DOI:
10.1039/C6RA11815A
(Paper)
RSC Adv., 2016,
6, 71046-71051
High-performance poly(lactide) composites by construction of network-like shish-kebab crystals†
Received
6th May 2016
, Accepted 6th July 2016
First published on 7th July 2016
Abstract
Poly(lactide), PLA, suffers from brittleness and poor heat-resistance. Herein, a novel strategy to in situ fabricate high-performance PLA composites is reported for the first time, i.e., by solid-state uniaxial stretching in the presence of N1,N1′-(ethane-1,2-diyl)bis(N2-phenyloxalamide) (OXA) as needle-like nucleation templates. As a consequence, a tensile strength of >120 MPa, an elongation at break of >25% and a heat-resistant temperature of >150 °C of the PLA composites are simultaneously achieved. The superior performances are ascribed to unique interlocked network-like shish-kebab crystal structures that formed upon solid-state hot-stretching in the presence of OXA. This research may create a new type of sustainable engineered plastic.
1. Introduction
Several favorable features such as biodegradability, biocompatibility and easy processability make renewable poly(lactide), PLA, promising as a substitute for petroleum-based plastics.1–3 However, PLA is still challenging for engineering applications due to a notably low heat distortion temperature (HDT, ∼60 °C), brittleness and relatively low tensile strength (∼50 MPa).4–6
The brittleness of PLA can be overcome by plasticization, blending and copolymerization.7–9 Among these approaches, blending is favored in both academia and industry. Consequently, tough(er) PLA-based materials were obtained via incorporation of ethylene–vinyl acetate copolymer, ethylene–octane copolymer, natural rubber or poly(ether-b-amide), accompanied with a reduction in strength and modulus.10–15 Although the strength and modulus can be enhanced to a certain extent by inorganic fillers, the toughness would deteriorate.16–18 Hence, toughness and strength/modulus are difficult for simultaneous achievement. Moreover, the renewability and biodegradability of PLA would be compromised if blended with traditional polymeric modifiers.
The poor heat resistance of PLA is ascribed to a lack of crystallinity and a low glass transition temperature (∼60 °C). An increase in crystallinity can enhance the heat-resistance temperature, stiffness and even mechanical strength, but not necessarily give a better toughness.19 Balanced superior performances like a combination of high strength, toughness and heat-resistance of PLA were mainly achieved via stereo-complexation technologies either in the PLLA/PDLA asymmetric blends or in the PMMA plasticized PLLA/PDLA symmetric blends where the PMMA content is higher than 40 wt%.20 However, the improvement in strength is limited up to ∼70 MPa, and an extra annealing process is required for high heat-resistant temperature. Therefore, it would be of interest to develop a novel route to high-performance PLA materials for engineering applications.
Solid-state hot-stretching technology presents advantages of high production rates, orientation and enhancements in the properties without a complex processing apparatus. Thus, it has been applied to polymeric materials such as poly(oxymethylene),21 poly(propylene)22 and PLA.23,24 Large-scale shish-kebab structures could be formed upon hot-stretching, resulting in improved properties to semi-crystalline materials such as high toughness and modulus.22 The shish-kebab-like structures of PLA were also initiated by one-dimensional nucleating templates.25–27 Recently, our group revealed, for the first time, that oxalamide compounds can serve as soluble-type nucleating agents that are capable of self-assembly via hydrogen bonding into needle-like superstructures from the PLA melt, and subsequently, accelerate crystallization kinetics.28 However, the combination effect of such nucleating templates and the solid hot-stretching technology on the properties of the PLA materials are still not known yet.
The primary objective of this work is to provide a simple but effective route to in situ construct high-performance PLA composites by using needle-like nucleating templates and solid-state uniaxial stretching technology. The resulting parallel-aligned shish-kebab crystals with interlocked boundaries are correlated to the processing and consequent properties. Therefore, the research may create a new type of sustainable engineered plastic.
2. Experimental section
2.1 Materials
Poly(L-lactide) (PLA, 4032D) was purchased from Nature Works LLC, U.S.A, with a Mn = 135 kDa, PDI = 1.7 and a L-lactide content of 98%. The nucleating agent (N1,N1′-(ethane-1,2-diyl)bis(N2-phenyloxalamide)) (OXA) with a melting temperature of 338 °C and a purity of >98% was synthesized in the laboratory. The chemical structure and morphology of OXA is shown in Fig. 1 and S2,† respectively.
