DOI:
10.1039/C6RA11363J
(Paper)
RSC Adv., 2016,
6, 75757-75765
Three-dimensional graphene/Fe3O4-based magnetic solid phase extraction coupled with high performance liquid chromatography for determination of carvedilol in human blood plasma
Received
2nd May 2016
, Accepted 5th July 2016
First published on 5th August 2016
Abstract
A magnetic three-dimensional graphene nano-composite (3D-G–Fe3O4) was synthesized and used as an effective adsorbent for the magnetic solid-phase extraction (MSPE) of carvedilol in human blood plasma samples followed by high performance liquid chromatography (HPLC) equipped with ultraviolet (UV) detection. The combination of magnetic nanoparticles with the porous structure of three dimensional graphene increases the adsorption capacity and surface area for extraction and determination of carvedilol in human blood plasma samples without the need of filtration or centrifugation. The properties and morphology of the synthesized adsorbent were characterized by Fourier transform-infrared spectroscopy (FT-IR), scanning electron microscopy (SEM), transmission electron microscope (TEM), vibrating sample magnetometry (VSM), Raman spectroscopy, X-ray diffraction (XRD), Brunauer–Emmett–Teller (BET), and Barrett–Joyner–Halenda (BJH) techniques. The main experimental parameters affecting the extraction recovery such as desorption conditions, pH of sample solution, dosage of adsorbent, extraction time, and salt concentration were investigated and optimized. Under the optimized experimental conditions, the figure of merit results showed an excellent linear dynamic range (2–1000 ng mL−1), with a determination coefficient (R2) higher than 0.994 and limits of detection (LOD) of 0.5 ng mL−1. Intra- and inter-day relative standard deviations (RSDs) were less than 2.70 and 3.85%, respectively.
Introduction
Carvedilol, 1-(carbazolyl-4-oxy)-3-[2-(O-methoxyphenoxyethyl) amino]-2-propranol, is in a group of drugs called beta-blockers. This family of drugs affects the heart and blood circulation. Carvedilol is a third-generation, nonselective β-blocker that also possesses α1-adrenergic blocking, antioxidant and calcium antagonist properties. Carvedilol blocks both the β1- and β2-adrenergic receptors, resulting in improved myocardial function and attenuation (or reversal) of adverse myocardial remodeling in heart failure.1,2 Carvedilol shows minimal inverse agonist activity when compared with other beta blockers and its use has been shown to decrease morbidity from congestive heart failure.3 In recent years, several methods such as chromatographic and spectroscopic techniques4–6 have been reported for determination of carvedilol in plasma, urine and pharmaceuticals. However, determination of small amounts of carvedilol in complex matrices such as human serum, urine and blood samples is difficult due to insufficient sensitivity and specificity. Therefore, in the most cases a separation and/or a preconcentration technique is required to improve the sensitivity and selectivity of carvedilol analysis method. Some extraction and sample preparation techniques such as dispersive liquid–liquid microextraction,7 nanoparticles amplified flow-injection chemiluminescence,3 and solid phase extraction (SPE)8 have been used for preconcentration and determination of carvedilol.
Among these techniques, SPE is a surface dependent method that enables the preconcentration and purification of analytes from solution by an adsorbent. Solid phase extraction offers some advantages such as simplicity, flexibility, high enrichment factors, absence of emulsion, low cost because of lower consumption of reagents, and more importantly environment friendly.9 In recent years, a type of solid phase extraction technique named magnetic solid-phase extraction (MSPE) that is based on a magnetic adsorbent has been developed for extraction and preconcentration of organic pollutants in environmental analysis,10 heavy metals,11,12 and drugs13,14 due to its high recovery and ease of operation. By taking advantages of combination of magnetic and non-magnetic adsorbent materials, the MSPE technique shows excellent adsorption efficiency. In this technique, a magnetic micro- or nano-particles is dispersed in sample solution. After adsorption completed, the adsorbent can be easily collected from the solution using a magnetic field which is more convenient than tedious filtration or centrifugation procedures. The most important component of the MSPE technique is the adsorbent material, which dominates the sensitivity and selectivity of the method.15 Among the adsorbent materials, nanoscale carbon-based materials have attracted considerable research interests due to their specific large surface area, chemical and thermal stability, ease of surface functionalization or modification, and excellent adsorption capacity.
Graphene is a two-dimensional (2D) monolayer of sp2 bonded carbon atoms. A unique feature of graphene compared with other carbon allotropes is an ultra large specific surface area and flat structure which provides ideal characteristics as an excellent adsorbent.16 However, due to its high hydrophobic property, graphene generally tends to form irreversible agglomerates or even restack to form graphite through strong π–π stacking interactions under certain conditions, which is undesirable for its applications. A change to three-dimensional (3D) graphene from 2D graphene sheets is not only a good solution for aggregation phenomenon, but also introduces desirable characteristics of fast ionic and electronic transport, large open pores, high specific area and strong mechanical properties.17 The combination of hydrophobic and π–π interactions is the guiding force for the formation of 3D random networks between the flexible graphene sheets. When the concentration of graphene oxide (GO) solution was sufficiently high, the cross-linking through partial overlapping of the flexible graphene sheets occurred immediately after GO was reduced, and eventually enough cross-linking sites were generated to form a 3D network with pores.18 The combination of 3D-G with iron oxide nanoparticles makes an efficient adsorbent with high adsorption capacity of 3D-G and separation convenience of magnetic materials.
The aim of this work is the application of 3D-G nano-composite (3D-G–Fe3O4) as an efficient adsorbent followed by high performance liquid chromatography (HPLC) with ultraviolet (UV) detection for the selective extraction and determination of carvedilol in human blood plasma matrix. Several parameters affecting the extraction technique such as desorption condition, pH of the sample solution, dosage of adsorbent, extraction time, and salt concentration were optimized with one-factor-at-a-time technique in order to achieve suitable analytical sensitivity. This is the first report of application of 3D-G–Fe3O4 as an adsorbent for the extraction and determination of carvedilol in human blood plasma sample.
