DOI:
10.1039/C6RA11450D
(Paper)
RSC Adv., 2016,
6, 67118-67126
Efficacy of polyethylene Interpolymer membranes for fluoride and arsenic ion removal during desalination of water via electrodialysis†
Received
3rd May 2016
, Accepted 28th June 2016
First published on 30th June 2016
Abstract
Herein, we report the comparative fluoride and arsenic ion removal efficacies of three different pairs of ion exchange membranes viz. indigenously developed polyethylene Interpolymer based ion-exchange membranes, commercial Ionsep and Fujifilm type I membranes during desalination via the electrodialysis process. Different experiments were conducted in a domestic electrodialysis unit (effective membrane area of 20 cm × 10 cm) using 30 pairs of each type of membrane pair. Both the Interpolymer and commercial Fujifilm type I membranes could effectively reduce the fluoride concentration (14.4 ppm) present in ground water of Rajasthan, India to a drinking water permissible limit (<1.5 ppm). The commercial Ionsep membrane could not reduce such a high concentration of fluoride ion to a permissible limit of drinking water. This is attributed to the moderate water uptake and high fluoride ion transport number of the Interpolymer and commercial Fujifilm type I membranes compared to that of the Ionsep membrane. All three different types of ion-exchange membranes could effectively reduce arsenate ion concentrations of 800 ppb (the maximum concentration present in Indian water) to a permissible limit for drinking water (∼10 ppb). The ion removal efficacy of the membranes depends on the concentration of both the fluoride and arsenate ions present in salt water.
Introduction
The demand for fresh ground water is increasing day by day worldwide. Unfortunately, ground water is contaminated with different ions such as fluoride, arsenic, nitrate etc. Fluoride, in addition to arsenic and nitrate ions is one of the contaminants of water for human consumption which, as stated by the World Health Organization (WHO), causes large-scale health problems. The amount of fluoride ions in drinking water should be <1.5 ppm as per guidelines provided by WHO, above which can cause various health problems such as birth, reproduction, and immunological defects, and dental and skeletal fluorosis.1,2 In India, more particularly in Rajasthan and Gujarat, the ground water is contaminated with fluoride ions. The concentration of fluoride ions present in ground water varies from 3 ppm to 15 ppm. The arsenic ion is also a toxic and carcinogenic element for human health.3 WHO has recommended a safe arsenic permissible limit in drinking water of 10 ppb. In India, West Bengal and Assam are the two most arsenic affected states. The arsenic contamination varies from 200 ppb to 800 ppb whereas the total dissolved solid content (TDS) of water is only 200–500 ppm. Therefore, removal of fluoride and arsenic ions from drinking water is desirable to maintain a healthy life in the above mentioned states.
Membrane technologies such as ultrafiltration, nanofiltration (NF) and thin film composite reverse osmosis (TFC RO) technologies are used for the successful removal of fluoride (F−) ion from water.4 Removal of F− (17.9 ppm) ion from ground water of Morocco using different NF and RO membranes has been reported.5 F− ion removal with low energy RO membranes as well as with ceramic membranes was also reported.6,7 The separation process using RO or NF membranes is robust but the main drawback of these two technologies is that both NF and TFC RO membranes undergo fouling.
The electrodialysis (ED) process using a cation exchange membrane (CEM) and anion exchange membrane (AEM) has been used for water desalination.8–10 The advantages of ED over RO and NF membrane based separation techniques include low energy costs, minimum water loss through reject water and higher membrane life due to less fouling.11 As regards water desalination, ED is advantageous over the RO process when the TDS of water is up to 3000 ppm. For example, the ED process requires 75%, 50% and 30% less energy than the RO process at feed water TDS values of 1000, 2000 and 3000 ppm respectively.11 The ED process has been used for the removal of F− ion from Moroccan ground water (TDS = 1100–3000 ppm and F− ion concentration = 1.8–4.5 ppm).12–14 A combination of an adsorption and ED process has also been reported for the removal of F− ion.15 Removal of F− from geothermal water of Turkey,16 ground water of Pine Hill Farm, Australia17 (TDS = 5000 ppm, F− ion concentration = 2.8 ppm) and artesian well-water in Brazil18 (F− concentration = 5–20 ppm) by the ED process has also been reported.16–18 In all the above mentioned ED processes16–18 commercial Neosepta16,17 and Asahi Glass Co membranes18 were used. Removal of F− ion from brackish water of India (TDS = 2100–4800 ppm with an additional F− ion concentration of 5–20 ppm) by the ED process using a polyethylene–polystyrene Interpolymer based CEM and AEM was also reported.19 The above mentioned membranes reduced the F− concentration to <1.5 ppm. The main drawback of the polyethylene–polystyrene Interpolymer based AEM is its preparation by chloromethylation of a styrene moiety using hazardous chloromethyl methyl ether (CME).19 Since CME is a banned chemical in India, the preparation of AEM using CME is restricted.20
Meanwhile, arsenate ion removal (As5+) from ground water of China using a commercial nanofiltration membrane (Toray) has been reported.21,22 The removal efficiency of As5+ ions depends on the operating conditions.23,24 TFC RO membranes have also been used for the removal of As5+ from ground water.25,26 There is only one report of removal of As3+ by the ED process from ground water using commercial SKS membranes.27
