (ESI) available: Additional figures, TG curves, XRPD patterns, IR spectra and analysis, detailed crystallographic data and structural refinement parameters, selected bond distances and selected hydrogen bond parameters for compounds 1–3. CCDC 1478067–1478069. For ESI and crystallographic data in CIF or other electronic format see DOI: 10.1039/c6ra12422d |
‡ These authors contributed equally to this work. |
|
This journal is © The Royal Society of Chemistry 2016 |
Click here to see how this site uses Cookies. View our privacy policy here.