Experimental
Chemicals and materials
Standard carvedilol was obtained from Darou Pakhsh Pharma Company (Tehran-Iran). All chemicals including sulfuric acid (H2SO4, 99.8%), hydrogen peroxide (H2O2, 30%), hydrochloric acid (HCl, 37%), nitric acid (HNO3, 65%), sodium chloride (NaCl), sodium nitrate (NaNO3), sodium hydroxide (NaOH), sodium acetate (CH3COONa), potassium permanganate (KMnO4), iron(III) chloride hexahydrate (FeCl3·6H2O), iron(II) chloride tetrahydrate (FeCl2·4H2O), and ammonia (NH3, 25%) with the purity higher than 99.9%, and also HPLC grade solvents such as ethanol (EtOH), methanol (MeOH), acetonitrile (ACN), dimethyl sulfoxide (DMSO) and dimethyl formamid (DMF) were purchased from Merck Chemicals (Darmstadt, Germany). The acetate buffer (pH 4.0; 0.1 M) solution was prepared by mixing of appropriate amount of glacial acetic acid and sodium acetate. A stock standard solution of carvedilol was prepared by dissolving of 1 mg of this drug in 1 mL of methanol. The carvedilol working standard solutions were prepared by diluting the stock solution with distilled water.
Apparatus
The Agilent Technologies 1260 HPLC system (Santa Clara, CA, USA) consisting of an Agilent 1260 infinity quaternary pump, manual injector (20 μL sample loop) and a variable wavelength detector was used with HPLC Chemstation (Rev B.04.03) software to control the system and for the acquisition and analysis of the data. Chromatographic separations were achieved by using an ultra C18 column (5 μm, 250 × 4.6 mm). The mobile phase was composed of acetonitrile
:
acetate buffer (pH 4.0; 0.1 M) (45
:
55, v/v) being used in an isocratic mode with a flow rate of 1.0 mL min−1. The experiments were carried out at the wavelength of 242 nm for optimization step, analytical method validation and comparison studies. All absorbance measurements in the optimization step were carried out by a UV-2601 (Beijing-China) double beam UV-Vis spectrophotometer fitted with a tungsten lamp as the source using a couple of 1 cm optical path length micro-cuvette (Fisher Scientific, USA). The pH of solutions was measured with a digital WTW Inolab 720 pH meter (Weilheim, Germany) with a combined glass electrode. An ultrasonic water bath with a temperature control (Eeurosonic 4D, Italy) was used to disperse the adsorbent into the solution. The FT-IR spectra were obtained on an Equinox 55 FT-IR spectrometer (Bruker, Bremen, Germany) in the range of 400 to 4000 cm−1. Scanning electron microscopy (SEM) images were obtained with a Hitachi S-4160 machine (Tokyo, Japan) operated at 5.0 kV. The magnetic properties of the adsorbent were investigated by a vibrating sample magnetometer (VSM/AGFM Meghnatis Daghigh Kavir Co., Kashan, Iran) at room temperature by cycling the field from −10 to 10 kOe. The X-ray diffraction (XRD) patterns were obtained using a X'Pert Pro MPD X-ray diffractometer (Almelo, Netherlands) with a Cu Kα radiation (λ = 1.54178 Å). Raman spectra were conducted on a Senterra Dispersive Raman microscope (Bruker, Germany) with a 785 nm wavelength incident laser light. Surface area determination and pore volume and size analysis were performed by Brunauer–Emmett–Teller (BET) and Barrett–Joyner–Halenda (BJH) methods using an ASAP 2000 Surface Area Analyzer (Micromeritics Instrument Corporation). The morphological analysis of GO and 3D-G–Fe3O4 were carried out using JEOL-JEM 2010 transmission electron microscopy (Michigan, USA).
Preparation of the adsorbent
Graphene oxide was prepared from natural graphite powders according to Hummers' method.19 Briefly, 1 g of graphite powder and 0.5 g of NaNO3 were sequentially added into 50 mL of cold H2SO4 under the condition of ice bath and the mixture was stirred for 2 h. Then, 6 g of KMnO4 was added slowly to the mixture while the temperature was maintained at less than 20 °C. Subsequently, the mixture was transferred to a 40 °C oil bath and maintained for 24 h, followed by slow addition of 40 mL ultrapure water under stirring. The final solution was diluted with 160 mL of distilled water with addition of 10 mL H2O2. Next, the mixture was washed with 500 mL HCl (5%) and distilled water. Finally, a solid GO was obtained after drying at 70 °C for 12 h in vacuum oven.
The magnetic 3D-G–Fe3O4 was prepared based on the literature method.20,21 At first, a portion (0.1 g) of GO was added to 50 mL of distilled water and sonicated for 15 min. Next, 0.7 g of FeSO4·7H2O was added to GO aqueous suspension and sonicated for about 5 min. Secondly, NH3 solution was added slowly to the mixture and pH was adjusted to 11 at 90 °C for 6 h in an oil bath without stirring. Finally, the 3D-G–Fe3O4 was obtained by washing with distilled water and then freeze-dried under vacuum to remove adsorbed water.
The procedure
At the extraction step, 40 mL of the sample solution containing 200 ng mL−1 of carvedilol with the pH value of 7 (buffer solution of KH2PO4/NaOH) was placed in a glass container. Then, 30 mg of adsorbent was added into the container contents and placed in an ultrasonic bath (3 min at 25 °C). Afterward, the mixture was exposed to a strong magnet (5 cm × 5 cm × 4 cm, 0.6 T) and the supernatant was discarded. After that, 2 mL of the desorption solvent (MeOH) was added to it and the mixture was sonicated for 1 min to disperse the adsorbent in MeOH. Finally, the mixture was exposed to the strong magnet and the supernatant was analyzed with HPLC (injection volume: 20 μL).