The above literature reports reveal that there is no report of removal of high concentrations of F− ion (around 15 ppm) at low water TDS (500–680 ppm). The removal of As5+ from water by the ED process is not reported in the literature. Therefore, the main objective in this work is the desalination of brackish water of TDS = 500–2000 ppm containing additional amounts of hazardous F− ion (12–14.4 ppm) by the ED process. Our other objective is the removal of arsenate ions (As5+) in concentrations of 200–800 ppb ions from water (TDS = 200–500 ppm) by the ED process. The indigenously developed polyethylene polystyrene Interpolymer based CEM and polyethylene poly-4-methyl styrene Interpolymer based AEM were used in both the experiments. The performance of the polyethylene Interpolymer based membranes towards the removal of F− and As5+ ions during water desalination was compared with two different types of commercial ion exchange membranes by the ED process under similar experimental conditions.28 Polyethylene Interpolymer based membranes are mechanically strong and stable towards exposure to acids and alkalis. We reported the use of these Interpolymer membranes for brackish water desalination via ED28 and ultrapure water production via electrodeionization.29 Herein, desalination along with defluoridation efficiencies of the indigenous polyethylene based membranes, commercial Ionsep and Fujifilm type I membranes were compared by performing the desalination of ground water as well as synthetic water samples (containing 12–14.4 ppm F−) by the ED process at 1.5 volts per cell pair applied potential. It was observed that both the indigenously developed Interpolymer based membrane and commercial Fujifilm membranes could effectively reduce the F− ion concentration to 1.5 ppm whereas the commercial Ionsep membrane could reduce F− ion concentration to ca. 2.34 ppm. The effect of hydration of the membrane on the F− ion removal efficiency has been established. Similarly the dearsenification efficiency of the above mentioned three different membranes was evaluated by the ED process during As5+ (concentration = 200–800 ppb) ion removal from water (TDS = 200–500 ppm) at 1.5 volts per cell pair applied potential. All the three different types of membranes could effectively remove As5+ ions (concentration up to 800 ppb) which is the maximum concentration of As5+ present in Indian ground water. The power consumption and current efficiency during F− and As5+ ions removal have been compared and correlated with the ion transport number and/or degree of hydration of the above membranes.
Experimental
Materials
4-Methylstyrene (4-MS) (96%) and divinyl benzene (DVB) (80%) were purchased from Sigma-Aldrich Chemicals and used as received. Styrene (St), N-bromosuccinimide (NBS) and benzoyl peroxide (BPO) were purchased from TCI, Japan. Film grade high density polyethylene (HDPE) and linear low density polyethylene (LLDPE) were purchased from Reliance Industries, India. Sodium fluoride, ethylene dichloride, xylene, toluene, and chlorosulfonic acid were purchased from S D Fine Chemicals India. Sodium arsenate dibasic heptahydrate, 98% (Na2HAsO4·7H2O) was purchased from Sigma Aldrich. Zinc powder and sulfamic acid were purchased from Finar Chemicals, India. The polyethylene–polystyrene Inter-polymer based cation exchange membrane (CEM) and polyethylene–poly-4-methylstyrene Interpolymer based anion exchange membrane (AEM) have been designated as CEMInter and AEMInter respectively. CEMs purchased from Ionsep and Fujifilm are designated as CEMIonsep and CEMFujifilm. AEMs purchased from Ionsep and Fujifilm are designated as AEMIonsep and AEMFujifilm. Ground water of Ganeshpura, Jhunjhunu, Rajasthan, in India of TDS 680 ppm contains fluoride (F− = 14.4 ppm), chloride (Cl− = 300 ppm), sulfate (20 ppm), carbonate (8 ppm), bicarbonate (4 ppm), calcium (10 ppm), magnesium (10 ppm), sodium (280 ppm) and potassium ions (40 ppm).
Preparation of Interpolymer based CEMInter and AEMInter
CEMInter was prepared by a three step process starting from the free radical polymerization of St and DVB in the presence of an 80
:
20 w/w ratio of HDPE and LLDPE. BPO (1 wt%) was used as an initiator and a mixture of xylene and toluene was used as the solvent. The PE/PSt Interpolymer pellets were converted to PE/PSt Interpolymer film by a blow extrusion process. CEMInter was prepared by treatment of the PE/PSt Interpolymer film with an 80
:
20 (v/v) mixture of ethylene dichloride (EDC) and chlorosulfonic acid at room temperature for 3.5 h. AEMInter was prepared by a four step process starting from polymerization of 4-MS, DVB in the presence of a 90
:
10 w/w mixture of HDPE and LLDPE. A mixture of xylene and toluene was used as the solvent and 1 wt% BPO was used as an initiator. The PE/P4-MS Interpolymer pellets were converted to PE/P4-MS Interpolymer film by a blow extrusion process. AEMInter was prepared by treatment of the PE/P4-MS Interpolymer film with NBS and BPO in the presence of EDC at 70 °C for 48 h. The quaternary ammonium moiety in the brominated film was incorporated after the reaction with trimethyl amine for 6 h at room temperature. The detailed procedure of the preparation of both types of Interpolymer pellets and Interpolymer films has been mentioned in ESI.† Both the CEMInter and AEMInter were washed consecutively with 1 M HCl and 1 M NaOH followed by washing with a large excess of distilled water. The membranes were stored in 0.1 M NaCl solution before use in the ED unit.
