DOI:
10.1039/C6RA09725A
(Communication)
RSC Adv., 2016,
6, 66767-66773
Molecular sieving effect of zeolites on the properties of PVA based composite membranes for total heat recovery in ventilation systems
Received
14th April 2016
, Accepted 30th June 2016
First published on 4th July 2016
Abstract
In this article, composite membranes of polyvinyl alcohol (PVA) with zeolites of three different pore sizes of 0, 3, and 4 Å were prepared and studied. The molecular sieving effect of the zeolite on the permeation of water vapor and CO2, the efficiency of total heat exchange and the morphology, mechanical properties, and thermal stability of the membranes were investigated related to their compositions.
Introduction
With the escalation of indoor air pollution, the design of high quality ventilation systems to timely vent out exhausted indoor air and vent in fresh outdoor air had become more and more desirable in our society.1 However, ventilation systems tend to add load to building air conditioning systems by introducing “coldness” and “hotness” into the building during the air exchange,2 contributing to about half of the energy used for building maintenance, or almost one sixth to one eighth of the total energy consumed in our society. How to maintain indoor air quality with reduced energy consumption, especially those caused by the ventilation processes, has become a central topic.
To reduce the energy consumption of an air conditioner during ventilation, it is important to recover the energy from the air streams in the ventilation. Theoretically, the energy recovery may be realized by recovering the sensible heat and latent heat contained in the air streams. The sensible heat relating to temperature change without a transforming physical state can be calculated from the change of the dry-bulb temperature while the latent heat relating to a phase transition without changing the temperature resulted mainly from the change of the moisture content in the air. The sum of sensible heat and latent heat is called the “total heat” or, generally, the “enthalpy” of wet air, and the concept of total heat exchange is actually the exchange of both moisture and heat.
Different types of Energy Recovery Ventilation (ERV) systems to recover energy through energy exchange between fresh air and exhaust air have been designed to reduce energy consumption of air conditioning.3 ERV systems in the designs of two-phase thermo-siphon-loops, rotary wheels,4–7 fixed-plates,8 run around coils,9 and heat pipes10 have been researched and designed for practical applications. The fixed-plate ERV systems are widely used in factories, buildings and other public places,2 whose energy exchange medium separating the exhaust air and fresh air were, traditionally, made from thin heat conducting metal such as aluminum foils which only allow sensible heat exchange. Total heat exchange membranes made of cellulose or synthetic polymers had shown a superior energy recovery property,11 allowing not only the exchange of heat but also the moisture.12–14
In the process of energy exchange for ERV, to prevent the contamination of fresh air by undesirable gases, such as CO2, from the exhaust air, total heat exchange membranes should also have a high gas barrier property when performing the heat and moisture exchange. The key strategy for designing high performance total heat exchange membranes is to maximize their selectivity for moisture over CO2.
The general effective way to increase the transfer of moisture in polymeric membranes, was to include polar or ionic functional groups to form water permeable channels. For example, polyvinyl alcohol (PVA) is a kind of water-soluble polymer, mainly produced by the hydrolysis of polyvinyl acetate.15–17 It had been used as a good base material for making high performance total heat exchange membranes for its good hydrophilicity, chemical stability, and biological compatibility.18,19 Total heat exchange membranes made of chitosan (CH) and guar gum (GG) had also been studied by Rao et al.20 To increase the gas barrier property of polymeric membranes, inorganic particles or nano-sheets may be composited into the membrane matrix. For example, Liu et al. in our groups had prepared and studied poly(vinyl chloride)/sodium-montmorillonite (PVC/Na+-MMT) composite total heat exchange membranes.2
Trade-offs have been observed between a high moisture permeability and good gas barrier property for total heat exchange membranes. In the tight membranes with little free volume, the exchange of heat and moisture are low although with good gas barrier property. On the other hand, the structure of loose membranes with a lot of channels or cracks tend to have increased free volume for moisture exchange but have decreased barrier properties for undesirable gases, such as CO2. The aim of this study is to find a way to break the trade-off between high moisture permeability and good gas barrier property and develop high performance total heat exchange membranes to be used in energy recovering ventilation systems.