Live subject statement
All experiments were performed in compliance with the relevant laws and institutional guidelines, and Tehran University of Medical Science (Tehran, Iran) has approved the experiments. The informed consent was obtained for any experimentation with human subjects. The human plasma samples were kindly donated by the Iranian Blood Transfusion Organization (Tehran, Iran).
Results and discussion
Characterization
In the synthesis of 3D-G–Fe3O4, GO was reduced to G with the Fe2+ ions. And, the Fe2+ was oxidized to Fe3+ by the oxygen-containing functional groups on the GO nanosheets. Then, the Fe3+ and Fe2+ ions co-precipitated into Fe3O4 nanoparticles, which were decorated onto the thin G sheets. Simultaneously, the G decorated with Fe3O4 was self-assembled into the 3D structure with interconnected networks. The reason for this can be ascribed to the partial overlapping or coalescence of the flexible reduced GO nanosheets via non-covalent interactions, such as π–π stacking, hydrogen bonding, and hydrophobic interactions.20,22 The prepared 3D-G–Fe3O4 was characterized using Fourier transform infrared (FT-IR) spectroscopy, scanning electron microscope (SEM), and transmission electron microscope (TEM), vibrating sample magnetometry (VSM), X-ray diffraction (XRD), Raman spectroscopy, Brunauer–Emmett–Teller (BET) and Barrett–Joyner–Halenda (BJH) techniques. Fig. 1a shows the FT-IR spectra of GO and 3D-G–Fe3O4. In the FT-IR spectrum of GO, the peak at 1734 cm−1 corresponds to the stretching band of –C
O in carboxylic acid or carbonyl moieties. The broad peak at 3440 cm−1 can be attributed to stretching of the phenolic –O–H groups. The peak at 1630 cm−1 (aromatic –C
C) could be assigned to the skeletal vibrations of unoxidized graphitic domains. The band at about 1300 cm−1 corresponded to the bending absorption of carboxyl group –O–C
O, while the deformation of the –C–O was observed at 1034 cm−1. In comparison with the FT-IR spectrum of GO, most of the oxygen functional groups of GO were removed in 3D-G–Fe3O4 (curve b), which illustrated that GO was almost totally reduced in the synthesis process. The presence of a strong peak at 574 cm−1 in the FT-IR spectrum of 3D-G–Fe3O4 is the characteristic vibration of Fe–O bond.
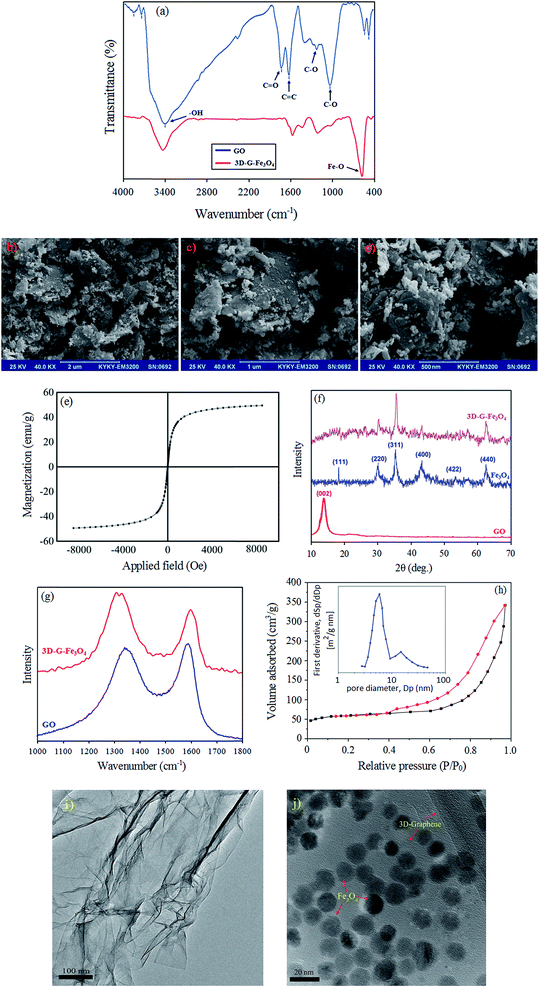 |
| Fig. 1 Characterization of 3D-G–Fe3O4 nanocomposite: (a) FT-IR spectrum of GO (upper curve) and 3D-G–Fe3O4 (lower curve), (b–d) low, middle and high-magnified SEM images of 3D-G–Fe3O4, a homogeneous pore structure of the sample can be clearly observed from SEM images, (e) VSM magnetization curve used to measure the magnetic properties of 3D-G–Fe3O4, (f) XRD pattern of the GO, Fe3O4 and 3D-G–Fe3O4; (g) the Raman spectra of GO and 3D-G–Fe3O4; and (h) nitrogen adsorption–desorption isotherms of 3D-G–Fe3O4, the inset shows BJH pore-size distributions. Adsorptions isotherms are shown by black line with black dots and desorption isotherms are shown by red lines with red dots, (i and j) transmission electron microscope (TEM) images of GO and 3D-G–Fe3O4 nanoparticles, respectively; the dark spots (j) are the Fe3O4 ions intercalated between the 3D-graphene sheets. | |
The morphology of 3D-G–Fe3O4 was investigated by SEM. As can be seen from the SEM images of 3D-G–Fe3O4 (Fig. 1b to d), the reduced GO sheets were well-assembled and interconnected to build a 3D network. In addition, the image also showed that the Fe3O4 nanoparticles were uniformly anchored onto the graphene layers.