Characterization
Physical and electrochemical characterization of CEMs and AEMs. The physical characterization such as water uptake (%) of all the membranes used in this work was determined by measuring the gain of weight of the membranes by using the procedure reported by us earlier.28,29 The ion-exchange capacity (IEC) of the CEMs and AEMs was determined by a titration method.28,29 The membrane conductivity (Km) and transport number (t+/t−) of all the CEMs and AEMs used in this work were measured by measuring the membrane resistance and membrane potential respectively.28,29 All the detailed characterization methods have been provided in the ESI.†
ED experiments. Water desalination using AEMInter and CEMInter was determined by the ED process using an in-house prepared ED cell. Scheme 1 shows the design of the ED unit and the membrane arrangements in the cell. The electrode housing, cathode and anode were made of a rigid PVC sheet, stainless steel and titanium tantalum respectively. A parallel-cum-series flow arrangement of water through the membranes was used in the ED unit. Peristaltic pumps were used to recirculate the inlet and outlet streams. Three different compartments such as the diluate, concentrate and electrode wash compartments exist in the ED unit. Both of the electrode wash compartments were circulated with dilute Na2SO4 solution (0.01% w/v) to avoid an oxidation reduction reaction on the electrode surface. Desalination experiments were carried out in the ED unit with an effective membrane area of 200 cm2 using 30 pieces of each type of membrane (total membrane area = 12
000 cm2) using salt solutions with varying TDS and varying F− (12.2–14.4 ppm) and arsenate (As5+) (200–800 ppb) ion concentrations under the recirculation mode of operation. Equal volumes (1 L) of salt solution circulated in both the diluate and concentrate compartments. A fixed 45 volts potential (1.5 volts per cell pair) was applied between the electrodes in all of the experiments by means of an AC-DC rectifier. The change of current value with desalination time was recorded in all the experiments. Similarly separate defluoridation/dearsenification experiments were carried out in the same ED unit with commercial Ionsep and Fujifilm type I membranes under similar experimental conditions.
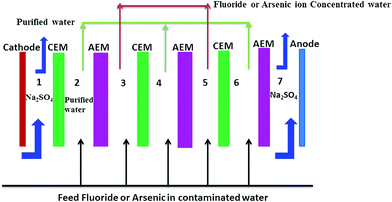 |
| Scheme 1 Schematic arrangement of membranes in the ED unit. | |
Determination of F− and As5+ concentration in water after the ED process. The F− ion concentration in both the diluate and concentrate compartments was analyzed by a fluoride selective electrode (Orion BNWP 9609) attached to a pH meter (Orion Versa Star from Thermo Scientific). The As5+ ion concentration in both the diluate and concentrate compartments was measured in a PerkinElmer atomic absorption spectrophotometer (AAS-100) at 193.7 nm wavelength using the flame-FIAS technique.30
Determination of power consumption and current efficiency. Power consumption and current efficiency are two important parameters that judge the suitability of any power driven separation process. Power consumption (W in kW h kg−1) during the ED process is defined as the amount of energy needed to transport one kg of NaCl from the diluted compartment to the concentrated compartment. W has been calculated using the following equation:9–11,28,29 |
 | (1) |
where V is the applied voltage; I is the current (A); dt is the time (h) allowed for the desalination process; and w is the weight of salt (g) removed.The current efficiency (CE) is defined as the fraction of the current transported by the specific ion and has been calculated using the following equation:9–11,28,29
|
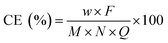 | (2) |
where
F is the Faraday constant (26.8 A h mol
−1);
M is the molecular weight of NaCl (58.5);
N is the number of membrane pairs used in the ED unit (30 pairs);
Q is the amount of electricity passed throughout the system (A h) during the defluoridation and dearsenification experiments. It is noted that both the
W and CE (%) values were calculated considering NaCl removal, but the ED experiments were continued until the F
− and As
5+ concentrations in the diluate compartment reached <1.5 ppm and 10 ppb respectively during all the defluoridation and dearsenification studies.
Results and discussions
Physical and electrochemical characterization of the different ion-exchange membranes
Three different pairs of membranes viz. (i) Inter polymer-based CEMInter/AEMInter, (ii) CEMIonsep/AEMIonsep and (iii) CEMFujifilm/AEMFujifilm were employed in the ED unit for the purification of water (containing NaCl and As5+ or F−). The CEMInter consists of a –SO3H containing crosslinked network of polyethylene and polySt and polyDVB.28 The AEMInter consists of a polyethylene based cross-linked network of poly(4-MS) and polyDVB in which the –CH3 moieties were converted to quaternized amine groups by the process reported earlier by us.28 The detailed FT-IR characterization of the CEMInter and AEMInter has been mentioned in the ESI (Fig. S1†). Table 1 summarizes the water uptake, IEC, Km and t values (Na+, Cl−, and F−) of the different ion-exchange membranes used here for the ED experiments.