The kinetic diameter of CO2 is about 3.3 Å, slightly larger than that of a water molecule, 2.6 Å.21,22 Is there any way to use the molecular sieving effect to improve the permselectivity of total heat exchange membranes for moisture over CO2? Zeolites are porous alkali aluminosilicates, which may be designed to have very high selectivity for water.23–27 Their pores can allow the transportation of molecules whose sizes are smaller. In this paper, we want to explore the possibility of using zeolites to create well defined free volume in the polymer membrane matrix to increase the permeation selectivity just for water. Therefore, zeolites with varied pore sizes, were chosen to prepare PVA based composite total heat exchange membranes and their moisture and CO2 transmission as well as morphology, mechanical property, thermal stability and total heat exchange effectiveness were investigated.
Experimental section
Materials
Powder of PVA-1799, was provided by the Sinopharm Chemical Reagent Co., Ltd, China; nonporous zeolite (mean particle size of 3–5 μm), 3A zeolite (pore size of 3 Å, mean particle size of 3–5 μm) and 4A zeolite (pore size of 4 Å, mean particle size of 3–5 μm), were provided by Anhui MingmeiChem Co., Ltd.
Composite membrane fabrication processes
The powders of nonporous zeolite, 3A zeolite and 4A zeolite were dried in a muffle furnace at 220 °C for 3 h, then put into an airtight glassware dryer with saturated salt water in the bottom for 24 h. Then a certain amount of weighed PVA was dissolved in the deionized water under mechanical stirring. After complete dissolution of PVA, the zeolite with varied ratios was added into the solution under high-speed mechanical stirring for 12 h. Then the mixture was vibrated under ultrasonic wave to disperse for 6 h. Finally, the formed suspension was cast on the surface of a clean glass, and further dried at 60 °C. Then the membranes were peeled off after the solvent evaporation. The thickness of membranes was kept at 40 ± 5 μm by controlling the mass of the cast solution. The compositions of casting solutions are listed in Table 1.
Table 1 The composition of the casting solution
Sample symbol |
PVA (g) |
H2O (g) |
Zeolites (g) |
M0 |
6 g |
94 g |
0 g |
M1 |
6 g |
94 g |
1 g |
M2 |
6 g |
94 g |
2 g |
M4 |
6 g |
94 g |
4 g |
M6 |
6 g |
94 g |
6 g |
Scanning electron microscopy
A Zeiss EVO18 was used for Scanning Electron Microscopy (SEM) to observe the morphology and configuration of the surfaces. Before SEM examination, samples were coated with a layer of gold film.
Tensile tests
Samples were cut into rectangle shapes with sizes of 200 mm × 10 mm. Tensile strength and elongation at the break of membrane samples were measured with an Instron 5567 testing machine at a speed of 50 mm min−1.
Thermogravimetric analysis
The thermal decomposition studies were performed over a temperature range of 50–600 °C using a Mettler Toledo TGA/DSC1 system under flowing nitrogen atmosphere (50 ml min−1) at a heating rate of 20 °C min−1.
Water vapor transmission test
Water vapour transmission tests of membranes were conducted with a Labthink Perme W3/060 system. The tests were conducted at 38.0 °C and 90.0% relative humidity. In this test, the membrane was put on the chamber containing double-distilled water in the bottom. The relative humidity of the air below the membrane was defaulted to be 100%, and the relative humidity of the air above the membrane was adjusted to 10%, so the difference between both sides of membrane was 90%. The temperature of the chamber was kept at 38 °C. The water vapor transmission rate through the membrane was calculated by weighing the loss of water in the chamber every 30 min. The schematic diagram of the water vapor transmission test is shown in Fig. 1.
 |
| Fig. 1 The schematic diagram for the water vapor transmission test. | |
Carbon dioxide transmission test
Carbon dioxide (CO2) transmission tests were carried out with a Labthink VAC-V2 system using the differential pressure (0.1 MPa) method at 23 °C. The membrane was put in the airtight test chamber. The upper space was filled with CO2 (0.1 MPa), and the lower space was vacuumized. Then the CO2 transmitted through the membrane by pressure difference. The pressure of the lower space was recorded by a pressure sensor. The CO2 transmission coefficient was calculated by the pressure varying with time. The schematic diagram of the CO2 transmission test is shown in Fig. 2.