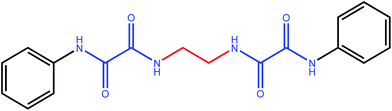 |
| Fig. 1 The structure of N1,N1′-(ethane-1,2-diyl)bis(N2-phenyloxalamide) (OXA). | |
2.2 Sample preparation
The PLA and nucleating agent (OXA) were dried at 60 °C in a vacuum oven for 12 hours before use. Both of the PLA/OXA and neat PLA samples were prepared in a Habo Rheometer (RM-200, HABO Electrical Appliance Manufacturing Company, China) chamber at 180 °C and 50 rpm for 4 min. The concentration of OXA in PLA was 0.5 wt%. The samples were then compressed into sheets at 230 °C and 15 MPa for 2 min using a hot compression molding machine and subsequently cooled down with room-temperature compression plates at a pressure of 5 MPa. The compression molded sheets were pre-stretched at 10 mm min−1 up to 0, 3 and 5 times, respectively. The pre-stretching was carried out at 75 and 85 °C, respectively, using a universal tester equipped with an environment oven (Instron 5967, USA). For comparison, all samples were treated in the same oven for a comparable time span. The sample abbreviation in this work, taking PLA/OXA75-3× for example, means that the PLA/OXA sheets were pre-stretched at 75 °C with a drawn ratio of 3. The pre-heated sheets were used for characterization.
2.3 Characterization
Mechanical properties. Tensile properties of the PLA and PLA/OXA sheets were measured using a universal tester (Instron 5967, USA) according to GBT529-2008 at a crosshead speed of 10 mm min−1. The geometry of the parallel section of the tensile bar is 25 × 4 × 1 mm3. Five specimens of each sample were examined and the averaged values were presented. All the tests were performed at room temperature.
Differential scanning calorimetry (DSC). The crystallization and melting behavior of the PLA and PLA/OXA sheets were investigated by using DSC (204F1, NETZSCH Gerätebau GmbH, Selb, Germany). The samples were heated in nitrogen atmosphere from 30 to 190 °C at a heating rate of 10 °C min−1. The crystallinity of PLA (Xc) is calculated via
29 where Hm and Hcc are the melt and cold crystallization enthalpy of the PLA, respectively, while H0m = 93.6 J g−1 is the melting enthalpy of 100% crystalline PLA.30
Wide angle X-ray diffraction (WAXD). WAXD measurements were carried out by using an X-ray diffractometer (Bruker AXS D8, Germany) equipped with a Ni-filtered Cu Kα radiation source with a wavelength of 1.542 Å. The measurements were operated at 40 kV and 40 mA with scan angles from 5° to 40° and a scan rate of 1° min−1.
Scanning electron microscopy (SEM). Crystal morphology of PLA in the as-prepared and pre-stretched sheets was observed using a scanning electron microscope (S-4800, HITACHI, Japan) at an accelerating voltage of 10 kV. Before observation, the cross sections of the sheets obtained by cryo-fracture were etched with a 1
:
2 water–methanol mixture containing 0.025 mol L−1 NaOH and subsequently coated with a thin gold layer.
Dynamic mechanical analysis (DMA). DMA-Q800 (TA Instruments) was performed to measure the dynamic mechanical properties and thermal behavior of the PLA and PLA/OXA sheets. Specimens with a dimension of 15 × 5.3 × 1.0 mm3 were tested in a tensile-film mode from 30 °C to 150 °C at 3 °C min−1. The amplitude and frequency were set as 20 μm and 1 Hz, respectively. The storage modulus and tan
δ were recorded.