Table 1 Physical and electrochemical properties of the CEMs and AEMs used for this work
Samples |
Thickness (mm) |
Water uptake (%) |
IEC (meq. g−1) |
Km (mS cm−1) |
t+ or t− (Na+ and Cl− or F−) |
Na+/Cl− |
Na+/F− |
CEMInter |
0.20 |
29 |
2.45 |
2.86 |
0.94 |
0.93 |
AEMInter |
0.20 |
20 |
1.30 |
1.12 |
0.92 |
0.91 |
CEMIonsep |
0.40 |
40 |
2.00 |
3.70 |
0.86 |
0.85 |
AEMIonsep |
0.40 |
40 |
2.20 |
3.20 |
0.85 |
0.82 |
CEMFujifilm |
0.14 |
30 |
2.07 |
2.17 |
0.93 |
0.91 |
AEMFujifilm |
0.13 |
25 |
2.12 |
2.69 |
0.91 |
0.89 |
The average water uptake values (hydration) of the CEMInter/AEMInter pair (24.5%) is close to the average hydration value of the CEMFujifilm/AEMFujifilm pair (27.5%) and lower than the CEMIonsep/AEMIonsep pair (40%). On the other hand, the average IEC values for these membranes are 1.875 meq. g−1, 2.095 meq. g−1 and 2.1 meq. g−1 respectively whereas the average t[(t+ + t−)/2] values are 0.93, 0.92 and 0.855 when NaCl was used as an electrolyte. The lower water uptake of the Inter-polymer and Fujifilm membranes lowers the back diffusion of ions with water molecules and maintained good transportation of the ions.9–11 A high degree of hydration (water uptake) of the Ionsep membranes lowers the t values of all the ions. The t value of F− was the lowest with Ionsep. For the removal of F− ions, it is essential to compare the t values of these membranes using NaF as an electrolyte. The average t values during F− ion transportation were 0.92, 0.90 and 0.835 for the Interpolymer, Fujifilm and Ionsep membranes which are slightly lower than the average t values obtained during Cl− transport. This small variation in average t values affects the F− transportation behaviour by the above membranes a lot. This transportation behaviour may be due to a higher hydration energy of the F− ions than that of Cl− ions.31
F− removal by the ED process
Water desalination experiments with a TDS of 540–2186 ppm and an additional F− ion content of 12.1–12.6 ppm have been carried out by ED using indigenous Interpolymer based and two commercial membranes (Ionsep and Fujifilm type I) at an applied potential of 1.5 volts per cell pair under recirculation mode of operation. Separate desalination experiments were also carried out using these membrane pairs with water samples (F− content = 14.4 ppm and TDS = 680 ppm) collected from Ganeshpura, Jhunjhunu, Rajasthan, India. Fig. 1A–C shows the remaining F− concentration with ED time plots in the diluate compartment using three different types of ion exchange membranes.
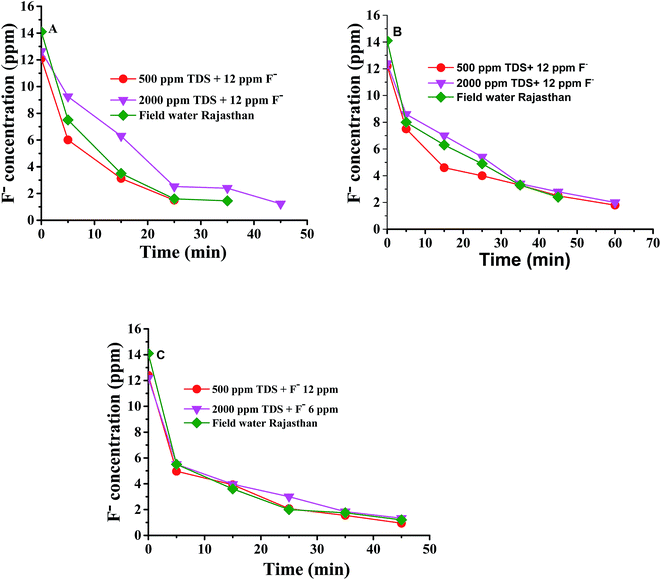 |
| Fig. 1 F− concentration in the diluate compartment with time during ED with the (A) Interpolymer (B) Ionsep and (C) Fujifilm-type I membranes. | |
It is seen from Fig. 1 that the concentration of F− was brought down from 14.4 ppm to <1.5 ppm in the diluate compartment at both high and low water TDS values with the Interpolymer and commercial Fujifilm membranes. The commercial Ionsep membrane could not reduce the F− concentration to <1.5 ppm by ED. It may take a long time to reduce the F− concentration to <1.5 ppm with commercial Ionsep membranes. It has been observed that 90, 84 and 91% of the F− ions have been removed from the ground water sample from Rajasthan by the Interpolymer, Ionsep and Fujifilm type I membranes respectively. Fig. 2A–C show the decrease of TDS of water in the diluate compartment with defluoridation time for the Interpolymer, commercial Ionsep and Fujifilm-type I membranes during the desalination of water containing 500–2000 ppm NaCl and 12 ppm F− ions. Fig. 2A–C also includes the desalination of ground water samples from Rajasthan carried out with the above three membranes.
