DOI:
10.1039/C6RA10620J
(Paper)
RSC Adv., 2016,
6, 67340-67352
Gelatin-based dissolvable antibacterial films reinforced with metallic nanoparticles
Received
25th April 2016
, Accepted 9th July 2016
First published on 11th July 2016
Abstract
Six different types of metallic and bimetallic nanoparticles (Au, Ag, Cu, AuAg, AuCu, and AgCu) were prepared using an aqueous extract of Artemisia scoparia (AS) as reducing and stabilizing agents and gelatin/metallic nanoparticle composite films were prepared. The nanoparticles (NPs) were characterized by UV-visible spectroscopy, TEM, XRD, zeta potential, and XPS. Silver and its bimetallic nanoparticles showed strong antimicrobial activity against both Gram-positive and Gram-negative bacteria. Apparent surface color and light transmittance of gelatin film was greatly influenced by the reinforcement of the NPs. However, mechanical properties were not changed significantly (p > 0.05) when blended with the NPs. All of the gelatin/NPs composite films exhibited strong UV light screening function. The gelatin-based composite films with AgNPs, CuNPs, and their alloy showed strong antibacterial activity against both Gram-positive and Gram-negative pathogenic bacteria. Gelatin films incorporated with AuNPs, AgNPs, and AuAgNPs exhibited strong antioxidant activity. The multifunctional gelatin-based metallic nanocomposite films have a high potential for applications as biocompatible functional hydrogel films.
Introduction
Acne is a serious skin problem which annoys more than 85% of adolescents aged between 11 and 30 years in the world by augmenting facial grease production and inflammation of papules or pustules with conspicuous skin blemishes.1 Pathogenesis of acne lesions is initiated by infectious colonization of bacteria such as Propionibacterium acne, Staphylococcus epidermidis, Escherichia coli, and Staphylococcus aureus, followed by lipolytic enzyme production, sebum excretion, comedogenesis, sebaceous hyperplasia, follicular hyperkeratinization, hormone imbalance, and immune hypersensitivity.2,3 In addition, free radicals generated by environmental factors such as toxins and UV rays of sunlight can attack and damage the vital components of healthy skin cells that aggravates acne lesion. Topical (acne patch, retinoids, antibiotics, benzoyl peroxide), photodynamic (levulan) and oral therapies (isotretinoin, antibiotics) are usually used to cure this chronic disease.4,5 However, the prolonged use of antibiotics would induce antibiotic resistant bacterial strains.6 Recently, isotretinoin has been claimed to be the most effective oral therapy and has been used for the treatment of severe acne trouble. However, its use is limited due to the teratogenicity and other side effects.1 In addition to the serious side effect of such oral medicine, a high cost of photodynamic therapy has limited their use pervasively. Instead, a topical therapy has been used for the treatment of moderate acne since it is considered to be safer than above mentioned therapies. Moreover, the versatility of topical formulation such as gel, cream, ointment, patch, hydrogel, film, and dressing extends its utility window in spite of their limitations such as less loading of anti-acne drugs (gel), irritation with repeated applications (cream, ointment), heat sensitivity and poor percutaneous penetration (patch). One of the problems of current topical formulation is that water based formulations are not available, which makes to rely on oil based vehicles for drug transportation that augments more oil to the skin. Not only to overcome such problems, but also to replace the harmful chemicals, there is a growing interest for the development of skin-care products using mild and natural alternatives with tailored properties. Recently, eco-friendly antimicrobial hydrocolloid-based bionanocomposite films with non-toxicity, dosage flexibility, proper mechanical strength, enhanced peelability, ease of use, and UV light screening property have been emerged as an effective alternative for the topical therapy.7
As one of such hydrocolloid materials, gelatin is interesting since it is totally biodegradable, biocompatible, and safe to use with good film forming properties.8 In order to improve the properties such as functional, mechanical, antimicrobial, transparency, and UV light barrier properties of the gelatin films, metallic or metallic oxide nanoparticles (gold, silver, zinc, and copper) have been used as reinforcing fillers in the polymer matrix.9 For the preparation of metallic nanoparticles, a green method using biological materials has been preferred to the chemical or physical methods since they exhibited higher antimicrobial and antioxidant activity with less side effects for the biomedical application.9 Regarding this, halophytes, an edible flora of saline habitat, have a high potential for the use of biological synthesis of metallic nanoparticles. Halophytes have gathered enormous attention due to their variety of bioactive compounds with beneficial effects on various skin disorders such as itching and inflammations, wounds and burns, skin eruptions, as well as boils and acne.10–13 As one of halophytes, Artemisia scoparia (AS) is promising for the biological synthesis of metallic nanoparticles. The AS, which belongs to the family Asteraceae and commonly called redstem wormwood, is known to have various medicinal functions such as anti-oxidative, antiseptic, antimicrobial, antibacterial, antifungal, antipyretic, anti-allergic, and anti-inflammatory functions.14,15 However, the efficacy of AS-mediated biological synthesis of metallic nanoparticles has not yet been investigated.
Therefore, the objectives of the present study were preparation of metallic nanoparticles such as AuNPs, AgNPs, CuNPs, AuAgNPs, AuCuNPs, and AgCuNPs using AS as a reducing and stabilizing agent, and preparation of gelatin-based functional bionanocomposite films.
Materials and methods
Materials
All chemicals were procured from Sigma-Aldrich (St. Louis, MO, USA) unless otherwise stated. Gelatin was procured from Gel-Tec Co., Ltd. (Seoul, Korea). Tryptic soy broth (TSB), brain heart infusion broth (BHI), and agar powder were purchased from Duksan Pure Chemicals Co., Ltd (Ansan, Gyeonggi-do, Korea). The pathogenic test microorganisms such as Escherichia coli ATCC 25922 and Staphylococcus epidermis KCTC 1917 were obtained from Korean Collection for Type Cultures (KCTC, Seoul, Korea). All strains were grown in TSB and BHI agar plates and stored at 4 °C for further test.
Preparation of Artemisia scoparia extract
Artemisia scoparia (AS) was collected from the west coast of Jeonnam province of South Korea in May, 2014. The aerial part of the plant was steamed and dried at 45 °C for 2 days, then ground using a mortar and stored at −70 °C still used. The dried powder of AS (4 kg) were extracted with distilled water (40 L) at 121 °C for 30 min and filtered through no. 2 filter paper (Whatman, Maidstone, UK) to obtain AS extract.
Preparation of metallic nanoparticles
The gold, silver, copper, and bimetallic nanoparticles were synthesized by the method of Shankar et al. with slight modification.16 10 mL of AS extract was added to 490 mL of deionized water with nitrogen gas flush and heated to 70 °C with continuous stirring using a magnetic stirrer. For the synthesis of gold (AuNPs), silver (AgNPs), and copper (CuNPs) nanoparticles, 0.5 mL of 1 M aqueous solution of gold(III) chloride trihydrate (HAuCl4), silver nitrate (AgNO3), and copper(II) nitrate trihydrate (CuN2O3), respectively, was added dropwise to the AS solution and heated till the color of the solutions changed. For the synthesis of bimetallic nanoparticles, 0.25 mL (1 M) of each metal salt solution (HAuCl4 and AgNO3; HAuCl4 and CuN2O3; AgNO3 and CuN2O3) was added dropwise as described above. The nanoparticles formed were designated as AuNPs (gold), AgNPs (silver), CuNPs (copper), AuAgNPs (gold–silver), AuCuNPs (gold–copper), and AgCuNPs (silver–copper) nanoparticles, respectively.