3. Results and discussion
3.1 Network-like shish-kebab crystals formed upon solid-state uniaxial stretching
The PLA and PLA/OXA sheets were uniaxially hot-stretched at 75 and 85 °C, respectively, which are between the Tg and Tcc of the PLA. The cryo-fractured surfaces of both stretched and un-stretched samples were analyzed using SEM after etching the top amorphous layer, as shown in Fig. 2. The PLA-0× sheet presents a smooth surface and large spherulite with radial lamellas (Fig. 2a). The surface became coarse and ordered after the uniaxial stretching (Fig. 2b). Interestingly, a unique shish-kebab-like morphology was clearly observed in the stretched PLA/OXA sheets (Fig. 2c). Such shish-kebab-like crystals exhibit a great tendency to orientate their shish axis parallel to the stretching direction because the stress field could facilitate the alignment of the fibrillar shishes (OXA)31 and the vertical kebab-like lamellae growth on the shishes surface (inset in Fig. 2c). A similar behavior was observed in nanofiber-filled poly(propylene) and PLA/TMC328 systems.27,32 The parallel alignment of the shish-kebab crystals offer an opportunity for most of the lamellae in one crystallite, readily penetrating into the adjacent crystallites and interlocking with their lamellae. As a consequence, PLA composites filled with the novel network-like shish-kebab crystals were in situ fabricated.
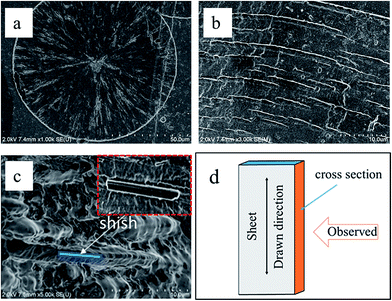 |
| Fig. 2 SEM imagines of etched cross sections of the sheet samples showing the crystal morphology of PLA with and without nucleating agents: (a) PLA-0×, (b) PLA-5×, (c) PLA/OXA-5× with an inset of OXA morphology and (d) the schematic of the direction that the SEM was performed. The hot stretching temperature is 75 °C and the blue lines indicate schematically the fibrillar nucleating agent formed by self-organization in the PLA melt upon cooling. | |
3.2 Mechanical properties of the solid-state stretched PLA/OXA sheets
Mechanical properties of the PLA and PLA/OXA sheets were studied via a uniaxial tensile test, as shown in Fig. 3, and the mechanical data are provided in the ESI (Table S1†). The degree of molecular PLA and OXA orientation, as well as the PLA crystallinity, increases with increasing the stretching ratio, leading to the enhancement in tensile strength and Young's modulus. Consequently, the tensile strength of PLA/OXA was increased from 47 to 120 MPa with a stretching ratio up to five (Fig. 3a). The PLA/OXA sheets possess higher tensile strength, by ∼20 MPa, more than the PLA sheets at the same drawing ratios (3 or 5), although they have comparable tensile strength prior to stretching. Obviously, the nucleating templates and solid-state stretching played a synergetic effect to the formation of shish-kebab-like crystal structures, and thus, a higher performance. On the other hand, the mechanical properties of the PLA and PLA/OXA sheets stretched at 65 or 85 °C were much lower than that of the ones stretched at 75 °C at the same drawing ratio because: (i) 65 °C is close to the glass transition temperature of the PLA and thus lower crystallinity was generated after pre-stretching, which was proved by the DSC results in Fig. S1;† while (ii) 85 °C is already approaching the cold crystallization temperature of PLA (Tcc, see DSC results below), thus too fast crystallization occurs and consequent defects in the crystal structures are formed. In the meantime, the elongation at break of both PLA and PLA/OXA sheets was increased by 5–16 times after pre-stretching regardless of the temperature, indicating a higher toughness as shown in Fig. 3b. It is worth noting that the PLA and PLA/OXA sheets can be drawn more than 5 times at 75 °C, which means an even higher strength and modulus can be achieved.