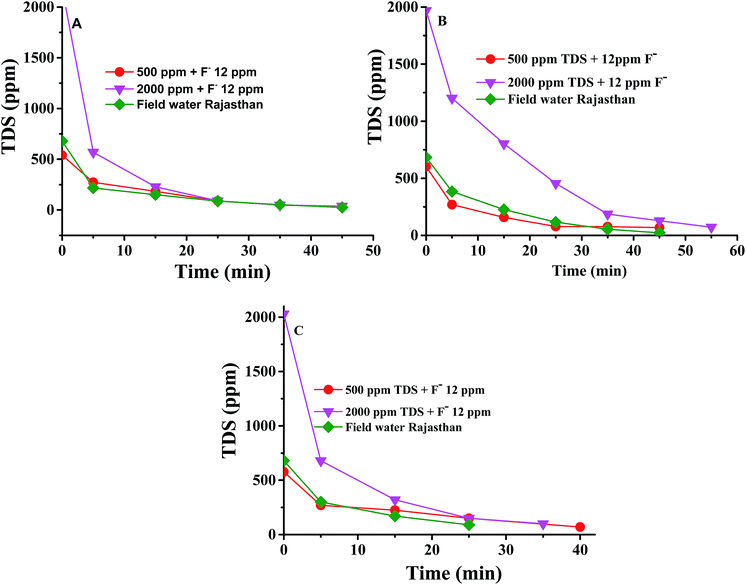 |
| Fig. 2 TDS of the diluate compartment with time during the ED experiment with the (A) Interpolymer (B) Ionsep and (C) Fujifilm-type I membranes. | |
Fig. 2 thus indicates that the TDS in water also gets lowered to the drinking level limit during defluoridation. Hence, the Interpolymer and Fujifilm type I membranes are best suited for the desalination of water containing harmful F− ions to the WHO permissible limit.
Table 2 shows the comparative F− ion removal efficacies of different types of ion-exchange membranes from water of different TDS, additionally contaminated with different concentrations of F−. The unit running time for our experiments (Table 2, entries 6–11) was 45–60 minutes.
Table 2 Comparative F− removal efficacies of the different types of ion-exchange membranes during desalination via the ED process
Type of membrane used |
Source of water |
Water uptake (%) |
Feed water TDS (ppm) |
Feed water F− (ppm) |
Final TDS (ppm) of water in the diluate compartment |
Final F− (ppm) in the diluate compartment |
Reference |
ACS, Neosepta |
Morocco |
20–30 |
1127 |
1.80 |
656 |
0.50 |
24 |
ACS, Neosepta |
Morocco |
20–30 |
1127 |
4.50 |
594 |
1.00 |
24 |
ACS, Neosepta |
Pine Hill Farm, Australia |
20–30 |
4255 |
2.80 |
500 |
1.50 |
28 |
ACS, Neosepta |
Morocco |
20–30 |
3000 |
3.00 |
400 |
0.63 |
27 |
AMX/CMX Neosepta |
Balcova geothermal, Turkey |
25–30 |
1026 |
7.72 |
33 |
0.06 |
26 |
Interpolymer |
Rajasthan, India |
24.5 |
680 |
14.40 |
25 |
1.45 |
This work |
Fujifilm-type I |
Rajasthan, India |
27.5 |
680 |
14.40 |
90 |
1.33 |
This work |
Ionsep |
Rajasthan, India |
40.0 |
680 |
14.40 |
22 |
2.34 |
This work |
Interpolymer |
NaCl solution |
24.5 |
2186 |
12.60 |
38 |
1.23 |
This work |
Fujifilm-type I |
NaCl solution |
27.5 |
2080 |
12.20 |
43 |
1.23 |
This work |
Ionsep |
NaCl solution |
40.0 |
1970 |
12.40 |
72 |
1.60 |
This work |
It has been observed from Table 2 (entries 6–11), that both the Interpolymer as well as commercial Fujifilm type I membranes can reduce high F− ion concentrations (12.2–14.4 ppm) to <1.5 ppm at both high and low water TDS (680–2186 ppm) under similar experimental conditions (such as the ion concentration of the source water and the ED unit operating times are the same). On the other hand, commercial Ionsep membranes failed to reduce the F− concentration to <1.5 ppm when the concentration of F− ions in salt water was 12.4 ppm or above. Other commercial membranes (ACS and AMX Neosepta, Table 2, entries 1–5) can effectively reduce the F− ion concentration to the permissible limit of drinking level when the water contains a relatively low concentration of F− (1.8–7.72 ppm). Data of F− ion removal from salt water with the ACS and AMX Neosepta membranes are not available when the F− ion concentration is ca. 12.2 ppm or above.
The inferior F− ion removal efficacy of the Ionsep membrane compared to the Interpolymer and Fujifilm type I membranes at a F− concentration of ca. 12 ppm in salt water is due to the comparatively high water uptake of the former membrane compared with that of the latter membranes. Therefore, the back diffusion of ions along with water is enhanced which lowers the transport number of the Ionsep membrane and hence, the efficacy towards F− ion removal is reduced (Table 1) by this membrane.9–11
Maximum F− ion removal efficiency of the Ionsep membranes by the ED process
Since the commercial Ionsep membranes cannot reduce high concentrations of F− (ca. 12 ppm or above) to the permissible limit of drinking water (<1.5 ppm), it is necessary to find out the maximum amount of dissolved F− ion that can be removed by this membrane from water by the ED process. Therefore, additional ED experiments were conducted with water with TDS = 500 ppm and containing varying amounts of F− (0.5 to 6 ppm) under the recirculation mode of operation. The applied potential used was 1.5 volts per cell pair. The results are presented in Table 3.
Table 3 Defluoridation results by ED at low F− concentration using the commercial Ionsep membrane
Serial no |
Time (min) |
Initial TDS (ppm) |
Required power consumption (watt min) |
Final TDS (ppm) |
Initial F− (ppm) |
Final F− (ppm) |
1 |
35 |
500 |
1.25 |
109 |
0.5 |
0.051 |
2 |
35 |
500 |
1.25 |
98 |
1.0 |
0.199 |
3 |
35 |
500 |
1.25 |
89 |
6.0 |
0.672 |
It is observed from Table 3 that the commercial Ionsep membrane at a fixed power consumption (1.25 watt min) can remove almost 89.8% and 88.8% of F− ion from the water when 0.5 and 6 ppm F− ion is present in water. The final F− concentration in the diluate compartment was <1.5 ppm. Therefore, if the contaminated F− ion concentration increases from 6 ppm, a long time (higher power consumption) may be required to bring the F− ion concentration to the drinking water permissible limit (<1.5 ppm).