 |
| Fig. 2 The schematic diagram for the CO2 transmission test. | |
Total heat exchange effectiveness tests
The total exchange effectiveness of the membrane was carried on the in-house system as shown in Fig. 3. The initial dehumidified main air stream was split into four sub streams, two of which go through the bubbler to have high relative humidity. The temperature and humidity of Airflow 1 and Airflow 3 were adjusted by varying the mixing ratios of the incoming two sub-streams with different temperature and humidity. The temperature and relative humidity of Airflow 1 was kept at (35 ± 0.2) °C and (40% ± 1%) to represent the hot and humid fresh air from outside in the summer while the temperature and relative humidity of Airflow 3 was kept at (25 ± 0.2) °C and (15% ± 1%) to represent the cool and dry exhaust air from indoors. The volume flow rates of Airflow 1 and Airflow 3 were kept at (15 ± 1) L h−1. The membrane was fixed in the exchanger to allow the heat and moisture exchange between Airflow 1 and Airflow 3 with the oblong exchange area of 30 cm2 and the flow channel height of 0.4 cm. The temperature and humidity of airflows were monitored using sensors while the moisture and enthalpy of each airflow were calculated using psychrometric charts. The temperature exchange effectiveness (ηT), moisture exchange effectiveness (ηM) and enthalpy exchange effectiveness (ηE) were figured out as formula (1)–(3), where T represents temperature, M represents moisture and E represents enthalpy. The standard deviations for exchange effectiveness were in the range of 4–7%. |
 | (1) |
|
 | (2) |
|
 | (3) |
 |
| Fig. 3 Test system for total heat exchange effectiveness. | |
Results and discussion
The molecular structure of polyvinyl alcohol (PVA) has provided many lateral hydroxyl groups to potentially transport moisture and create high crystalline zones with little free volume to slow down the permeation of CO2.28 As shown in the SEM images in Fig. 4, membrane M0 from pure PVA showed a very dense cross sectional structure, through which gas molecules may transfer according to the adsorb–diffuse–desorb model.29,30 As illustrated in Fig. 5, the molecules in the gas phase needed to be firstly absorbed on the surface of membranes and then diffused to the other side by the driving of the concentration difference, which then desorbed into the gas phase. The adsorption process may be accelerated by increasing the roughness of surfaces and the solubility of gases on the membrane surfaces; the diffusion of gases through the membranes can be greatly enhanced by any free volume introduced in the cross section while the desorption step can be impacted by the surface roughness and the concentration of components in the gas stream.
 |
| Fig. 4 SEM images of the surface (left) and cross section (right) of PVA/3A zeolite composite membranes. | |
 |
| Fig. 5 Illustration for gas molecule transfer in a PVA membrane. | |
The addition of a zeolite could change the structure and property of the PVA membrane. Firstly, it could reduce the solubility and diffusion of small molecules in the PVA matrix by reducing the PVA proportion on the surfaces and in the cross sections. Secondly, the internal porosity of zeolitic particles and irregular gaps created in the interphasal boundary areas could act as free volume to accelerate gas permeability. As shown in Fig. 4, these irregular cracks or holes (marked with a red box) became visible on M6. Thirdly, the potential increase in surface roughness after zeolitic addition could also favor the solubility of gases to the membranes for higher transfer rates. The protuberances on the membrane surface influenced airflow to make turbulence in the membrane surface regions and increase the probability and time of air touching with the membrane surface, so the heat could efficiently transfer through the membrane.31
The addition of a zeolite had changed the gas molecule transferring mechanism by changing the structure of the PVA membrane. As illustrated in Fig. 6, small molecules have three major routes to cross the membranes: Route (1) through the pure PVA matrix, Route (2) through the pores inside the zeolite particles, and Route (3) through the gaps or interphasal boundaries. The total transferring rate is the sum of rates of three parts. The transfer rate through Route (1) of the PVA matrix related to PVA content, which would decrease with addition of the zeolite. The transfer rate through Route (2) in the pores of the zeolite related to the zeolite content and pore size. The larger the pore size, the faster the transfer rate. The transfer rate through Route (3) in the gaps or interphase boundary related to the zeolitic content, the interaction between different components and the homogeneity of the membranes.