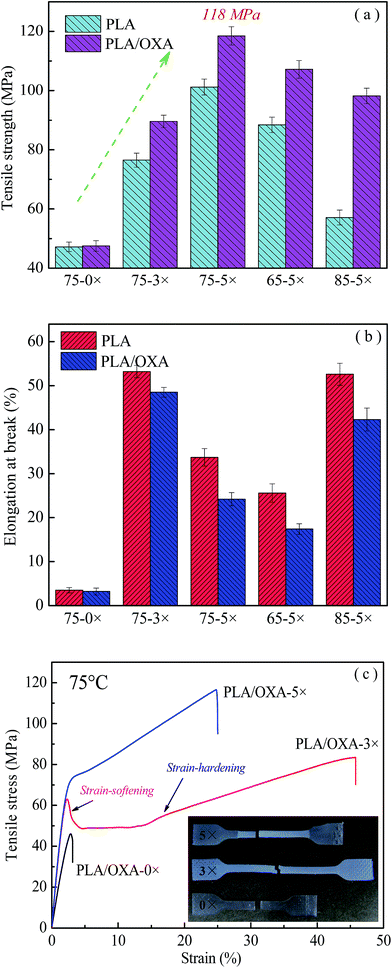 |
| Fig. 3 (a) Tensile strength and (b) elongation at break of the stretched PLA and PLA/OXA sheets as a function of drawing ratios and temperatures, and (c) stress–strain curves of the stretched PLA/OXA sheets with different drawn ratios. The area under the stress–strain curves represents toughness of the materials. A digital image showing the tensile bars after the tensile test, inset of (c), showing the fracture and stress-whitening behavior of the PLA/OXA specimens. All the tensile tests of the stretched sheets were carried out at room temperature. | |
To provide deeper insight in the mechanical behavior, stress–strain curves of the PLA and PLA/OXA sheets were recorded, as shown in Fig. 3c. The un-stretched PLA/OXA sheet failed immediately after yielding. In contrast, the stretched PLA/OXA sheets exhibited weak strain-softening followed by a strain-hardening and larger elongation at break, demonstrating typical tough behaviors. Strain-hardening is usually referred to the stretch of a polymer network that can delocalize stress/strain to prevent material fractures.33 Interestingly, the strain-hardening of the stretched PLA/OXA sheet is even more pronounced at higher stretching ratios, referring to more remarkable network-like shish-kebab crystals and pre-oriented PLA chains. Therefore, the in situ fabricated PLA composites possess both high strength and toughness.
3.3 Mechanism discussion
Superior properties of PLA were achieved by a stereocomplex-induced phase-separation in a recent study.34 Thus, regulating the crystalline morphology is critical in the design of high-performance PLA composites. Fig. 4 gives the phenomenological framework of orientation-induced structural evolution in the PLA/OXA sheets upon solid-state hot-stretching. The oriented PLA macromolecules may form local macromolecular shishes and grown with kebab-like crystals (Part B in Fig. 4), thus improving the mechanical properties. As evidenced by SEM (Fig. 2c) and our previous study,31 many needle-like nucleating templates existed in the samples (Part A in Fig. 4) that facilitated the parallel-aligned shishes and interlocked shish-kebab crystals upon uniaxial stretching (Part C in Fig. 4 and 2c). A network-like structure in the PLA matrix is then constructed by the paralleled shish-kebab crystals with interlocked boundaries. Phase separation between the shish-kebab network and amorphous PLA domains result in stress concentration accompanied with cavitation and/or multi-crazing of the weak(er) phase upon later tensile tests, thus yielding ductile behavior.34 This analysis is in agreement with the stress-whitening behavior of the specimens, see the inset digital image in Fig. 3c. The high orientation of the shish-kebab network and PLA chains is then proposed to be responsible for the improved tensile strength and toughness.