Vibrating sample magnetometer (VSM) system at room temperature by cycling the field was used to measure the magnetic properties of 3D-G–Fe3O4. As shown in Fig. 1e, a high saturation magnetization amount of 54.8 emu g−1 with no hysteresis, coercivity and remanence was observed for the adsorbent. Thus, in accordance with the above results, it was reasonable to conclude that our hydrogels were formed by co-assembly of graphene sheets and the synthesis of adsorbent was correct and complete.
The X-ray diffraction (XRD) patterns of the GO, Fe3O4 and 3D-G–Fe3O4 samples are displayed in Fig. 1f. The XRD pattern of GO shows a sharp peak at 2θ = 12.26° corresponding to the (0 0 2) reflection of GO. The characteristic diffraction peaks at 18° (111), 30° (220), 36° (311), 43° (400), 54° (422) and 64° (440) are consistent with the standard XRD data of Fe3O4 nano-particles according to the standard spectrum of Fe3O4 (JCPDS, no. 65-3107). The upper curve of Fig. 1f shows the final XRD pattern of adsorbent. As can be seen, the sharp peak of GO has been removed and Fe3O4 nano-particles are observed. These patterns indicate complete synthesis of 3D-G–Fe3O4.
The change in structure after the self-assembly and reduction process, including disordered and defect structures, is further reflected in the Raman spectra of GO and 3D-G–Fe3O4 (Fig. 1g). There are two peaks at approximately 1340 and 1590 cm−1 in GO spectra corresponding to the D and G band, respectively. The D band originates from the disorder induced mode associated with structural defects and imperfections, while the G band is characteristic of the sp2-hybridized carbon–carbon bonds. The intensity ratio of ID/IG is often used as a measure of disordering. The intensity ratio (ID/IG) of D and G bands is 0.95 in GO and increases to 1.28 in 3D-G–Fe3O4. The increased ID/IG value demonstrates the reduction and magnetization altered the structure of GO and introduced a large number of structural defects.
The specific surface area and pore size distribution of 3D-G–Fe3O4 was obtained using Brunauer–Emmett–Teller (BET) and Barrett–Joyner–Halenda (BJH) techniques, respectively (Fig. 1h). The nitrogen adsorption–desorption isotherm display a hysteresis loop in the relative pressure (p/p0) range of 0.45–0.95 that is used to characterize the porous structure. The sharp increase in adsorption near the relative saturation pressure and the sharp decrease in desorption at certain relative saturation pressure is the typical adsorption–desorption characteristic of a uniform pore system with parallel wall slit-like pore structure. The BET analysis showed a total specific surface area of 218 m2 g−1 for pure 3D-G–Fe3O4. The BJH technique for calculation of pore size indicated that a narrow distribution of pores for 3D-G–Fe3O4 was nearly 5.9 nm (Fig. 1h).
In order to further discussion about the morphology of GO and 3D-G–Fe3O4 nanoparticles, transmission electron microscope (TEM) was performed (Fig. 1i and j). Fig. 1i shows the TEM image for aqueous dispersion of GO. Although, GO nanosheets tend to assembled with each other and forms multilayer agglomerate, the presence of topological features along with overlapping area of graphene oxide nanosheets reveals that they are highly dispersed in water. Bends and wrinkles on GO nanosheets are originated by various defects and functional groups. Thus, the structure of multilayer GO is easily distinguishable in this figure. Furthermore, as can be seen in the TEM image of 3D-G–Fe3O4 (Fig. 1j), all of the rod-like Fe3O4 nanoparticles with a size of about 20 nm were uniformly decorated onto the thin graphene sheets and no free nanoparticles from the sheet supports can be found. Moreover, it should be noted that the nanoparticles are still firmly anchored on the surface of 3D-graphene sheets even after a long time of ultrasonic treatment during the sample preparation for TEM analysis. These results show that the Fe3O4 nanoparticles were uniformly anchored onto the graphene layers and no obvious aggregation or free nanoparticles were detected.
Optimization
The optimum separation condition was achieved by the optimization of some parameters which could affect the extraction recovery. The investigated effective parameters in this study include desorption condition, pH of sample solution, adsorbent dosage, extraction time, and salt concentration. The extraction recovery (ER) of carvedilol was considered as response to evaluate the method performance. The enrichment factor (EF) and ER were calculated using the following equations: |
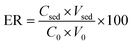 | (1) |
|
 | (2) |
where Csed, C0, Vsed and V0 are final concentration of analyte in organic phase (desorption solvent), initial concentration of analyte in sample solution, volume of desorption solvent, and volume of initial sample solution, respectively. The absorbance measurements were performed using a UV-Vis spectrophotometer. For this purpose, different concentrations of carvedilol were used for construction of calibration graph. As can be shown in Fig. 2, the spectrum shows a well-defined peak, with λmax of 242 nm, in the measuring wavelength region (MeOH as solvent). This wavelength was used for the quantitative determination of carvedilol.
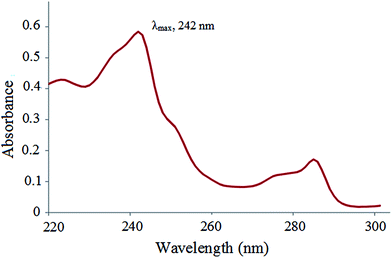 |
| Fig. 2 UV-Vis spectrum of carvedilol (λmax for carvedilol: 242 nm, concentration of analyte: 4 μg mL−1 in MeOH). | |
Influence of desorption conditions
One of the basic steps in obtaining efficient extraction is selecting the type of desorption (extraction) solvent. The extraction solvent was chosen based on capability to dissolve carvedilol and compatibility with HPLC conditions. An appropriate desorption solvent could elute the maximum amount of the adsorbed analyte without destruction of the adsorbent. Therefore, several solvents such as EtOH, MeOH, ACN, DMSO and DMF were tested according to proposed method to choose a suitable desorption solvent. The results indicated that the maximum extraction recovery was achieved by using MeOH as desorption solvent (Fig. 3). This could be explained by the fact that carvedilol has the highest solubility in MeOH (33.8 mg mL−1), due to the stronger hydrogen bonding between MeOH and carvedilol compared with that of other solvents. Moreover, MeOH is a protic solvent that can disrupt hydrogen bonding between analyte and the adsorbent.