Transport behavior of F− ions through different membranes
We discussed earlier that (Table 1) the average t of F− ions is marginally and consistently lower than that of Cl− or Na+ ions for all the membranes. Hence, it is essential to find out the transport behaviour of Cl− and F− (when present in small quantities in water) through these three different types of membranes. Hence, two separate ED experiments were conducted using feed water containing (i) 500 ppm KCl and 10 ppm NaF and (ii) 500 ppm KCl and 10 ppm NaCl. The concentration of F− ions in the case of experiment (i) and concentration of Na+ ions (which is equivalent to the concentration of Cl− ions) in the case of experiment (ii) in the diluate compartments were measured at different time intervals. Fig. 3A and B show the F− concentration vs. time and concentration of Na+ (equivalent to Cl−) vs. time plots respectively for all three different membranes. These experiments indicate that the removal of a small amount of Na+ (equivalent to Cl−) is faster by all three membranes than the removal of a similar amount of F− from water. Thus a small change in the transport property of the ions (marginally lower t of F− compared to that of Cl−) has a greater effect on the F− ion removal efficacy of the membranes. Such small differences in t between F− compared to that of Cl− may be attributed to the higher degree of hydration (hydration energy) of the F− ions compared to that of the Cl− ions.31 On the other hand, the t value of Na+ is thus influenced by the type of counter ion (Table 1). For example the t value of Na+ was somewhat lower when the counter ion was F− (NaF was the electrolyte) than when it was Cl− (NaCl was the electrolyte) for all experiments. This may be the reason for the faster transport of Na+ than that of F−.
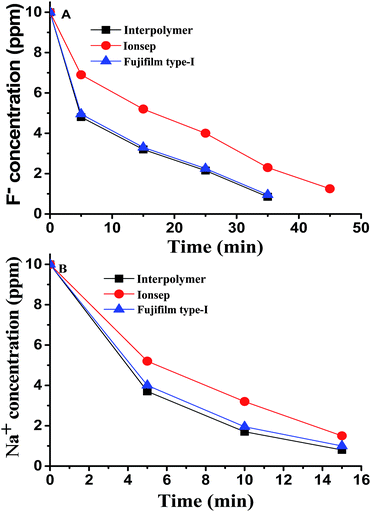 |
| Fig. 3 Concentrations of (A) F− and (B) Na+ in the diluate compartments with time using three different types of membrane. | |
As5+ removal by the ED process
The As5+ removal efficacy of the three different membranes was also assessed by the ED process under the recirculation mode of operation. The experiments were conducted with water TDS = 200 and 500 ppm. Since the ground water of India mostly contains an As5+ concentration of 200–800 ppb, the concentration of As5+ salt was kept at 200 ppb and 800 ppb in the synthetic salt mixture of TDS = 200 ppm and 500 ppm. Fig. 4A and B show the As5+ concentration in the diluate compartment with dearsenification time carried out with water of TDS = 200 ppm (Fig. 4A) and with water TDS = 500 ppm (Fig. 4B) for the Interpolymer, Fujifilm and Ionsep membranes respectively.
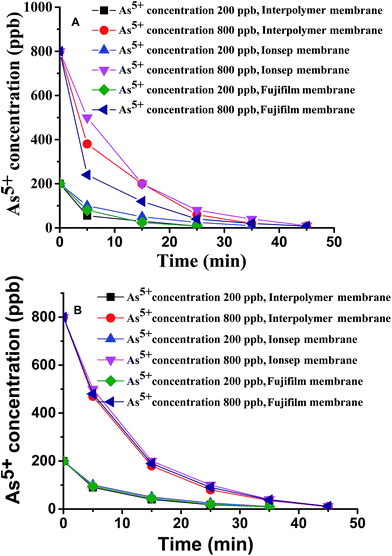 |
| Fig. 4 As5+ concentration in the diluate compartment with time during the ED experiment with all three different types of membranes at different TDS, (A) 200 ppm and (B) 500 ppm. | |
It is clearly observed from Fig. 4A and B that all the three different types of membranes reduce the As5+ concentration from 800 to 10 ppb. The good As5+ ion removal efficacy of the membranes is attributed to the low concentration of As5+ in salt water which was not influenced by the water uptake of the membranes. However, at high concentrations of As5+ in the salt water the water uptake values of the membranes may affect the comparative As5+ ion removal efficacy.
Determination of W and CE values during F− and As5+ ion removal by ED
The feasibility of any separation process via ED is determined by measuring the W and CE (%) values during the process. The W and CE (%) values during F− and As5+ ion removal using three different types of membrane has been measured from the current values obtained at different time intervals during the whole defluoridation and dearsenification processes using eqn (1) and (2) and presented in Tables 4 and 5. The experimental time was dependent on the F− and As5+ ions’ removal rate. The experiment was stopped when the concentration of F− in the diluate compartments was at a minimum viz. <1.5 ppm for all membranes except Ionsep. For Ionsep the final F− ion concentrations achieved in the diluate compartments were 2.40, and 2.34 ppm respectively for entries 5 and 6 in Table 4. Similarly, during As5+ removal the final concentrations of As5+ in the diluate compartments were 8.5, 9.2 and 9.8 for the Interpolymer, Fujifilm and Ionsep membranes.