Characterization of nanoparticles
The formation of NPs was monitored by observing the color change and light absorption behavior of the solution using a UV-visible spectrophotometer (Mecasys Optizen POP Series UV/Vis, Seoul, Korea) in the wavelength of 200–700 nm. Shape and size of the NPs were determined by transmission electron microscopy (TEM). The image analysis of NPs was done using JEOL-1010 instrument operated at an accelerating voltage of 120 kV. Fourier transform infrared (FT-IR) spectra of the NPs were obtained in the wavenumber of 4000–600 cm−1 using an attenuated total reflectance-Fourier transform infrared (AT-FTIR) spectrophotometer (TENSOR 37 spectrophotometer with OPUS 6.0 software, Billerica, MA, USA) operated at a resolution of 4 cm−1. The crystalline nature of NPs was determined with X-ray diffraction (PANalytical Xpert pro MRD diffractometer, Amsterdam, Netherlands) by placing NPs powder on a glass slide and spectra were recorded using Cu-Kα radiation at 40 kV and 30 mA with scanning at 2θ = 30–80°. X-ray photoelectron spectroscopy (XPS) measurements were carried out with a Thermo K-Alpha XPS instrument (Thermo Scientific, NY, USA) at a pressure of ∼1 × 10−9 Torr (1 Torr = 1.333 × 102 Pa). The core-level spectra from the respective samples were recorded with monochromated aluminum Kα radiation (photon energy = 1486.6 eV) at a pass energy of 20 eV and an electron takeoff angle (angle between the electron emission direction and surface plane) of 90°. The core level binding energies were aligned with the C1s (adventitious carbon) binding energy of 284.6 eV. The surface charge of NPs was measured by zeta potential and particle size by dynamic light scattering (DLS) method using Zeta PALS-zeta potential analyzer (Brookhaven Instruments Corporation, NY, USA).
Antibacterial activity of nanoparticles
The antimicrobial activity in terms of minimum inhibitory concentration (MIC) and minimum bactericidal concentration (MBC) of NPs against Gram-positive (S. epidermis) and Gram-negative (E. coli) pathogenic bacteria was performed using a modified broth microdilution method recommended by the Clinical Laboratory Standardization Institute (CLSI) guideline.17,18 An aliquots (50 μL) of two fold-serially diluted NPs suspensions were prepared in a 96-well microtiter plate to obtain final concentration ranged from 0.9–1000 μM, followed by the addition of 50 μL of TSB or BHI media. One hundred microliters of the bacteria (∼106 CFU mL−1) was added into each well. After incubation at 37 °C for 15 h, resazurin (Sigma, St. Louis, MO, USA) as a redox indicator was added to each well, and incubated for 3 h. The growth was assessed by a microtitre plate reader by measuring the optical density (OD) at 595 nm. The minimum inhibitory concentration (MIC) was defined as the lowest concentration of the NPs suspension that inhibited the bacterial growth completely. MBC, the lowest concentration of the NPs that are completely preventing bacterial growth, was determined with the NPs that gave MIC by subculturing on fresh TSB and BHI agar plates. All assays were carried out in triplicates.
Preparation of gelatin/nanoparticles composites film
Gelatin and gelatin/NPs composite films were prepared by the solution casting method.17 To make gelatin/NPs composite films, 4 g of gelatin was added slowly into the above prepared NPs solutions (150 mL) until they dissolved completely, then 1.2 g of glycerol was added as a plasticizer and heated at 80 °C for 20 min with constant stirring. The completely solubilized film forming solution was cast evenly on a leveled Teflon film (Cole-Parmer Instrument Co., Chicago, IL, USA) coated glass plate (24 × 30 cm) and dried at room temperature for about 48 h, then peeled off from the glass plates and preconditioned in a constant temperature and humidity chamber (model FX 1077, Jeio Tech Co. Ltd., Ansan, Korea) controlled at 25 °C and 50% RH at least for 48 h before further tests. The control gelatin film was prepared by the same method without the addition of NPs. The gelatin/NPs composite films were designated as gelatin/AuNPs, gelatin/AgNPs, gelatin/CuNPs, gelatin/AuAgNPs, gelatin/AuCuNPs, and gelatin/AgCuNPs, respectively.
Characterization of gelatin/NPs composite films
Morphology and optical properties. For the microstructure analysis of the film samples, small pieces of films were placed on the SEM specimen holder and analyzed using a field emission scanning electron microscopy (FE-SEM, S-4800, Hitachi Co., Ltd., Matsuda, Japan) with an accelerating voltage of 5.0 kV. The surface color of the films was measured using a Chroma meter (Konica Minolta, CR-400, Tokyo, Japan) with a white color plate (L = 97.75, a = −0.49, and b = 1.96) as a standard background for color measurement.18 Hunter color values (L, a, and b) were determined by taking an average of five readings from each film sample. Total color difference (ΔE) was calculated as follows:
ΔE = [(ΔL)2 + (Δa)2 + (Δb)2]0.5 |
where ΔL, Δa, and Δb are the difference between each color value of standard color plate and film samples, respectively. Optical properties of the films were determined by measuring light absorption spectra and the transparency of the films. Each film was cut into a square piece (5 cm × 5 cm) and directly mounted between spectrophotometer magnetic cells, and the absorbance and percent transmittance were measured using a UV-vis spectrophotometer (Mecasys Optizen POP Series UV/Vis, Seoul, Korea). Absorbance was taken in the wavelength of 200–700 nm. Transparency of the films was tested by measuring percent transmittance at 280 nm (T280) and 660 nm (T660).
Tensile properties of the films. The thickness of the film samples was measured by hand-held micrometer (Dial Thickness Gauge 7301, Mitutoyo Corporation, Kanagawa, Japan). The average values of five random measurements were taken for each film thickness. Each film was cut into rectangular strips (2.54 cm × 15 cm) and the tensile strength (TS), elongation at break (E), and elastic modulus (EM) of each film were measured according to the standard test method of ASTM D 882-88 using Instron Universal Testing Machine (Model 5565, Instron Engineering Corporation, Canton, MA, USA). The machine was operated in a tensile mode with an initial grip separation of 50 mm and crosshead speed of 50 mm min−1. The TS was determined by dividing the maximum load (N) by the initial cross-sectional area (m2) of the films and expressed in MPa. The E (%) was determined by dividing the extension at the rupture of the film by the initial length of the film (50 mm) multiplied by 100. The EM (MPa) was determined from the slope of the linear portion of the stress–strain curve, which corresponds to the stress divided by the strain of the film sample. Ten measurements were carried out for each film and the average values were presented.
Moisture content (MC) and water contact angle (WCA). The surface hydrophobicity of the films was determined by measuring the water contact angle (WCA) of the film surface using a WCA analyzer (model Phoenix 150, Surface Electro-Optics Co., Ltd., Kunpo, Korea). Each film was cut into rectangular pieces (3 cm × 10 cm) and placed on the horizontal stage (Black Teflon coated steel, 7 cm × 11 cm). A drop of water (∼10 μL) was placed on the surface of the film and contact angle was measured on both sides of the water drop to assume symmetry and horizontal level.19 The average values of three measurements of each sample were presented as the degree of WCA. For moisture content (MC) analysis, each film was cut into 3 cm × 3 cm and dried at 105 °C for 24 h using a drying oven and expressed as percent MC in wet basis.20
Antimicrobial activity of films
The antibacterial activities of neat gelatin and gelatin/NPs composite films were also examined against S. epidermis, and E. coli using viable colony count method.17,21 S. epidermis, and E. coli were aseptically inoculated to 20 mL of BHI and TSB broth and subsequently incubated at 37 °C for 16 h. Each cultured broth was centrifuged at 5000 rpm for 10 min, and the cell pellets were suspended in 100 mL of sterile TSB and BHI broth, and diluted 10 times with sterile distilled water. 50 mL of diluted broth (106 to 107 CFU mL−1) was taken into 100 mL of the conical flask containing film sample (5 cm × 5 cm) and subsequently incubated at 37 °C for 12 h under mild shaking. The same diluted broth without film sample was used as the control. At every 3 h interval, the cell viability of each pathogen was calculated by counting bacterial colonies on the plates. Antimicrobial tests were performed in triplicate with individually prepared films.
Determination of antioxidant activity
For antioxidant assay, 200 mg of film samples were dissolved into 10 mL of double distilled water to get 20 mg mL−1 film solutions.
DPPH radical scavenging assay. The free radical-scavenging activity of gelatin/NPs nanocomposite films were evaluated following the methods of Brand-Williams et al.22 with a slight modification. Briefly, aqueous solution (100 μL) of each film sample (equivalent to 2 mg sample dry wt) was added into the 900 μL of DPPH (2,2-diphenyl-1-picrylhydrazyl) radical ethanol solution (final concentration, 100 μM). The mixed solution was incubated for 30 min in the dark. The free radical-scavenging activity of each sample was quantified by observing the decolorization of DPPH at 517 nm. The DPPH radical-scavenging activities of samples were also determined as a percentage decrease in optical density compared with the absorbance of a blank sample.