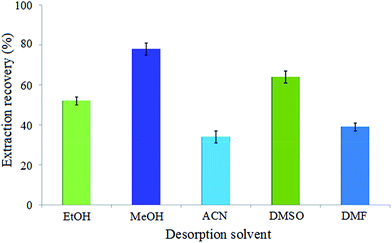 |
| Fig. 3 Effect of desorption solvent type. Extraction conditions: concentration of carvedilol (500 ng mL−1); pH of the sample solution, 6 (buffer solution of KH2PO4/NaOH); dosage of adsorbent, 20 mg; extraction time, 5 min and salt concentration 5 (w/v%). | |
The volume of desorption solvent according to eqn (1) and (2) has a significant role in enrichment factor and extraction recovery. Moreover, desorption solvent volume should be enough to elute completely the adsorbed analyte. The effect of this parameter was investigated with different volumes of MeOH from 1 to 5 mL. The results in Fig. 4a show that the highest extraction efficiency was obtained with 2 mL of desorption solvent. The lower recoveries in 2–4 mL is due to insufficient MeOH for desorption of all carvedilol in the solution. And, the reduced recoveries at the volumes higher than 4 mL could be ascribed to the dilution effect. Therefore, 2 mL of MeOH was selected as the volume of desorption solvent and used in next steps.
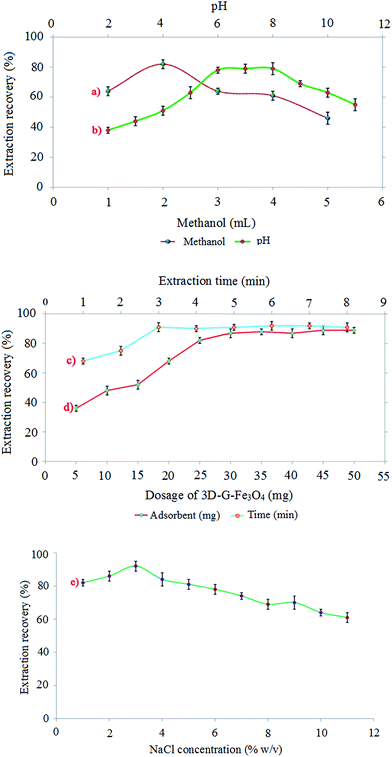 |
| Fig. 4 (a) Effect of desorption solvent volume. Extraction conditions: concentration of carvedilol (200 ng mL−1); pH of the sample solution, 6; dosage of adsorbent, 20 mg; extraction time, 5 min and salt concentration 5 (w/v%). (b) Effect of pH, extraction conditions: concentration of carvedilol (200 ng mL−1); desorption solvent volume, 2 mL; dosage of adsorbent, 20 mg; extraction time, 5 min and salt concentration 5 (w/v%). (c) Effect of adsorbent dosage, extraction conditions: concentration of carvedilol (200 ng mL−1); desorption solvent volume, 2 mL; pH of the sample solution, 7; extraction time, 5 min and salt concentration 5 (w/v%). (d) Effect of extraction time, extraction conditions: concentration of carvedilol (200 ng mL−1); desorption solvent volume, 2 mL; pH of the sample solution, 7; dosage of adsorbent, 30 mg; and salt concentration 5 (w/v%). (e) Effect of salt concentration, extraction conditions: concentration of carvedilol (200 ng mL−1); desorption solvent volume, 2 mL; pH of the sample solution, 7; dosage of adsorbent, 30 mg; extraction time, 3 min. | |
In addition, the effect of desorption time was also investigated from 1 to 8 min under sonication by sonication of the adsorbent in MeOH. The results indicated that desorption time had no obvious effect on the extraction efficiency, because the desorption process was quick and efficient. This is attributed to the high solubility of carvedilol in MeOH. However, for the adsorption process, the mass transfer of the analyte from water samples onto the adsorbent was much slower. Thus, desorption time was selected as 1 min for sonication of adsorbent in MeOH at 25 °C.
Effect of pH
The pH of sample solution plays a critical role in the adsorption process and thus extraction efficiency of the target analyte. Since, the pH affects the existing forms of the analytes and the surface charge type and density of the adsorbent.23 The point zero charge (PZC) of graphene-based material occurs in pH of 6–7.24 Therefore, at pH < pHPZC, the adsorbent surface is positively charged, whereas at pH > pHPZC, the adsorbent surface is negatively charged. In addition, the solubility and dissolution of carvedilol are highly dependent on pH. In carvedilol, the NH group of carbazole is acidic of pKa of 15.0 because the lone pair of electrons is involved in aromaticity. Thus, the N–H bond of carbazole is weak and hydrogen is easily hydrolyzed as H+. Contrarily, the aliphatic secondary amine is weakly basic of pKa = 7.8 due to the existence of electronegative surrounding oxygen atoms and the bulkiness of attached alkyl groups carrying aromatic rings on two sides. Moreover, the amphoteric property (having both acidic and basic moieties) of carvedilol explains its ionization pattern. In acidic pH medium, the aliphatic NH is ionized to form a cationic center, whereas in basic pH medium, the carbazole NH is ionized to produce an anionic center. Therefore, the zwitterion characteristic of carvedilol demonstrates its solubility manner.25 Although, the adsorption of carvedilol onto 3D-G–Fe3O4 surface is mainly governed by π–π stacking and hydrogen bonding, but the electrostatic interactions between the adsorbent and analyte partially influences the adsorption process. Therefore, the effect of pH on the extraction recovery of carvedilol was investigated in the range of 2 to 11 by adjusting pH of the sample solution with HCl or NaOH. As can be seen from Fig. 4b, the extraction recovery increased with a mild slope by increasing the pH in the range of 2 to 6 and approximately fixed in the region of 6 to 8, then decreased rapidly when pH was further increased. Regarding the normal pH of plasma which is about 7, and based on the above explanations neutral pH (pH = 7) was considered as the optimum pH value for further experiments.