Table 4 Determination of W and CE values during F− ion removal by the ED process. The experimental time was set to the point when the F− ion concentration in the diluate compartment was at its minimum (<1.5 for a and for b F− concentration = 2.34 and 2.40 ppm)
Types of membranes |
Feed water TDS (ppm) |
F− concentration (ppm) |
W (kW h kg−1) |
CE (%) |
aInterpolymer |
2186 |
12.6 |
0.943 |
75.0 |
aInterpolymer |
680 |
14.4 |
1.346 |
50.0 |
bIonsep |
1970 |
12.4 |
1.898 |
60.0 |
bIonsep |
680 |
14.4 |
2.400 |
30.0 |
aFujifilm-type I |
2080 |
12.2 |
1.200 |
72.0 |
aFujifilm-type I |
680 |
12.4 |
1.430 |
45.0 |
Table 5 Determination of W and CE values during As5+ removal by the ED process. The experiment was stopped when the As5+ concentration reached 10 ppb in the diluate compartment
Types of membranes |
Feed water TDS (ppm) |
As5+ concentration (ppb) |
W (kW h kg−1) |
CE (%) |
Interpolymer |
200 |
800.4 |
1.24 |
74.5 |
Ionsep |
212 |
800.2 |
1.32 |
70.0 |
Fujifilm-type I |
216 |
800.8 |
1.26 |
73.5 |
The W value was decreased and the CE (%) value was increased at comparatively high water TDS for all three different types of membranes. This is usually due to the high ionic concentration of the feed solution which enhanced the current and favours faster F− ion removal.9–11 The obtained W and CE (%) values are comparable during F− removal from water at both high and low water TDS for the Interpolymer and Fujifilm membranes (Table 4, entries 1, 2, 5 and 6). The obtained W value is higher and CE (%) value is lower with the Ionsep membranes (Table 4, entries 3 and 4). This once again supports the slower F− ion transportation with the Ionsep membranes than with the other two membranes.
It has been observed from Table 5 that the W and CE (%) values of all three different types of membranes are not much different for all the membranes during As5+ removal. Strictly, the Ionsep membranes showed a somewhat lower performance than the other two membranes. The low W and high CE achieved with any of the types of membranes during As5+ removal may be due to the low concentration of As5+ (800 ppb) in feed salt water.
Conclusion
We have demonstrated the removal of fluoride ion (14.4 ppm) and arsenate ions (800 ppb) from salt water by the ED process under recirculation mode using three different types of ion-exchange membranes. Indigenously developed Interpolymer and commercial Fujifilm type I membranes effectively reduced the fluoride ion concentration to <1.5 ppm at both low and high water TDS, whereas the commercial Ionsep membrane could not lower the fluoride concentration to the permissible limit of drinking water at high F− concentration (14.4 ppm). Approximately 90%, 91% and 84% of fluoride ions from ground water of Rajasthan were removed by the indigenously developed Interpolymer based, commercial Fujifilm type I, and Ionsep membranes by the ED process. The power consumption values were 1.346 kW h kg−1, 1.43 kW h kg−1, and 2.40 kW h kg−1 and the current efficiency values were 50%, 45%, and 30% respectively during fluoride removal from ground water of Rajasthan (TDS = 680 ppm and F− concentration = 14.4 ppm) with the Interpolymer, Fujifilm type I and Ionsep membranes respectively. The values for the power consumption and current efficiency obtained during fluoride ion removal by the ED process using the indigenously developed Interpolymer based membranes and commercial Fujifilm type I membranes are comparable, whereas for the commercial Ionsep membranes, the power consumption value was higher and the current efficiency value was lower during fluoride removal from water samples from Rajasthan. This may be due to the high water uptake and low fluoride ion transport number of the Ionsep membranes compared to the other two membranes, which allows the back diffusion of fluoride ion with water in the diluate compartment. The commercial Ionsep membrane can reduce fluoride concentrations to < 1.5 ppm when the concentration of fluoride ion present in salt water is up to 6 ppm. On the other hand, all three different types of the ion exchange membrane combinations can effectively reduce arsenate (As5+) concentrations from 800 ppb to <10 ppb by ED. Almost 98.75–99% of arsenate salts are removed by all three types of membranes by the ED process. The power consumption during the arsenate removals for both the Interpolymer and Fujifilm type I membranes was similar and lower than that of the Ionsep membranes.
Acknowledgements
CSIR-CSMCRI communication number 014/2016. Uma Chatterjee acknowledges DST, Govt of India for financial support under the Young Scientist Scheme (YSS/2015/000653). V. Bhadja and J. S. Trivedi acknowledge CSIR network projects (CSC0104 and CSC0105) for providing fellowships. The centralized analytical facility of CSMCRI is acknowledged for analytical support. Mr Rahul Dhabu of Ltek Systems, Nagpur, Maharashtra is acknowledged for providing the ground water samples from Ganeshpura, Jhunjhunu, Rajasthan. Dr V. Kulsrestha, Dr S. Sharma, Mr B. S. Makwana, Mr P. D. Maru and Mr M. N. Parmar are acknowledged for helping during the Interpolymer pellet preparation.