ABTS + radical scavenging assay. ABTS + radical-scavenging activity was determined using the method of Re et al.23 with slight modification. One hundred milliliters each of 2.5 mM ABTS (2,2′-azino-bis(3-ethylbenzothiazoline-6-sulphonic acid)) solution and 1 mM AAPH (2,2′-azobis(2-amidinopropane)dihydrochloride) solution were mixed to form ABTS + radical solution and incubated for 30 min in the dark at 70 °C. The ABTS + radical solution was further diluted with phosphate buffer saline (PBS) to get pH 7.4. Then, 980 μL of diluted ABTS + solution was added to 20 μL of film sample solution (equivalent to 0.4 mg film dry wt). After 30 min of incubation, the absorbance was measured at 734 nm by UV/VIS spectrophotometer (HP-8452A, Hewlett Packard, USA).
Ferric reducing (FRAP) ability assay. The ferric ion reducing ability of the metallic and bimetallic nanocomposite films were measured by a FRAP assay following the method of Benzie & Strain with slight modification.24 Film solution (30 μL) was added to 970 μL of FRAP reagent [250 mM sodium acetate buffer (pH 3.6)/10 mM TPTZ solution/20 mM ferric chloride solution with the ratio of 10
:
1
:
1. The mixture was immediately incubated in the dark at 50 °C for 4 min. The absorbance was monitored at 593 nm using the spectrophotometer, and the reducing activity of each sample was expressed as ferrous sulfate equivalent per g FRAP.
Statistical analysis
Film properties were measured with individually prepared films in triplicate and the values were presented as mean ± SD (standard deviation). Statistical analysis was done by one-way analysis of variance (ANOVA), and the significance of each mean value was determined (p < 0.05) with the Duncan's multiple range tests using the SPSS software (SPSS Inc., Chicago, IL, USA).
Results and discussion
Characterization of nanoparticles
Visual observation and UV-visible spectra. Various types of metallic and bimetallic nanoparticles were formed during the heating of the respective metal salt solutions with the AS extract. All the initial metal salt solutions with the AS extract was almost colorless, however, they were changed into ruby red, brownish yellow, pale brown, pale ruby, brownish red, and pale yellow after the formation of AuNPs, AgNPs, CuNPs, AuAgNPs, AuCuNPs, and AgCuNPs, respectively (Fig. 1). The development of color in the solution was due to the formation of metallic nanoparticles and the difference in color of different types of nanoparticles was attributed to the characteristic surface plasmon vibrations of respective nanoparticles.9,16 The progress in the formation of nanoparticles was monitored using the UV-visible spectrophotometer. The absorption maxima were recorded at 558, 424, and 344 nm for Au, Ag, and Cu nanoparticles, respectively. However, the peaks became broad in the case of bimetallic nanoparticles (Fig. 1). A similar peak broadening of bimetallic NPs has been reported, when Lansium domesticum fruit peel extract was used to synthesize the bimetallic NPs of gold and silver.16 The absorption maximum of AuAgNPs was observed at near 540 nm. The formation of AuAgNPs was presumably initiated with the nucleation of Ag atoms followed by co-deposition of Au and Ag, followed by the final deposition of Au on the surface of the seed. The presence of single peak indicated the formation of AuAgNPs, however, double peaks around 420 nm and 540 nm were due to the physical mixing of gold and silver nanoparticles.25
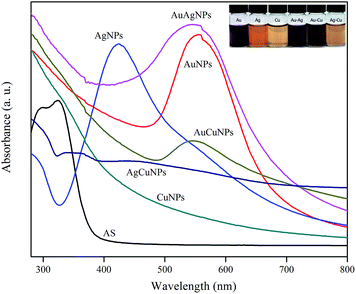 |
| Fig. 1 UV-visible spectra of nanoparticles. | |
Transmission electron microscopy. TEM was used to determine the morphology (size and shape) of the nanoparticles, and the resulting TEM images with different magnifications of the NPs were shown in Fig. 2. As shown in the TEM micrographs, all the NPs of AuNPs (20–30 nm), AgNPs (20–50 nm), CuNPs (<15 nm), AuAgNPs (10–20 nm), AuCuNPs (20–30 nm), and AgCuNPs (80–120 nm) and the NPs were well separated and spherical in shape with the size in the range of 20–120 nm depending on the type of NPs. However, the average particle size of the NPs determined by the dynamic light scattering (DLS) method was more than 100 nm (Table 1). The average particle size of these nanoparticles estimated by the TEM analysis was smaller than those determined by the DLS method. Since the DLS provides information on the hydrodynamic radius of particle in a solution, an apparent larger size of NPs determined by the DLS method compared with the TEM method was presumably due to the capping of nanoparticles by the AS extract.
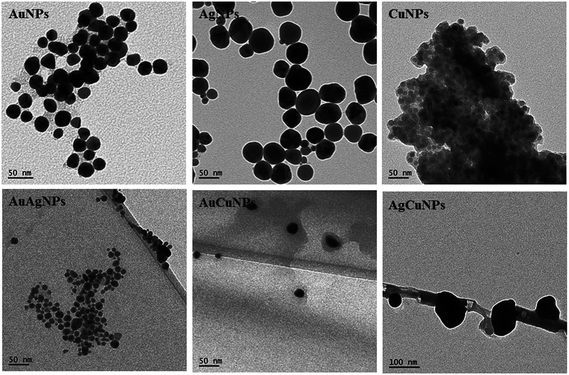 |
| Fig. 2 Transmission electron micrographs of nanoparticles. | |
Table 1 Zeta potential, particle size, and polydispersity index of nanoparticles
Samples |
Zeta potential (mV) |
Particle size by TEM (nm) |
Particle size by DLS (nm) |
Polydispersity index |
AuNPs |
−22.7 ± 1.4 |
20–30 |
120 ± 15 |
0.276 ± 0.037 |
AgNPs |
−42.3 ± 3.4 |
20–50 |
132 ± 13 |
0.214 ± 0.023 |
CuNPs |
−25.2 ± 1.8 |
<15 |
104 ± 15 |
0.127 ± 0.019 |
AuAgNPs |
−23.4 ± 2.6 |
10–20 |
108 ± 19 |
0.258 ± 0.048 |
AuCuNPs |
−22.1 ± 2.7 |
20–30 |
129 ± 13 |
0.294 ± 0.054 |
AgCuNPs |
−16.5 ± 1.9 |
80–120 |
157 ± 20 |
0.285 ± 0.032 |
Fourier transform infrared spectroscopy. FTIR analysis was carried out to check interactions between NPs and AS extract (Fig. 3). The vibrational peaks at 3300, 2931, 1749, 1635, 1550, 1456, 1240, 1033, 921, and 846 cm−1 were due to the presence of N–H of amines or O–H stretch of carboxylic acid or water, C–H stretch of alkanes, C
O stretch of carboxylic acid, N–H bend of primary amine, C–C stretch of aromatic ring, C–H bend of alkanes, C–N stretch of aromatic amines or C–O stretch of alcohol or carboxylic acid, C–N stretch of aliphatic amines, O–H bend of carboxylic acid, N–H of phenolic compounds, respectively in the AS extract.26 The presence of these peaks in the synthesized nanoparticles confirmed the presence of AS extract which acted as a capping material of the nanoparticles. Change in the peak intensity after formation of nanoparticles indicated the involvement of AS extract in the bioreduction process or in the binding with nanoparticles.27
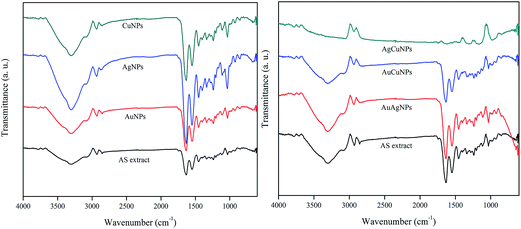 |
| Fig. 3 FTIR spectra of nanoparticles. | |
X-ray diffraction. The powder X-ray diffraction (XRD) patterns of all the NPs were presented in Fig. 4. All of the mono- and bimetallic nanoparticles were polycrystalline in nature and exhibited the characteristic diffraction peaks corresponding to the metallic gold, silver, and copper. All the nanoparticles exhibited intense (111) peaks and the resulting peaks revealed the crystalline nature of nanoparticles.28,29 Gold and silver exhibited similar XRD patterns due to their similar crystalline nature, however, copper diffraction planes were different from them. Diffraction planes corresponding to copper nanoparticles were not observed in the bimetallic nanoparticles such as AgCuNP and AuCuNP, which indicated the formation of alloy phase. The relative intensities of different crystalline planes and the peak width of (111) plane of bimetallic nanoparticles as well as monometallic particles were found to be different, which was an indication of alloy formation in the bimetallic nanoparticles.