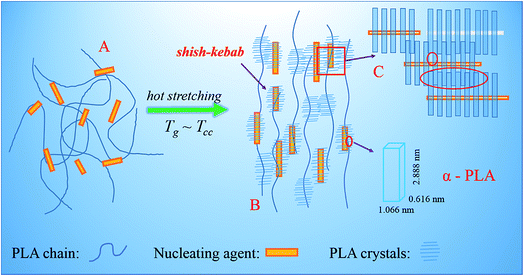 |
| Fig. 4 Schematic of the crystalline morphology of the PLA induced by the nucleating agent and uniaxial solid-state hot-stretching. The symbols of PLA chains, nucleating agent and PLA crystals are denoted in the figure. | |
3.4 Crystallization and crystal structure analysis by DSC and WAXD
Fig. 5a shows the first heating DSC curves of the PLA and PLA/OXA sheets that were pre-stretched at 75 °C with different ratios. The cold crystallization temperature (Tcc), melting temperature (Tm) and crystallinity (Xc) are indicated in Fig. 5a. Un-stretched PLA showed a pronounced cold crystallization behavior (Tcc = 101 °C). The Tcc was decreased by 7 °C after addition of the OXA and even disappeared after hot-stretching regardless of the OXA. Meanwhile, the crystallinity was increased from 3% to 50%. Thus, both the OXA and hot-stretching were beneficial to crystallization. Although the melting temperatures of the PLA were hardly changed (Tm = 170 °C), shoulder melting peaks were detected, notably at higher stretching ratios due to a melting/re-crystallization/re-melting mechanism and the possible different perfections between the shish-kebab and spherulitic crystals.35
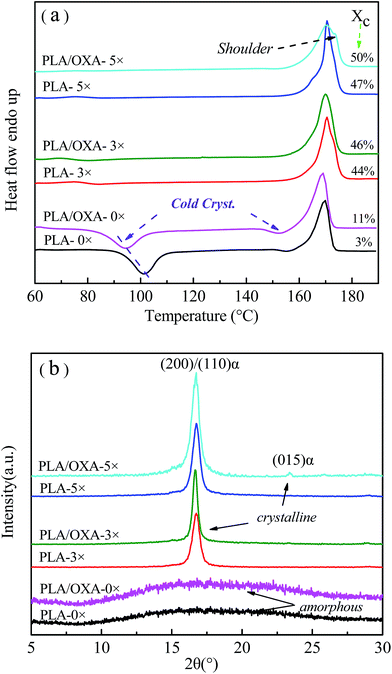 |
| Fig. 5 (a) First heating DSC curves and (b) WAXD patterns of the PLA and PLA/OXA sheets pre-stretched with different ratios at 75 °C. The samples pre-stretched at 80 °C showed similar DSC and WAXD responses that can be found in ESI Fig. S1.† | |
Three crystal forms, i.e., α/δ, β and γ, have been identified for PLA.36,37 The α-form, with a 103 helical chain conformation is grown from the melt or cold crystallization,38–40 while the β-form with a left-handed 31 helical conformation was formed by drawing at high temperatures (175 °C).41 In this work, single α-form crystals were obtained after the pre-stretching regardless of the OXA and temperature (75 or 85 °C), as evidenced by the WAXD patterns (Fig. 5b) where only a sharp diffraction peak at 2θ = 16.6° and an indistinctive peak at 2θ = 23.3° are monitored corresponding to the 200/110 and 015 planes of the PLA α-crystals.38 In comparison, the un-stretched PLA or PLA/OXA are almost amorphous. Therefore, solid-state uniaxial hot-stretching in combination with OXA significantly enhanced the growth of 200/110 planes in the α-form PLA crystals, leading to higher crystallinity.
3.5 Improved heat resistance without extra treatments
Storage modulus (E′) and tan
δ of the PLA and PLA/OXA sheets as a function of temperature are shown in Fig. 5a and b. The E′ values are used to evaluate the heat resistance of the PLA and PLA/OXA sheets (Fig. 5a). The E′ of the un-stretched PLA reduced steeply at around 70 °C due to the glass transition (Tg). Interestingly, the Tg of PLA was increased by 28 °C after hot-stretching in the presence of OXA (Fig. 6b) indicating that the PLA chain mobility is significantly confined due to the orientation and formation of network-like shish-kebab crystals and a higher crystallinity. As a consequence, the E′ of the stretched PLA reduced much less, between 70 and 100 °C, and the E′ of the stretched PLA/OXA was even higher. Taking 100 °C for example, the E′ of stretched the PLA/OXA is 600 MPa compared with 250 and 3 MPa of the stretched and un-stretched PLA, respectively (Fig. 6a). In the literature, an increase in crystallinity and Tg (by 7 °C) of the PLLA/PDLA asymmetric system was achieved by extra annealing at 100 °C, leading to improved heat resistance (E′PLLA/PDLA = 350 MPa at 100 °C).34 Impressively, the high E′ values of the stretched PLA and PLA/OXA specimens persisted up to >150 °C, meaning that the application temperatures of PLA may be broadened from 70 °C to at least 150 °C by the combination of needle-like nucleating templates and solid-state uniaxial hot-stretching technology.