Notes and references
- A. K. Yadav, C. P. Kaushik, A. K. Haritash, B. Singh, S. P. Raghuvanshi and A. Kansal, J. Hazard. Mater., 2007, 142, 77 CrossRef CAS PubMed.
- B. Singh, S. Gaur and V. K. Garg, J. Hazard. Mater., 2007, 144, 147 CrossRef CAS PubMed.
- C. K. Jain and I. Ali, Water Res., 2000, 34, 4304 CrossRef CAS.
- D. Cohen and H. M. Conrad, Desalination, 1998, 117, 19 CrossRef CAS.
- M. Tahaikt, A. Ait Haddou, R. El Habbani, Z. Amor, F. Elhannouni, M. Taky, M. Kharif, A. Boughriba, M. Hafsi and A. Elmidaoui, Desalination, 2008, 225, 209 CrossRef CAS.
- P. Sehn, Desalination, 2008, 223, 73 CrossRef CAS.
- D. Ghosh, M. K. Sinha and M. K. Purkait, Desalination, 2013, 327, 2 CrossRef CAS.
- V. Bhadja, U. Chatterjee and S. K. Jewrajka, RSC Adv., 2015, 5, 40026 RSC.
- U. Chatterjee and S. K. Jewrajka, J. Mater. Chem. A, 2014, 2, 8396 CAS.
- U. Chatterjee, V. Bhadja and S. K. Jewrajka, J. Mater. Chem. A, 2014, 2, 16124 CAS.
- N. C. Wright and A. G. Winter, Desalination, 2014, 352, 82 CrossRef CAS.
- M. Tahaikt, I. Acharya, M. A. Menkouchi Sahli, Z. Amor, M. Taky, A. Alami, A. Boughriba, M. Hafsi and A. Elmidaoui, Desalination, 2006, 189, 215 CrossRef CAS.
- M. A. Menkouchi Sahli, S. Annouar, M. Tahaikt, M. Mountadar, A. Soufiane and A. Elmidaoui, Desalination, 2007, 212, 37 CrossRef CAS.
- Z. Amor, S. Malki, M. Taky, B. Bariou, N. Mameri and A. Elmidaoui, Desalination, 1998, 120, 263 CrossRef CAS.
- M. Arda, E. Orhan, O. Arar, M. Yuksel and N. Kabay, Sep. Sci. Technol., 2009, 44, 841 CrossRef CAS.
- Z. Amor, B. Bariou, N. Mameri, M. Taky, S. Nicolas and A. Elmidaoui, Desalination, 2001, 133, 215 CrossRef CAS.
- L. J. Banasiak, T. W. Kruttschnitt and A. I. Schäfer, Desalination, 2007, 205, 38 CrossRef CAS.
- M. Zeni, R. Riveros, K. Melo, R. Primieri and S. Lorenzini, Desalination, 2005, 185, 241 CrossRef CAS.
- S. K. Adhikary, U. K. Tipnis, W. P. Harkare and K. P. Govindan, Desalination, 1989, 71, 301 CrossRef CAS.
- S. Laskin, M. Kusschner, R. T. Drew, V. P. Capiello and N. Nelson, Arch. Environ. Health, 1971, 23, 135 CrossRef CAS PubMed.
- R. S. Xia, B. Dong, Q. Zhang, B. Xu, N. Gao and C. Causseranda, Desalination, 2007, 204, 374 CrossRef.
- Y. Sato, M. Kang, T. Kamei and Y. Magara, Water Res., 2002, 36, 3371 CrossRef CAS PubMed.
- E. M. Vrjenhoek and J. J. Waypa, Desalination, 2000, 130, 265 CrossRef.
- H. Saitua, M. Campderros and P. A. Perez, Desalination, 2005, 172, 173 CrossRef CAS.
- M. M. Gholami, M. A. Mokhtari, A. Aameri and M. R. Alizadeh Fard, Desalination, 2006, 200, 725 CrossRef CAS.
- M. Sen, A. Manna and P. Pal, J. Membr. Sci., 2010, 354, 108 CrossRef CAS.
- R. M. O. Mendoza, C.-C. Kan, S.-S. Chaung, S. M. B. Pingulong, M. L. P. Dalida and M.-W. Wan, J. Environ. Sci. Health, Part A: Toxic/Hazard. Subst. Environ. Eng., 2014, 49, 545 CrossRef CAS PubMed.
- V. Kulshrestha, U. Chatterjee, S. Sharma, B. S. Makwana and P. D. Maru, Macromol. Symp., 2015, 357, 194 CrossRef CAS.
- V. Bhadja, B. S. Makwana, S. Maiti, S. Sharma and U. Chatterjee, Ind. Eng. Chem. Res., 2015, 54, 10974 CrossRef CAS.
- P. Pal, Z. Ahammad, A. Pattanayek and P. Bhattacharya, Water Environ. Res., 2007, 79, 357 CrossRef CAS PubMed.
- A. Tongraar and B. M. Rode, Phys. Chem. Chem. Phys., 2003, 5, 357 RSC.
Footnote |
† Electronic supplementary information (ESI) available: Preparation of the PE-PSt and PE/P4-MS Inter-polymer film, FT-IR spectra of CEMInter and AEMInter, physical and electrochemical characterization of different membranes. See DOI: 10.1039/c6ra11450d |
|
This journal is © The Royal Society of Chemistry 2016 |