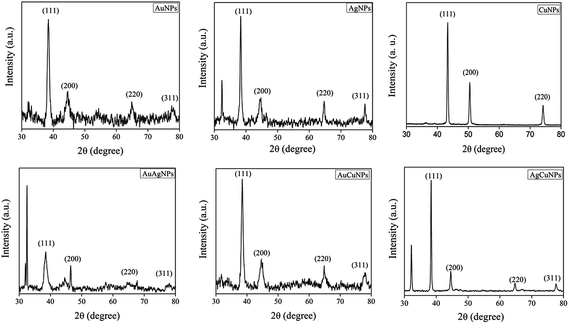 |
| Fig. 4 XRD patterns of metallic and bimetallic nanoparticles. | |
X-ray photoelectron spectroscopy. Fig. 5 shows the Au4f, Ag3d and Cu2p core-level spectra recorded from AuNPs, AgNPs, CuNPs, AuAgNPs, AuCuNPs, and AgCuNPs. The binding energies of Au4f7/2 level observed in the AuNPs, AuAgNPs, and AuCuNPs were 84 eV, 83.8 eV, and 83.7 eV, respectively. The binding energy corresponding to the metallic gold clearly indicated that the gold ions have been reduced into its metallic nanoparticles by the aqueous extract of AS. Similarly, Ag3d5/2 binding energy of AgNPs was 367.7 eV, however, Ag3d5/2 binding energy of bimetallic AuAgNPs and AgCuNPs nanoparticles were 367.8 and 367.4 eV, respectively. All the binding energy values were corresponding to the metallic silver, which confirmed the ability of AS extract for reducing the silver ions into silver nanoparticles. However, the binding energies corresponding to the Au4f7/2 and Ag3d5/2 core levels of bimetallic nanoparticles (AuAgNPs, AgCuNPs, and AuCuNs) found to be lower than those of the monometallic gold and silver nanoparticles. This is a strong evidence of alloy formation between the two metallic nanoparticles. Copper nanoparticles exhibited its Cu2p3/2 binding energy at 932.8 eV, which corresponded to the metallic copper. Although it has been generally known to be difficult to form copper nanoparticles, the aqueous extract of AS reduced copper ions into nanoparticles. However, the peak intensity of copper in bimetallic nanoparticles (AuCuNPs and AgCuNP) was very low, which was probably due to the low amount of copper in the bimetallic nanoparticles.
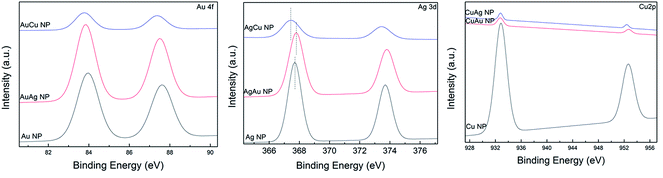 |
| Fig. 5 XPS core-level spectral data of AuNPs, AgNPs, CuNPs, AuAgNPs, AuCuNPs, and AgCuNPs. | |
Zeta-potential and dynamic light scattering. Zeta-potential of the nanoparticles prepared by the AS extract was measured to evaluate the stability of the nanoparticles in the colloidal system and the results were also shown in Table 1. Zeta-potential of the nanoparticles showed negative charges of −22.7 ± 1.4, −42.3 ± 3.4, −25.2 ± 1.8, −23.4 ± 2.6, −22.1 ± 2.7, and −16.5 ± 1.9 mV for AuNPs, AgNPs, CuNPs, AuAgNPs, AuCuNPs, and AgCuNPs, respectively. All the nanoparticle dispersions were charged high enough to maintain stability in the solution for long periods of time. The average particle size of these nanoparticles by dynamic light scattering (DLS) method was found to be 120 ± 14.8 nm for AuNPs, 132 ± 12.6 nm for AgNPs, 104 ± 15.3 nm for CuNPs, 108 ± 18.5 nm for AuAgNPs, 129 ± 13.2 nm for AuCuNPs, and 157 ± 19.6 nm for AgCuNPs with less than 0.3 of polydispersity indices for all the NPs (Table 1).
Antibacterial activity of nanoparticles
The use of AgNPs and CuNPs for the control of bacterial growth as well as for the treatment of burns and wound healing has been widely investigated.30,31 The metal ions have been assumed to bind with the bacterial membrane and to disintegrate the bacterial morphology. The metal ions and partially oxidized NPs have been known to possess antibacterial activity by either rupturing the negatively charged bacterial cell wall or destabilizing the outer membrane, thereby gaining the access to the mitochondria to interfere with the respiratory chain.32 Therefore, silver and copper possesses high antimicrobial properties and known to have considerably lower toxicity toward mammalian cells than toward bacteria when biomolecules are used as reducing and capping agents.33 The antimicrobial activity of the metallic and bimetallic NPs was tested using the broth micro-dilution method and the results of MIC/MBC values are presented in Table 2. The MIC/MBC values of AuNPs, AgNPs, CuNPs, AuAgNPs, AuCuNPs, and AgCuNPs against E. coli ATCC 25922 were >500/>500, 15.6/31.2, 250/500, 7.8/31.2, >500/>500, and 31.2/125 μM, respectively, and those against S. epidermis were >500/500, 31.2/125, >500/>500, 7.8/62.5, >500/>500, and 31.2/62.5 μM, respectively. It was observed that silver and their bimetallic nanoparticles showed stronger antimicrobial activity compared to copper or its bimetallic nanoparticles. Moreover, their antibacterial activity was stronger against Gram-negative than Gram-positive bacteria.