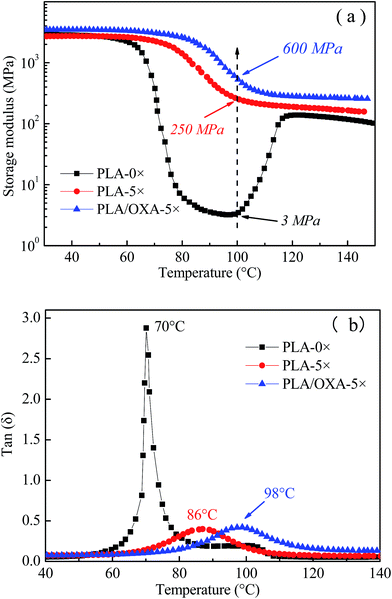 |
| Fig. 6 (a) Storage modulus and (b) tan δ of the PLA and PLA/OXA sheets as a function of temperature. The sheets were pre-stretched with different ratios at 75 °C and the tan δ peak temperatures are referred to as the Tg of PLA. | |
4. Conclusions
High-performance PLA composites were obtained by solid-state uniaxial hot-stretching technology in the presence of needle-like OXA nucleating templates. The significantly improved mechanical performances, such as high tensile strength and toughness, are associated with a unique network-like shish-kebab crystal structure drivingly formed by the hot-stretching and the needle-like nucleating templates. Meanwhile, the increase in Tg (by ∼30 °C) and crystallinity (by ∼50%) and the unique network-like shish-kebab crystal structures lead to the superior heat-resistant properties. The high strength (Ts > 120 MPa), toughness (Eb > 25%) and heat-resistant temperature (>150 °C) of the resulting PLA composites may create applications for PLA in engineered plastics.
Acknowledgements
This work is supported by the Natural Science Foundation of Jiangsu Province (BK20130147, BK20130144), the National Natural Science Foundation of China (51573074) and the Fundamental Research Funds for the Central Universities (JUSRP51624A).
References
- L. T. Lim, R. Auras and M. Rubino, Prog. Polym. Sci., 2008, 33, 820–852 CrossRef CAS.
- R. M. Rasal, A. V. Janorkar and D. E. Hirt, Prog. Polym. Sci., 2010, 35, 338–356 CrossRef CAS.
- S. M. Martinez, R. A. Lopez and J. M. Lagaron, Biomacromolecules, 2012, 13, 3887–3899 CrossRef PubMed.
- J. K. Kim, D. J. Park, M. S. Lee and K. J. Ihn, Polymer, 2001, 42, 7429–7441 CrossRef CAS.
- O. Martin and L. Avérous, Polymer, 2001, 42, 6209–6219 CrossRef CAS.
- V. M. Hiljanen, T. Karjalainen and J. Seppälä, J. Appl. Polym. Sci., 1996, 59, 1281–1288 CrossRef.
- K. S. Anderson, K. M. Schreck and M. A. Hillmyer, Polym. Rev., 2008, 48, 85–108 CrossRef CAS.
- H. Liu and J. Zhang, J. Polym. Sci., Part B: Polym. Phys., 2011, 49, 1051–1083 CrossRef CAS.
- G. Kfoury, J. Raquez, F. Hassouna, J. Odent, V. Toniazzo, D. Ruch and P. Dubois, Front. Chem., 2013, 1, 1–45 CAS.
- P. Ma, D. G. Hristova-Bogaerds and J. G. P. Goossens, Eur. Polym. J., 2012, 48, 146–154 CrossRef CAS.
- D. Li, B. Shen and Z. Weng, J. Macromol. Sci., Part B: Phys., 2011, 50, 2050–2059 CrossRef CAS.
- D. Yuan, K. Chen and C. Xu, Carbohydr. Polym., 2014, 113, 438–445 CrossRef CAS PubMed.
- W. Zhang, L. Chen and Y. Zhang, Polymer, 2009, 50, 1311–1315 CrossRef CAS.
- L. Han, C. Han and L. Dong, Polym. Int., 2013, 62, 295–303 CrossRef CAS.
- L. Han, C. Han and L. Dong, Polym. Compos., 2013, 34, 122–130 CrossRef CAS.