Effect of adsorbent dosage
In order to achieve the highest extraction recovery, the effect of 3D-G–Fe3O4 amount was studied in the range from 5 to 50 mg (n = 3) with the standard solution of carvedilol (200 ng mL−1) of according to the extraction procedure. The results in Fig. 4c show that, the extraction recovery for carvedilol increased rapidly when the adsorbent amount was increased from 5 to 30 mg and then remained almost constant. This may be due to increasing the accessible surface area of 3D-G–Fe3O4 that increases the adsorption capacity. Since 30 mg of the adsorbent is enough for the quantitative adsorption of carvedilol, therefore higher amounts of the adsorbent have no effect on the efficiency of the method. Therefore, 30 mg was selected as optimum amount of adsorbent for extraction of 200 ng mL−1 carvedilol from 40 mL of sample solution in the subsequent studies.
Effect of extraction time
In the MSPE process, extraction time is one of the main parameters that influence the extraction efficiency of the analyte. Extraction time is the time duration that the adsorbent is in contact with the sample solution to complete the extraction process. In order to find a suitable extraction time, several experiments were carried out using 40 mL of carvedilol solutions (200 ng mL−1) at pH 7 and 40 mg adsorbent in a series of sonication time (1–8 min). The results displayed in Fig. 4d showed that the extraction efficiency was increased to a maximum in 3 min and then remained almost constant. This is the time required to achieve equilibrium conditions between the sample solution and the adsorbent. Based on the above experimental results, 3 min was selected as the optimum extraction time (Fig. 4d).
Effect of salt concentration
To evaluate the effect of ionic strength on the extraction recovery of carvedilol, different amounts of NaCl in the concentration range of 0–10 (% w/v) was added to the sample solution. The experiments were performed with a 200 ng mL−1 solution of carvedilol in accordance with the proposed procedure. Based on the results shown in Fig. 4e, it is evident that when salt content was increased from 0 to 2% w/v, extraction recovery was increased and then decreased with further increase of the salt. The increasing of ionic strength by the addition of salt up to 2% w/v can improve the extraction recovery by decreasing the solubility of carvedilol in the aqueous phase due to the salting-out effect. When salt content was increased from 2 to 10% w/v, the decrease of extraction recovery can be ascribed to occupying active sites on the adsorbent surface by the salt ions. Therefore, the diffusion rate of carvedilol onto the adsorbent surface was decreased with increasing salt concentration. According to the above results, 2.0% w/v was selected as the optimum amount of NaCl concentration.
Reusability of the adsorbent
Reusability is an important parameter in evaluating practical application of the adsorbent. Because of its high surface area, three-dimensional nanoporous structure and excellent magnetic properties, the adsorbent (3D-G–Fe3O4) is a superior MSPE adsorbent for different extraction–desorption cycles. In order to examine this property of the adsorbent, after extraction completed, it was washed twice sequentially with 2 mL of MeOH and 2 mL of deionized water. Then, it was dried and reused for next experiments according to the proposed procedure. The results showed that the adsorbent can be reused at least 15 times with the extraction recoveries higher than 90%.
Validation of the method
The validation of the proposed method was carried out by analyzing of carvedilol standard sample (200 ng mL−1) under the optimized experimental conditions (pH of 7, 3 min for extraction time, 30 mg of adsorbent, 2% (w/v) of NaCl, and 2 mL of MeOH as desorption solvent). The calibration curve was prepared using 10 concentration levels of carvedilol standard solutions in the range of 2–1000 ng mL−1 with the proposed procedure and constructed by plotting peak areas against the respective concentrations. The least-squares regression technique was used to evaluate the linearity of the method in calibration curve range and R2-value (determination coefficient), y-intercept and slope of the regression line were calculated by this statistical technique. The limit of detection (LOD) value was calculated for carvedilol using the 3Sd/m (where Sd is the standard deviation of the blank and m is the slope of calibration curve) equation. To evaluate the precision of the method, the intra- and inter-day precisions were calculated from the same samples of carvedilol. The intra-day precision was calculated by performing three determinations at the same concentration (200 ng mL−1) in a day under the same experimental conditions, and the inter-day precision was evaluated by comparing the assays on three different successive days. The relative standard deviations (RSDs%) of the intra- and inter-day precision were less than 2.70 and 3.85%, respectively. The results given in Table 1 indicated that the proposed method has a good precision and high sensitivity for carvedilol measuring.
Table 1 Summary of validation data of proposed method for carvedilol analysis
Statistical parameter |
Measured value |
Standard deviation. Determination of coefficient. Linear dynamic range. Limit of detection. |
Slope ± SDa |
0.1381 ± 0.0021 |
Intercept ± SD |
0.0273 ± 0.0008 |
R2b |
0.994 |
LDRc (ng mL−1) |
2–1000 |
LODd (ng mL−1) |
0.5 |
Inter day RSD (n = 5) |
3.85 |
Intraday RSD (n = 5) |
2.70 |
To investigate the effect of sample matrices on extraction efficiency, the relative recovery (RR)26 was calculated based on following equation (eqn (3)):
|
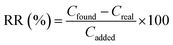 | (3) |
where
Cfound is concentration of analyte in the final solution of spiked sample which is calculated from the calibration curve,
Creal is the concentration of analyte in the final solution of non-spiked sample and
Cadded is the concentration of standard solution that was spiked into the real sample. Therefore, in order to evaluate performance of the method, it was applied to detect the carvedilol amount in human blood plasma samples containing 100, 200 and 500 ng mL
−1 of carvedilol. All the experiments were performed under the optimal conditions. The results summarized in
Table 2 indicated that carvedilol was not detected in the drug-free human blood plasma samples, and the recoveries for the spiked samples were in the range of 96 to 105% (RSD < 5%). Accordingly, human blood plasma matrices have no significant effect on extraction efficiency and this method can be used for determination of carvedilol in complex matrix samples.