Table 2 Minimal inhibitory concentration (MIC) and minimum bactericidal concentration (MBC) of nanoparticles against E. coli ATCC 25922 and S. epidermis KCTC 1917
Nanoparticles |
MIC/MBC (μM) |
E. coli ATCC 25922 |
S. epidermis KCTC 1917 |
AuNPs |
>500/>500 |
>500/>500 |
AgNPs |
15.6/31.2 |
31.2/125 |
CuNPs |
250/500 |
>500/>500 |
AuAgNPs |
7.8/31.2 |
7.8/62.5 |
AuCuNPs |
>500/>500 |
>500/>500 |
AgCuNPs |
31.2/125 |
31.2/62.5 |
Characterization of gelatin-based nanocomposite films
Surface morphology. The gelatin and gelatin/NPs composite films were free-standing and flexible with smooth-surface. The surface morphology of the gelatin and gelatin/NPs composite films were examined by FE-SEM (Fig. 6). The gelatin film had a smooth and compact surface while gelatin/NPs nanocomposite films showed less smooth surfaces than the neat gelatin film. The NPs were uniformly distributed through the films, and no aggregation of particles was observed when metallic NPs (AuNPs, Ag NPs, and CuNPs) were incorporated into the gelatin, but some aggregated particles was observed in the nanocomposite films with bimetallic NPs (AuAgNPs, AuCuNPs, AgCuNPs). Similar surface morphology of nanocomposite film was observed when ZnO nanoparticles were blended with gelatin.17
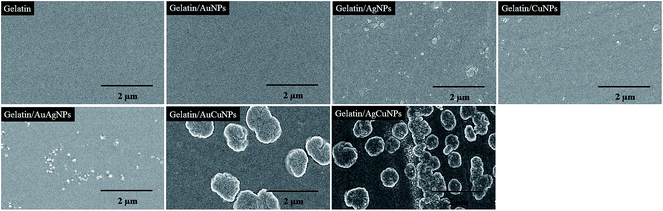 |
| Fig. 6 FE-SEM micrographs of gelatin and gelatin/NPs composite films. | |
Optical properties. Optical properties of films such as surface color and light transmittance are of importance because they might influence the product demand and consumer preference. Table 3 shows the optical properties of the composite films. The neat gelatin film was transparent without any color tint, however, the films incorporated with NPs were less transparent with different color shade depending on the type of NPs. As shown in Table 3, Hunter L-values of gelatin films decreased by blending with NPs, i.e., the Hunter L-value of gelatin film decreased from 93 to 25 depending on the type of NPs. The lightness of nanocomposite films decreased the most in the film reinforced with AuNPs followed by AgNPs and CuNPs. On the other hand, Hunter a-values increased after NPs incorporation and the degree of increase was also dependent on the types of NPs. The Hunter a-value (redness) was the highest for AuNPs followed by AuAgNPs, and AgNPs with the least increase in the CuNPs incorporated composite films. Increase in Hunter a-values indicate an increase in redness of the films. However, Hunter b-values (yellowness) of the gelatin/NPs nanocomposite films increased or decreased depending on the type of NPs, i.e., yellowness of the composite films decreased in the AuNPs and their bimetallic NPs incorporated films, but yellowness of the film increased in AgNPs and CuNPs incorporated films. The surface color of the nanocomposite films was mainly attributed to the apparent color of nanoparticles as shown in Fig. 1.34 Consequently, the incorporation of NPs remarkably increased the total color difference (ΔE) of the gelatin film from 2.2 up to 72.5, 29.2, 38.7, 71.6, 64.4, and 48.9 for Au, Ag, Cu, AuAg, AuCu, and AgCu NPs incorporated films, respectively. Similar results were observed with the addition of AgNPs and CuNPs into agar-based films.9,18
Table 3 Color and optical properties of gelatin/NPs composite filmsa
Film |
L |
a |
b |
ΔE |
T280 (%) |
T660 (%) |
The values were presented as mean ± SD. Any two means in the same column followed by the same superscript letter are not significantly different (p < 0.05) by Duncan's multiple range tests. |
Gelatin |
93.15 ± 0.16f |
−0.56 ± 0.03a |
4.57 ± 0.11c |
2.19 ± 0.16a |
29.4 ± 2.6b |
89.3 ± 0.9g |
Gel/AuNPs |
25.81 ± 0.20a |
22.55 ± 0.30f |
3.76 ± 0.08b |
72.49 ± 0.22g |
0.0 ± 0.0a |
50.1 ± 0.4e |
Gel/AgNPs |
38.20 ± 0.49c |
15.08 ± 0.50d |
12.24 ± 0.38e |
29.20 ± 0.30d |
0.1 ± 0.0a |
43.0 ± 0.9c |
Gel/CuNPs |
62.44 ± 1.05e |
5.20 ± 0.35b |
23.65 ± 0.15g |
38.65 ± 0.99b |
0.2 ± 0.0a |
73.4 ± 0.7f |
Gel/AuAgNPs |
25.26 ± 0.41a |
16.56 ± 0.82e |
7.34 ± 0.47d |
71.55 ± 0.20f |
0.1 ± 0.0a |
40.4 ± 1.4b |
Gel/AuCuNPs |
30.50 ± 0.70b |
5.72 ± 0.22c |
3.45 ± 0.24a |
64.38 ± 0.68e |
0.2 ± 0.0a |
35.6 ± 0.9a |
Gel/AgCuNPs |
48.56 ± 0.40d |
5.60 ± 0.07bc |
18.26 ± 0.07f |
48.88 ± 0.37c |
0.1 ± 0.0a |
48.9 ± 0.4d |
The light absorption properties and transparency of the gelatin and gelatin/NPs composite films were determined by measuring the light absorption spectra in the range of 200–700 nm as shown in Fig. 7. The control gelatin film showed the maximum absorption at 280 nm due to the absorption of light by tyrosine and tryptophan in the gelatin.18 The gelatin/NPs extract film showed the broad absorption peak between 280 and 350 nm due to the presence of gelatin and phenolic compounds in the AS extract and additional absorption peaks at a respective wavelength of NPs in addition to the peak of gelatin and AS extract. It has been reported that the phenolic compounds in the plant extract absorbs light in the UV range.16,29 The light transmittance of gelatin and gelatin/NPs composite films was measured at 280 and 660 nm, and the results are also presented in Table 3. The neat gelatin film was clear and transparent as indicated in the high transmittance values of 89.3 ± 0.9% in visible light (T660), however, the films with NPs showed significantly (p < 0.05) decreased transmittance at 660 nm. The AuCuNPs incorporated gelatin film was the least transparent and followed by the AuAgNPs, AgNPs, AgCuNPs, AuNPs, and CuNPs incorporated films, respectively. The control gelatin film exhibited high transmittance against UV light with the transmittance at 280 nm (T280) of 29.4 ± 2.6%, however, the UV light transmittance decreased substantially down to 0–0.2% after the formation of nanocomposite films with the metallic NPs. This result indicates that the gelatin/metallic NPs nanocomposite films have strong UV light screening function, and this fact suggests that they can be used for making hydrophilic anti-acne films with protection from UV light. UV irradiation of skin drives several signalling events that enhance reactive oxygen species and hydrogen peroxide production and decreases anti-oxidant enzymes. In either case, increased ROS production alters gene and protein structure, leading to skin damage.35 However, the inner part of skin is exposed to light and becomes more prone to damage by UV light at acne site. Moreover, heaps of evidence suggested that the skin lesions caused by acne can develop into squamous cell carcinoma, a skin cancer which has direct nexus with cumulative sun exposure. The prolonged exposure of skin to UV-A rays with long wavelengths penetrated deep into the skin that might be harmful to the connective tissues of skin and lead to skin damage.36
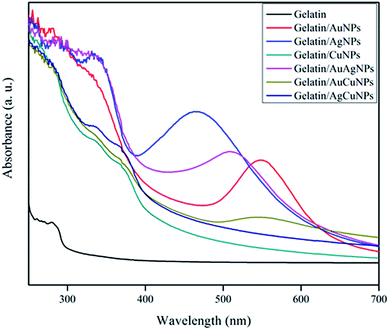 |
| Fig. 7 UV-visible spectra of gelatin/NPs composite films. | |
Mechanical properties. Mechanical properties of the neat gelatin and gelatin/NPs composite films were presented in Table 4. The thickness of the neat gelatin film was 54.0 ± 2.7 μm, which was not affected by the incorporation of nanoparticles except CuNPs. The tensile properties of gelatin film with the TS and E of 97.0 ± 6.9 MPa and 8.3 ± 1.4%, respectively, indicates that the gelatin film is strong and brittle. The tensile properties of the gelatin films were also influenced by the type of metallic NPs. The TS of nanocomposite films incorporated with metallic nanoparticles such as AuNPs, AgNPs, and CuNPs was not changed significantly (p < 0.05), but it decreased slightly when bimetallic nanoparticles such as AuAgNPs, AuCuNPs, and AgCuNPs were incorporated. The decrease in the TS of bimetallic NPs incorporated films might be attributed to the aggregation and larger size of bimetallic nanoparticles compared with metallic NPs resulted in the lower interfacial interaction between the NPs and gelatin matrix.