- H. Li and M. A. Huneault, Polymer, 2007, 48, 6855–6866 CrossRef CAS.
- N. Ogata, G. Jimenez, H. Kawai and T. Ogihara, J. Polym. Sci., Part B: Polym. Phys., 1997, 35, 389–396 CrossRef CAS.
- S. S. Ray, K. Yamada, M. Okamoto, Y. Fujimoto, A. Ogami and K. Ueda, Polymer, 2003, 44, 6633–6646 CrossRef.
- J. P. Mercier, J. J. Aklonis, M. Litt and A. V. Tobolsky, J. Appl. Polym. Sci., 1965, 9, 447–459 CrossRef CAS.
- C. Samuel, J. Cayuela and I. Barakat, ACS Appl. Mater. Interfaces, 2013, 5(22), 11797–11807 CAS.
- L. Li, T. Zhou and J. Liu, Polym. Adv. Technol., 2015, 26(1), 77–84 CrossRef CAS.
- Y. Koike and M. Cakmak, Macromolecules, 2004, 37(6), 2171–2181 CrossRef CAS.
- G. Kokturk, T. F. Serhatkulu, M. Cakmak and E. Piskin, Polym. Eng. Sci., 2002, 42, 1619–1628 CAS.
- P. B. Smith, A. Leugers and S. Kang, J. Appl. Polym. Sci., 2001, 82(10), 2497–2505 CrossRef CAS.
- H. Bai, C. Huang and H. Xiu, Polymer, 2014, 55(26), 6924–6934 CrossRef CAS.
- H. Bai, W. Zhang, H. Deng and Q. Fu, Macromolecules, 2011, 44(6), 1233–1237 CrossRef CAS.
- H. Bai, C. Huang, H. Xiu and Q. Fu, Biomacromolecules, 2014, 15(4), 1507–1514 CrossRef CAS PubMed.
- P. Ma, Y. Xu and D. Wang, Ind. Eng. Chem. Res., 2014, 53(32), 12888–12892 CrossRef CAS.
- H. Tsuji, Biomaterials, 2003, 24, 537–547 CrossRef CAS PubMed.
- A. Sodergard and M. Stolt, Prog. Polym. Sci., 2002, 27, 1123–1163 CrossRef CAS.
- P. Ma, Y. Xu, D. Wang, W. Dong and M. Chen, Ind. Eng. Chem. Res., 2014, 53, 12888–12892 CrossRef CAS.
- B. Larin, C. A. Avila-Orta, R. H. Somani, B. S. Hsiao and G. Marom, Polymer, 2008, 49, 295–302 CrossRef CAS.
- H. E. H. Meijer and L. E. Govaert, Prog. Polym. Sci., 2005, 30, 915–938 CrossRef CAS.
- P. Ma, T. Shen, P. Xu, W. Dong, P. J. Lemstra and M. Chen, ACS Sustainable Chem. Eng., 2015, 3(7), 1470–1478 CrossRef CAS.
- T. Ke and X. Sun, J. Appl. Polym. Sci., 2003, 89(5), 1203–1210 CrossRef CAS.
- F. Luo, C. Geng and K. Wang, Macromolecules, 2009, 42(23), 9325–9331 CrossRef CAS.
- J. Kotek, I. Kelnar and J. Baldrian, Eur. Polym. J., 2004, 40(4), 679–684 CrossRef CAS.
- J. Zhang, Y. Duan, H. Sato, H. Tsuji, I. Noda, S. Yan and Y. Ozaki, Macromolecules, 2005, 38, 8012–8021 CrossRef CAS.
- P. Pan, W. Kai and B. Zhu, Macromolecules, 2007, 40, 6898–6905 CrossRef CAS.
- W. Hoogsteen, A. R. Postema and A. J. Pennings, Macromolecules, 1990, 23, 634–642 CrossRef CAS.
- J. Puiggali, Y. Ikada, H. Tsuji, L. Cartier, T. Okihara and B. Lotz, Polymer, 2000, 41, 8921–8930 CrossRef CAS.
Footnote |
† Electronic supplementary information (ESI) available. See DOI: 10.1039/c6ra11815a |
|
This journal is © The Royal Society of Chemistry 2016 |