Fig. 5 shows the overlaid HPLC-UV chromatograms of non-spiked and spiked human plasma samples with carvedilol. As can be seen, in the chromatogram of the spiked sample only the sharp and symmetric peak of carvedilol at 5.56 min is observed, without any peak due to impurities. However, in the chromatogram of the non-spiked sample no peak was appeared. Therefore, these two chromatograms confirm that in both cases there is no serious interference from macromolecules and other components of blood plasma matrix. This confirms the selectivity of the method in blood plasma sample. Based on these results, we can conclude that the proposed method show a good selectivity for extraction and determination of carvedilol in complex matrices.
Table 2 Determination of four levels of carvedilol concentration in human plasma samples
Sample |
Spiked (ng mL−1) |
Found (ng mL−1) |
RRa (%) |
RSDb (%) |
Relative recovery: percentage of (concentration of founded analyte spiking of standard − concentration of founded analyte without spiking of standard)/(concentration of spiked standard). Relative standard deviation (n = 5). Not detected. |
Human plasma |
0 |
n. d.c |
— |
— |
100 |
105 |
105 |
5 |
200 |
194 |
97 |
3 |
500 |
471 |
96 |
4 |
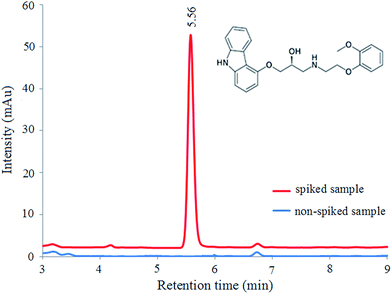 |
| Fig. 5 The overlaid HPLC-UV chromatograms of non-spiked and spiked carvedilol in human plasma samples (Rt: 5.56 min). Extraction conditions: desorption solvent volume, 2 mL; pH of the sample solution, 7; dosage of adsorbent, 30 mg; extraction time, 3 min; salt concentration 2% (w/v); concentration of carvedilol, upper chromatogram: 200 ng mL−1 and lower chromatogram: plasma sample without carvedilol. | |
The performance of the proposed MSPE method was compared with the other reported methods.2,7,27–31 As shown in Table 3, the LOD of the presented work is better than flow injection (FI), dispersive liquid–liquid microextraction (DLLME), and liquid–liquid extraction methods (LLE), and is comparable with stir bar sorptive extraction (SBSE). Moreover, the linear dynamic range of the presented method is considerably wider than other methods. This can be due to the porous structure of the adsorbent and its high adsorption capacity. The RSD parameter indicates the quality of the MSPE method for accurate extraction and determination of carvedilol. The precision of the proposed method based on RSD is better than that of the others. Furthermore, the presented work had short extraction time in comparison with other reported methods, which can be due to the high specific surface area of 3D-G–Fe3O4. These results indicate that MSPE method is accurate, fast and sensitive for extraction and determination of carvedilol in human blood plasma matrix.
Table 3 Comparison of the proposed method with other previously reported methods for the determination of carvedilol
Method |
Matrices |
Analysis |
LODa |
LDRb |
RSDc |
Extraction time (min) |
Ref. |
Limit of detection (ng mL−1). Linear dynamic range (ng mL−1). Relative standard deviations (%). Liquid–liquid extraction. High performance liquid chromatography with tandem mass spectroscopy. Without data. Flow injection. High performance liquid chromatography with ultraviolet spectroscopy. Dispersive liquid–liquid microextraction. Stir bar sorptive extraction. Magnetic solid phase extraction. |
LLEd |
Human plasma |
HPLC-MS/MSe |
WDf |
0.1–200 |
<20.00 |
3.5 |
27 |
FIg |
Human plasma and blood |
HPLC-UVh |
WD |
0.5–25 |
<5.90 |
25 |
28 |
LLE |
Dog plasma |
HPLC-MS/MS |
WD |
1–100 |
<5.00 |
5 |
29 |
FI |
Medicine dosage |
Synchronous fluorimetry |
1 |
0.5–100 |
2.47 |
WD |
30 |
DLLMEi |
Urine and plasma |
HPLC-UV |
14 |
50–750 |
<15.00 |
5 |
7 |
SBSEj |
Human serum |
HPLC-UV |
0.3 |
1–120 |
<6.00 |
50 |
2 |
LLE |
Human serum |
HPLC-UV |
2.5 |
5–500 |
<20.00 |
5 |
31 |
MSPEk |
Human plasma |
HPLC-UV |
0.5 |
2–2000 |
3.85 |
3 |
This work |
Conclusions
In this research, 3D-graphene magnetic nanocomposite was synthesized and successfully applied as an efficient adsorbent for the extraction and determination of carvedilol in human blood plasma sample. The dispersion of adsorbent in sample solution resulted in an increased mass transfer from the solution onto the adsorbent, and thus shortened the equilibration time. In addition, magnetic property of the adsorbent greatly facilitated the phase separations, and thus some limitation of solid phase extraction such as time-consuming column passing or filtration operations were avoided. The high porous structure of 3D-graphene provided a large surface area per unit volume that speeds up the adsorption process. In addition, the high porosity of the adsorbent surface creates the conditions that diffusion of macromolecules is hindered, and therefore enhances selectivity of adsorbent toward the analyte in complex matrices such as blood serum. According to the results obtained in this study, 3D-G–Fe3O4 provide advantages such as a simple and eco-friendly procedure, high extraction efficiency, chemical stability, good reusability, short analysis time, consumption of low volumes, and high capacity for carvedilol extraction.