Table 4 Tensile properties, moisture content and water contact angle of gelatin/NPs composite filmsa
Film |
Thickness (μm) |
TS (MPa) |
E (%) |
EM (GPa) |
MC (%) |
WCA (°) |
The values were presented as mean ± SD. Any two means in the same column followed by the same superscript letter are not significantly different (p < 0.05) by Duncan's multiple range tests. |
Gelatin |
54.0 ± 2.7ab |
97.0 ± 6.9c |
8.3 ± 1.4a |
2.63 ± 0.07d |
10.3 ± 0.2c |
56.9 ± 0.7e |
Gel/AuNPs |
54.8 ± 0.9b |
97.4 ± 5.2c |
8.5 ± 1.0a |
2.55 ± 0.11cd |
9.7 ± 0.3b |
54.2 ± 0.5d |
Gel/AgNPs |
53.8 ± 2.0ab |
98.1 ± 6.4c |
8.3 ± 1.3a |
2.61 ± 0.13d |
9.2 ± 0.4ab |
49.1 ± 1.4ab |
Gel/CuNPs |
56.4 ± 1.8c |
89.9 ± 8.0ab |
8.8 ± 1.1a |
2.40 ± 0.13b |
9.6 ± 0.3b |
50.1 ± 1.2b |
Gel/AuAgNPs |
53.1 ± 3.2a |
87.3 ± 7.5a |
8.8 ± 1.2a |
2.30 ± 0.15a |
8.7 ± 0.7a |
48.7 ± 0.9a |
Gel/AuCuNPs |
56.8 ± 0.8c |
88.8 ± 6.3a |
8.5 ± 0.6a |
2.45 ± 0.23bc |
9.3 ± 0.3ab |
51.6 ± 0.2c |
Gel/AgCuNPs |
55.0 ± 2.2b |
94.1 ± 3.1bc |
9.0 ± 0.8a |
2.55 ± 0.09cd |
9.4 ± 0.1ab |
48.1 ± 0.4a |
On the other hand, the elongation at break (E) of gelatin/NPs nanocomposite films was not significantly (p > 0.05) different from that of the gelatin film. However, the stiffness of the films determined by the EM was slightly decreased after the formation of nanocomposite with metallic NPs. Though it is generally known that the distribution and density of inter and intra-molecular interactions between the polymer matrix and NPs determine the mechanical properties of the films, the mechanical properties of the gelatin/NPs nanocomposite films have not been changed distinctively.37 The gelatin-based nanocomposite films with strong mechanical properties are expected to maintain their integrity when they were applied as an anti-acne biopolymer hydrogel films or hydrophilic gelatin formulations. In addition, the biopolymer films can absorb water to be applied to the skin without any artificial adhesives for a prolonged time period. The prolonged contact of anti-acne films with the skin permits the slow release of effective NPs to treat the acne site.7
Moisture content and water contact angle. The moisture content (MC) and water contact angle (WCA) of the gelatin and gelatin/NPs composite films are shown in Table 4. Compared with the control films, the nanocomposite films exhibited slightly lower MC. The surface hydrophobicity of the neat gelatin and their nanocomposite films was tested by measuring the water contact angle (WCA) (Table 4). WCA is one of the basic wetting properties of films, which is usually used as an indicator for hydrophilic/hydrophobic properties of the film surface. The WCA of the neat gelatin film was 56.9 ± 0.7°. However, it decreased significantly (p < 0.05) after the formation of nanocomposite with NPs. The decrease in the values of WCA was due to the rough surface of gelatin film after NPs incorporation. The above mentioned properties suggested that the nanocomposite films have the ability to retain the moisture to make the superficial surface dry, which is essential for drying the acne lesions.
Antibacterial activity of composite films. Fig. 8 shows the antibacterial activity of the nanocomposite films against skin trouble causing bacteria, S. epidermis and E. coli determined by the colony count method. As expected, the gelatin and gelatin/AS extract films did not exhibit antibacterial activity against the test microorganisms. However, the gelatin/NPs nanocomposite films except AuNPs incorporated film showed antibacterial activity depending on the types of nanoparticles and the test organisms. AgNPs and their alloy incorporated films showed stronger antibacterial activity than those incorporated with CuNPs and their alloys. Among the bacterial pathogens, E. coli was more susceptible to AgNPs, AuAgNPs, AgCuNPs, and CuNPs than S. epidermis. Overall, the Gram-negative pathogens were highly suppressed by the nanocomposite films compared with the Gram-positive bacterial pathogens, which is probably due to the different membrane charge and thickness of cell wall of the bacterial strains.
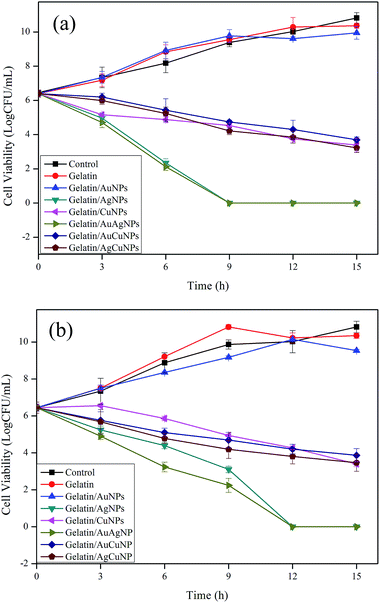 |
| Fig. 8 Antibacterial activity of gelatin and gelatin/NPs composite films against (a) E. coli ATCC 25922 and (b) S. epidermis KCT1917. | |
Generally, Gram-positive bacterial strains are composed of a thicker peptidoglycan (PG ∼ 20–80 nm) layer than the Gram-negative bacteria. The PG layer possesses linear polysaccharide chains cross-linked by short peptides and thus forms a complex structure that makes it difficult for NPs to penetrate into Gram-positive bacteria.38 On the contrary, Gram-negative bacteria are coated with a negatively charged outer membrane and thin peptidoglycan layer (∼7–8 nm), which facilitates penetration of NPs.38 Busolo et al. reported that antibacterial activities of silver based films are influenced by the availability of ionic silver for bacterial contact.39 Similar results were observed by Yoksan and Chirachanchai who reported that a chitosan/starch/AgNPs nanocomposite film inhibited the growth of Gram-negative bacteria (E. coli) more than the Gram-positive bacteria (S. aureus and B. cereus).40 Ghosh et al. observed that the incorporation of AgNPs into the agar films gave greater inhibition zones against E. coli than S. aureus.41 Vimala et al. prepared porous structure of antimicrobial nanocomposite films that exhibited superior antibacterial activity against E. coli, and Bacillus spp.42 This is probably due to the porous structure of the films which facilitates water absorption and thereby releases AgNPs efficiently from the films to the surrounding medium.
Several antimicrobial mechanisms have been proposed for silver and AgNPs against bacterial strains. Morones et al. examined the antimicrobial action of AgNPs against E. coli, P. aeruginosa, and Syphilis typhus using TEM.43 They observed noticeable cell membrane disruptions along with AgNPs that may be due to the interaction of AgNPs with phosphorus and sulfur-containing proteins and DNA, which prevents DNA replication and leads to cell death. Raffi et al. also demonstrated that a TEM analysis showed the presence of AgNPs in the membrane and interior part of the bacterium.44 It is believed that the penetration of AgNPs into the bacteria could inactivate enzymes and produce H2O2, leading to cell death. In addition, the positively charged AgNPs are believed to bind with negatively charged bacterial cell membranes, which disrupt cell walls and surface proteins, leading to cell death.38
It was expected that the well dispersed nano sized CuNPs with high surface area might interact with the bacterial cells leading to inhibition of bacteria. The inhibition of bacterial growth by CuNPs could be due to the inhibition of DNA replication and destruction of the cell wall of bacteria.45,46 On the other hand, depletion of the metal may cause the formation of pits in bacterial cell membranes and change the permeability of membrane, leading to cell death.47 The metallic NPs may accumulate in the bacterial cytoplasmic membrane, causing a significant increase in membrane permeability and lead to cell death. The antimicrobial activity test of the nanocomposite films was carried out in 100 mL conical flask containing 50 mL of diluted media (106 to 107 CFU mL−1 bacteria) and 5 cm × 5 cm film samples (∼100 mg films) at 37 °C with mild shaking. Since, the gelatin is a water soluble protein, the gelatin-based nanocomposite films were dissolved in media completely within 3–4 h. Hence, the antibacterial activity presented in the present study was the combined effects of total nanoparticles and metal ions which was leached out from the film.
There is also a concern about the safety of nanoparticles. Presently, little information is available to assess the safety for human beings or the effect on the ecosystem of NPs.38 On the other hand, a beneficial effect on the health of small amounts of NPs has also been published in the literature.48 The present results show that the gelatin/AgNPs, gelatin/AuAgNPs, gelatin/CuNPs, and gelatin/AgCuNPs composite films were very effective against acne inducing bacteria, which indicates high potential use of the composite films as acne treatment hydrogel films.