References
- B. Dulin and W. T. Abraham, Am. J. Cardiol., 2004, 93, 3–6 CrossRef PubMed.
- Z. Talebpour, M. Taraji and N. Adib, J. Chromatogr. A, 2012, 1236, 1–6 CrossRef CAS PubMed.
- P. Biparva, S. M. Abedirad and S. Y. Kazemi, Talanta, 2014, 130, 116–121 CrossRef CAS PubMed.
- R. Rathod, L. P. Prasad, S. Rani, M. Nivsarkar and H. Padh, J. Chromatogr. B: Anal. Technol. Biomed. Life Sci., 2007, 857, 219–223 CrossRef CAS PubMed.
- M. Machida, M. Watanabe, S. Takechi, S. Kakinoki and A. Nomura, J. Chromatogr. B: Anal. Technol. Biomed. Life Sci., 2003, 798, 187–191 CrossRef CAS.
- C. Theivarasu, S. Ghosh and T. Indumathi, Asian J. Pharm. Clin. Res., 2010, 3, 64–68 CAS.
- A. R. S. Soltani, N. Soltani and A. Jouyban, Bioanalysis, 2012, 4, 2805–2821 CrossRef PubMed.
- S. L. F. Behn and H. Scholz, J. Chromatogr. Sci., 2001, 39, 121–124 Search PubMed.
- T. P. Rao, P. Metilda and J. M. Gladis, Talanta, 2006, 68, 1047–1064 CrossRef CAS PubMed.
- C. D. D. Huang and X. Zhang, Anal. Methods, 2014, 6, 7130–7141 RSC.
- G. Cheng, M. He, H. Peng and B. Hu, Talanta, 2012, 88, 507–515 CrossRef CAS PubMed.
- J. Dong, Z. Xu and F. Wang, Appl. Surf. Sci., 2008, 254, 3522–3530 CrossRef CAS.
- C. Y. Hong and Y.-C. Chen, J. Chromatogr. A, 2007, 1159, 250–255 CrossRef CAS PubMed.
- H. Abdolmohammad-Zadeh and Z. Talleb, Microchim. Acta, 2012, 179, 25–32 CrossRef CAS.
- J. Sun, Q. Liang, Q. Han, X. Zhang and M. Ding, Talanta, 2015, 132, 557–563 CrossRef CAS PubMed.
- F. Liu, S. Chung, G. Oh and T. S. Seo, ACS Appl. Mater. Interfaces, 2012, 4, 922–927 CAS.
- S. H. Choi, J.-K. Lee and Y. C. Kang, Nano Res., 2015, 8, 1584–1594 CrossRef CAS.
- S. Zhang, Z. Li, X. Yang, C. Wang and Z. Wang, RSC Adv., 2015, 5, 54329–54337 RSC.
- W. Hummers, J. Am. Chem. Soc., 1958, 80, 1339–1340 CrossRef CAS.
- H.-P. Cong, X.-C. Ren, P. Wang and S.-H. Yu, ACS Nano, 2012, 6, 2693–2703 CrossRef CAS PubMed.
- L. Liu, T. Feng, C. Wang, Q. Wu and Z. Wang, Microchim. Acta, 2014, 181, 1249–1255 CrossRef CAS.
- X. Liu, X. Zhou, C. Wang, Q. Wu and Z. Wang, Food Addit. Contam., Part A, 2015, 32, 40–47 CrossRef CAS PubMed.
- G. Zhao, S. Song, C. Wang, Q. Wu and Z. Wang, Anal. Chim. Acta, 2011, 708, 155–159 CrossRef CAS PubMed.
- H. Rashidi Nodeh, W. A. Wan Ibrahim, M. A. Kamboh and M. M. Sanagi, RSC Adv., 2015, 5, 76424–76434 RSC.
- R. Hamed, A. Awadallah, S. Sunoqrot, O. Tarawneh, S. Nazzal, T. AlBaraghthi, J. Al Sayyad and A. Abbas, AAPS PharmSciTech, 2016, 17, 418–426 CrossRef CAS PubMed.
- Q. Ye, Z. Chen, L. Liu and L. Hong, Anal. Methods, 2016, 8, 3391–3396 RSC.
- N. C. do Carmo Borges, G. Duarte Mendes, D. de Oliveira Silva, V. Marcondes Rezende, R. E. Barrientos-Astigarraga and G. De Nucci, J. Chromatogr. B: Anal. Technol. Biomed. Life Sci., 2005, 822, 253–262 CrossRef CAS PubMed.
- M. Saito, J. Kawana, T. Ohno, M. Kaneko, K. Mihara, K. Hanada, R. Sugita, N. Okada, S. Oosato, M. Nagayama, T. Sumiyoshi and H. Ogata, J. Chromatogr. B: Anal. Technol. Biomed. Life Sci., 2006, 843, 73–77 CrossRef CAS PubMed.
- M. P. McIntosh, B. J. Carlson, K. S. Schorno and R. A. Rajewski, J. Chromatogr. B: Anal. Technol. Biomed. Life Sci., 2007, 852, 665–668 CrossRef CAS PubMed.
- Y. Xiao, H. Y. Wang and J. Han, Spectrochim. Acta, Part A, 2005, 61, 567–573 CrossRef PubMed.
- R. Gannu, V. V. Yamsani and Y. M. Rao, J. Liq. Chromatogr. Relat. Technol., 2007, 30, 1677–1685 CrossRef CAS.
|
This journal is © The Royal Society of Chemistry 2016 |