Antioxidant activity of composite films. Antioxidant activity of gelatin/NPs composite films determined by DPPH, ABTS, and FRAP activities are presented in Table 5. As shown in Table 5, antioxidant activity of gelatin/NPs composite films was varied depending on the type of NPs and their alloys. Among the NPs tested, AgNPs-incorporated composite film exhibited the highest activity and followed by the AuNPs, and the CuNPs showed the lowest antioxidant activity. The DPPH activity of gelatin/AgNPs, gelatin/AuNPs, and gelatin/CuNPs were 89.7%, 66.0, and 22.9%, respectively, and that of gelatin/bimetallic NPs composite films were varied depending on their component NPs. For example, gelatin/CuAgNPs composite films exhibited higher antioxidant activity (50.6%) than gelatin/CuNPs (22.4%) films, which indicates that the antioxidant activity of CuNPs could be increased by making alloy with AgNPs. Similar trend of antioxidant activity of the gelatin/NPs composite films were observed when it was determined by the ABTS and FRAP activity methods. Again, the CuNPs and its alloy (AuCuNPs) showed the lowest antioxidant activity, which was probably due to its prompt oxidation in an aqueous solution to reduce antioxidant activity.49 The free radical scavenging activity of the gelatin/NPs composite films was occurred by the interaction between biomolecules and nanoparticles, as evidenced by the antioxidant activity of metallic (gelatin/AgNPs) and bimetallic (gelatin/AuAgNPs and gelatin/AgCuNPs) nanocomposite films.50 Rajakannu et al. and Veeraapandian et al. also found that the antioxidant activity of NPs (such as AgNPs and AuNPs) synthesized by fruit extract and E. coli, respectively, increased when they formed alloys.51,52 The high antioxidant activity of gelatin and silver based nanocomposite films along with strong UV light barrier properties is expected to protect the cells from oxidative damage and free radical production.
Table 5 Antioxidant activities of gelatin/NPs composite filmsa
Sample |
DPPH activity (%) |
ABTS activity (μmol mL−1) |
FRAP activity (μmol mL−1) |
The values were presented as mean ± SD. Any two means in the same column followed by the same superscript letter are not significantly different (p < 0.05) by Duncan's multiple range tests. |
Gelatin/AuNPs |
66.0 ± 0.3d |
1413.3 ± 0.1e |
562.9 ± 0.2c |
Gelatin/AgNPs |
89.7 ± 0.2f |
1415.8 ± 0.0f |
727.3 ± 0.2e |
Gelatin/CuNPs |
22.9 ± 0.2a |
1165.4 ± 0.2b |
467.6 ± 0.4a |
Gelatin/AuAgNPs |
83.3 ± 0.1e |
1404.0 ± 0.1d |
656.2 ± 0.2d |
Gelatin/AuCuNPs |
28.0 ± 0.2b |
1110.7 ± 0.2a |
470.5 ± 0.1a |
Gelatin/AgCuNPs |
50.5 ± 0.2c |
1283.8 ± 0.2c |
538.7 ± 0.3b |
Conclusions
Artemisia scoparia extract was used as combined reducing and capping agents for the preparation of six different types of metallic and bimetallic nanoparticles. All gelatin/metallic NPs composite film exhibited UV light screening function. Except gelatin/AuNPs film, the other gelatin/NPs composite films, especially AgNPs and AuAgNPs incorporated films, exhibited strong antimicrobial activity against both Gram-positive and Gram-negative bacteria. Except gelatin/CuNPs composite films, the other nanocomposite films, especially AgNPs and AuAgNPs incorporated films, exhibited strong antioxidant activity. The antimicrobial property is highly useful to minimize the growth of skin trouble causing bacteria, and the UV barrier property of those films is expected to protect the skin from UV radiation. The gelatin/metallic NPs composite films with UV barrier, antimicrobial, and antioxidant functions have a high potential for the application as a biocompatible acne treatment hydrogel films.
Acknowledgements
This work was supported by Business for Cooperative R&D between Industry, Academy, and Research Institute funded by the Korea Small and Medium Business Administration in 2015 (Grants No. C0297411) and Solar Salt Research Center of Mokpo National University from Ministry of Oceans and Fisheries of Korea (Grant No. 20130290).
References
- H. C. Williams, R. P. Dellavalle and S. Garner, Acne vulgaris, J.-Lancet, 2012, 379, 361–372 CrossRef.
- N. Janiczek-Dolphin, J. Cook, D. Thiboutot, J. Harness and A. Clucas, Can sebum reduction predict acne outcome?, Br. J. Dermatol., 2010, 163, 683–688 CrossRef CAS PubMed.
- B. Wei, Y. Pang and H. Zhu, et al., The epidemiology of adolescent acne in North East China, J. Eur. Acad. Dermatol. Venereol., 2010, 24, 953–957 CrossRef CAS PubMed.
- W. P. Bowe, J. B. Glick and A. R. Shalita, Solodyn and updates on topical and oral therapies for acne, Curr. Dermatol. Rep., 2012, 1, 97–107 CrossRef.
- J. J. Leyden, N. Preston, C. Osborn and R. W. Gottschalk, In vivo effectiveness of adapalene 0.1%/benzoyl peroxide 2.5% gel on antibiotic-sensitive and resistant Propionibacterium acnes, J. Clin. Aesthet. Dermatol., 2011, 4, 22–26 Search PubMed.
- M. T. Chomnawang, S. Surassmo, K. Wongsariya and N. Bunyapraphatsara, Antibacterial activity of thai medicinal plants against methicillin-resistant Staphylococcus aureus, Fitoterapia, 2009, 80, 102–104 CrossRef CAS PubMed.
- E. Mathiowitz, B. E. Laulicht, S. H. Bakhru and S. S. Steiner, Anti-Acne Topical Films, US Pat., 206,523, 2014.
- P. Kanmani and J. W. Rhim, Physicochemical properties of gelatin/silver nanoparticle antimicrobial composite films, Food Chem., 2014, 148, 162–169 CrossRef CAS PubMed.
- S. Shankar, X. Teng and J. W. Rhim, Properties and characterization of agar/CuNPs bionanocomposite films prepared with different copper salts and reducing agents, Carbohydr. Polym., 2014, 114, 484–492 CrossRef CAS PubMed.
- M. Qasim, S. Gulzar and M. A. Khan, Halophytes as medicinal plants, NAM Meeting, Denizli, Turkey, 2011 Search PubMed.
- M. S. Shafi, M. Y. Ashraf and G. Sarwar, Wild medicinal plants of Cholistan area of Pakistan, Pak. J. Biol. Sci., 2001, 4, 112–116 CrossRef.
- G. Agoramoorthy, F. A. Chen, V. Venkatesalu, D. H. Kuo and P. C. Shea, Evaluation of antioxidant polyphenols from selected mangrove plants of India, Asian J. Chem., 2008, 20, 1311–1322 CAS.
- R. Qureshi and G. R. Bhatti, Ethnobotany of plants used by the Thari people of Nara desert, Pakistan, Fitoterapia, 2008, 79, 468–473 CrossRef PubMed.
- S. B. Erel, G. Reznicek, S. G. Senol, N. U. K. Yavasogulu, S. Konyalioglu and A. U. Zeybek, Antimicrobial and antioxidant properties of Artemisia L. species from western Anatolia, Turk. J. Biol., 2012, 36, 75–84 CAS.
- H. P. Singh, S. Mittal, S. Kaur, D. R. Batish and R. K. Kohli, Chemical composition and antioxidant activity of essential oil from residues of Artemisia scoparia, Food Chem., 2009, 114, 642–645 CrossRef CAS.
- S. Shankar, L. Jaiswal, R. S. L. Aparna and R. G. S. V. Prasad, Synthesis, characterization, in vitro biocompatibility, and antimicrobial activity of gold, silver and gold silver alloy nanoparticles prepared from Lansium domesticum fruit peel extract, Mater. Lett., 2014, 137, 75–78 CrossRef CAS.
- S. Shankar, X. Teng, G. Li and J. W. Rhim, Preparation, characterization, and antimicrobial activity of gelatin/ZnO nanocomposite films, Food Hydrocolloids, 2015, 45, 264–271 CrossRef CAS.
- S. Shankar and J. W. Rhim, Amino acid mediated synthesis of silver nanoparticles and preparation of antimicrobial agar/silver nanoparticles composite films, Carbohydr. Polym., 2015, 130, 353–363 CrossRef CAS PubMed.
- J. W. Rhim, S. I. Hong, H. M. Park and P. K. W. Ng, Preparation and characterization of chitosan-based nanocomposite films with antimicrobial activity, J. Agric. Food Chem., 2006, 54, 5814–5822 CrossRef CAS PubMed.
- J. W. Rhim and L. F. Wang, Mechanical and water barrier properties of agar/k-carrageenan/konjac glucomannan ternary blend hydrogel film, Carbohydr.
Polym., 2013, 96, 71–81 CrossRef CAS PubMed.
- S. Shankar, J. P. Reddy, J. W. Rhim and H. Y. Kim, Preparation, characterization, and antimicrobial activity of chitin nanofibrils reinforced carrageenan nanocomposite films, Carbohydr. Polym., 2015, 117, 468–475 CrossRef CAS PubMed.
- W. Brand-Williams, M. E. Cuvelier and C. Berset, Use of a free radical method to evaluate antioxidant activity, LWT--Food Sci. Technol., 1995, 28, 25–30 CrossRef CAS.
- R. Re, N. Pellegrini, A. Pannala, M. Yang and E. C. Rice, Antioxidant activity applying an improved ABTS radical cation decolorization assay, Free Radical Biol. Med., 1999, 26, 1231–1237 CrossRef CAS PubMed.
- I. F. F. Benzie and J. J. Strain, The ferric reducing ability of plasma (FRAP) as a measure of “antioxidant power”: the FRAP assay, Anal. Biochem., 1996, 239, 70–76 CrossRef CAS PubMed.
- K. S. Shin, J. H. Kim, I. H. Kim and K. Kim, Novel fabrication and catalytic application of poly(ethylenimine)-stabilized gold–silver alloy nanoparticles, J. Nanopart. Res., 2012, 14, 734–735 CrossRef.
- M. Nishizawa, H. Nishide, S. Kosela and Y. Hayashi, Structure of lansiosides: biologically active new triterpene glycosides from Lansium domesticum, J. Org. Chem., 1983, 48, 4462–4466 CrossRef CAS.
- B. Ankamwar, Biosynthesis of gold nanoparticles (green-gold) using leaf extract of Terminalia catappa, Chem.–Eur. J., 2010, 7, 1334–1339 CAS.
- S. Shankar and J. W. Rhim, Effect of copper salts and reducing agents on characteristics and antimicrobial activity of copper nanoparticles, Mater. Lett., 2014, 132, 264–271 CrossRef.
- S. Shankar, J. Chorachoo, L. Jaiswal and S. P. Voravuthikunchai, The effect of reducing agent concentrations and temperature on characteristics and antimicrobial activity of silver nanoparticles, Mater. Lett., 2014, 137, 160–163 CrossRef CAS.
- B. Boonkaew, P. Suwanpreuksa, L. Cuttle, P. M. Barber and P. Supaphol, Hydrogels containing silver nanoparticles for burn wounds show antimicrobial activity without cytotoxicity, J. Appl. Polym. Sci., 2014, 131, 40215–40224 CrossRef.
- M. Tiwari, K. Narayanan, M. B. Thakar, H. V. Jagani and J. Venkata Rao, Biosynthesis and wound healing activity of copper nanoparticles, IET Nanobiotechnol., 2014, 8, 230–237 CrossRef PubMed.
- K. B. Holt and A. J. Bard, Interaction of silver(I) ions with the respiratory chain of Escherichia coli:
an electrochemical and scanning electrochemical microscopy study of the antimicrobial mechanism of micromolar Ag+, Biochemistry, 2005, 44, 13214–13223 CrossRef CAS PubMed. - H. K. Daima, P. R. Selvakannan, A. E. Kandjani, R. Shukla, S. K. Bhargava and V. Bansal, Synergistic influence of polyoxometalate surface corona towards enhancing the antibacterial performance of tyrosine-capped Ag nanoparticles, Nanoscale, 2014, 6, 758–765 RSC.
- S. Shankar and J. W. Rhim, Preparation of nanocellulose from micro-crystalline cellulose: the effect on the performance and properties of agar-based composite films, Carbohydr. Polym., 2016, 135, 18–26 CrossRef CAS PubMed.
- L. Rittié and G. J. Fisher, UV-light-induced signal cascades and skin aging, Ageing Res. Rev., 2002, 1, 705–720 CrossRef.
- A. Angel, D. W. Osborne and G. J. Dow, Topical acne vulgaris medication with a sunscreen, Patent no. US7326408 B2, 2008.
- H. Chambi and C. Grosso, Edible films produced with gelatin and casein crosslinked with transglutaminase, Food Res. Int., 2006, 39, 458–466 CrossRef CAS.
- T. V. Duncan, Application of nanotechnology in food packaging and food safety: barrier materials, antimicrobials and sensors, J. Colloid Interface Sci., 2011, 363, 1–24 CrossRef CAS PubMed.
- M. A. Busolo, P. Fernandez, M. J. Ocio and J. M. Lagaron, Novel silver-based nanoclay as an antimicrobial in polylactic acid food packaging coatings, Food Addit. Contam., Part A, 2010, 27, 1617–1626 CrossRef CAS PubMed.
- R. Yoksan and S. Chirachanchai, Silver nanoparticle-loaded chitosan-starch based films: fabrication and evaluation of tensile,
barrier and antimicrobial properties, Mater. Sci. Eng., C, 2010, 30, 891–897 CrossRef CAS.
- S. Ghosh, R. Kaushika, K. Nagalakshmi, S. L. Hoti, G. A. Menezes, B. N. Harish and H. N. Vasan, Antimicrobial activity of highly stable silver nanoparticles embedded in agar–agar matrix as a thin film, Carbohydr. Res., 2010, 345, 2220–2227 CrossRef CAS PubMed.
- K. Vimala, Y. M. Mohan, K. S. Sivudu, K. Varaprasad, S. Ravindra, N. N. Reddy, Y. Padma, B. Sreedhar and K. MohanaRaju, Fabrication of porous chitosan films impregnated with silver nanoparticles: a facile approach for superior antibacterial application, Colloids Surf., B, 2010, 76, 248–258 CrossRef CAS PubMed.
- J. R. Morones, J. L. Elechiguerra, A. Camacho, K. Holt, J. B. Kouri and J. T. Ramirez, The bactericidal effect of silver nanoparticles, Nanotechnology, 2005, 16, 2346–2353 CrossRef CAS PubMed.
- M. Raffi, F. Hussain, T. M. Bhatti, J. I. Akhter, A. Hameed and M. M. Hasan, Antibacterial characterization of silver nanoparticles against E. coli ATCC 15224, J. Mater. Sci. Technol., 2008, 24, 192–196 CAS.
- Q. L. Feng, J. Wu, G. Q. Chen, F. Z. Cui, T. N. Kim and J. O. Kim, A mechanistic study of the antibacterial effect of silver ions on E. coli and Staphylococcus aureus, J. Biomed. Mater. Res., 2000, 52, 662–668 CrossRef CAS PubMed.
- M. Singh, S. Singh, S. Prasad and I. S. Gambhir, Nanotechnology in medicine and antibacterial effect of silver nanoparticles, Dig. J. Nanomater. Biostruct., 2008, 3, 115–122 Search PubMed.
- N. A. Amro, L. P. Kotra, K. Wadu-Mesthrige, A. Bulychev, S. Mobashery and G. Liu, High-resolution atomic force microscopy studies of the Escherichia coli outer membrane: structural basis for permeability, Langmuir, 2000, 16, 2789–2796 CrossRef CAS.
- V. K. Sharma, R. A. Yngard and Y. Lin, Silver nanoparticles: green synthesis and their antimicrobial activities, Adv. Colloid Interface Sci., 2009, 145, 83–96 CrossRef CAS PubMed.
- L. C. Wu, H. W. Hsu, Y. C. Chen, C. C. Chiu, Y. I. Lin and J. A. Ho, Antioxidant and antiproliferative activities of red pitaya, Food Chem., 2006, 95, 319–327 CrossRef CAS.
- P. Dauthal and M. Mukhopadhyay, In vitro free radical scavenging activity of biosynthesized gold and silver nanoparticles using Prunus armeniaca (apricot) fruit extract, J. Nanopart. Res., 2013, 15, 1366 CrossRef.
- S. Rajakannu, S. Shankar, S. Perumal, S. Subramanian and G. P. Dhakshinamoorthy, Biosynthesis of silver nanoparticles using Garcinia mangostana fruit extract and their antibacterial, antioxidant activity, Int. J. Curr. Microbiol. Appl. Sci., 2015, 4, 944–952 Search PubMed.
- S. Veeraapandian, S. N. Sawant and M. Doble, Antibacterial and antioxidant activity of protein capped silver and gold nanoparticles synthesized with Escherichia coli, J. Biomed. Nanotechnol., 2012, 8, 140–148 CrossRef CAS PubMed.
Footnote |
† SS and LJ contributed equally and shared the first authorship. |
|
This journal is © The Royal Society of Chemistry 2016 |
Click here to see how this site uses Cookies. View our privacy policy here.