 |
| Fig. 6 Illustration for the gas molecule transfer in PVA/zeolite composite membranes (1, 2 and 3 stand for the gas transfer through PVA matrix, zeolite pores and boundary gaps respectively. The black spots stand for the gas molecule, and the dotted line balls with channels stand for the porous zeolite. The broken circle area stands for gaps or interphasal boundaries). | |
As shown in Fig. 4, similar to pure PVA membrane M0, when the inorganic zeolite content was lower than M2 (25% by weight), as in M1 and M2, the inorganic zeolite particles homogeneously dispersed inside the tight continuous PVA matrix. Transfer through Route (3) was limited. The membrane with nonporous zeolite showed increased barrier properties for both H2O and CO2 as shown in Fig. 7 and 8. When porous zeolites were used, to avoid the dense PVA matrix, small gas molecules tended to diffuse through the free volume created by the pores inside the zeolites via Route (2). From the experimental results, the molecular sieving effect of the pores had play a big role on the gas diffusion rates and the total heat exchange properties of the membranes under these conditions. As shown in Fig. 7, from M0 to M2, with the increase of the 3A zeolite and 4A zeolite, the water vapor transmission coefficient increased continually because the kinetic diameters of the 3A zeolite and 4A zeolite were both larger than the kinetic diameter of the H2O molecule (2.6 Å). As shown in Fig. 8, CO2 diffusion was thwarted by the addition of the 3A zeolite and nonporous zeolite by having a larger kinetic diameter of 3.3 Å. This agreed with what was previously reported that 3A zeolite had made a CO2 diffusion route longer.32–35
 |
| Fig. 7 Water vapor transmission rate of composite membranes with different zeolites. | |
 |
| Fig. 8 CO2 transmission coefficient of composite membranes with different zeolites. | |
Also as shown in Fig. 4, when the inorganic zeolite content was higher than M2 (25% by weight) as shown in M4 and M6, the particles tended to agglomerate. Interphasal cracks and gaps formed as observed in the cross section of M6. Gas diffusion through the large free volume in the interphasal areas of Route (3) became dominant, so the molecular sieving effects on the selectivity for moisture observed in M1 and M2 disappeared. As shown in Fig. 7 and 8, the diffusion coefficients for H2O and CO2 both increased with the increasing content of zeolites regardless of the internal pore size of the zeolites. In this heavy inorganic loading region, as shown in Fig. 9–11, high gas diffusion coefficients resulted from leakage in the cracks or gaps that had generated high heat and moisture exchange data, but the membranes had lost their practical values for their low gas barrier property as shown in Fig. 8. This result agreed well with what observed previously by Liu et al. in the PVC/Na+-MMT composite membranes.2
 |
| Fig. 9 Temperature exchange effectiveness of PVA/zeolite composite membranes. | |
 |
| Fig. 10 Moisture exchange effectiveness of PVA/zeolite composite membranes. | |
 |
| Fig. 11 Enthalpy exchange effectiveness of PVA/zeolite composite membranes. | |
The molecule sieving effect has rendered sample M2, with 25% (by weight) of the 3A zeolite content, with high potential for practical applications in energy saving ventilation systems by breaking the trade-off between the energy recovering efficiency and gas barrier property. Its relatively tighter structure prevented the leakage of undesirable gases such as CO2 from the gaps or cracks as in M6 while its medium content of the 3A zeolite provided adequate 3 Å size internal pores to allow just the water molecules to pass. Compared to the pure PVA membrane, its CO2 transmission rate was decreased from 27 to 24 (×10−14 cm3 (STP) per cm per cm2 S Pa), while its temperature exchange effectiveness was improved from 56 to 60%, and enthalpy exchange effectiveness was improved from 33.3 to 35.1%. However, given the tolerance of the ventilation industry to certain levels of gas leakage, sample M4, with 40% (by weight) of the 3A zeolite content, as well as those of M3 and M4 containing 4A zeolite, may also be good choices for their higher energy exchange rates.
Mechanical properties of the membranes were also evaluated and shown in Fig. 12. Pure PVA (M0) with no 3A zeolite particles revealed relative high tensile strength and elongation at break.36 With the addition of 3A zeolite, the homogeneous structure of PVA was replaced by an inorganic structure. As expected, the mechanical properties of the membranes tended to decay. Very high zeolite content as in M6, had generated obvious gaps and cracks in the system to allow the leakage of undesirable gas phase components and made the membrane very fragile. These data also suggested that M2, or even M4, as reasonable choices with balanced total heat exchange and mechanical properties.
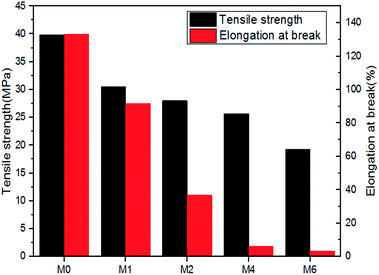 |
| Fig. 12 Tensile strength and elongation at break values of PVA/3A zeolite composite membranes. | |
The graphs of thermal gravimetric analyses (TGA) of the samples with different 3A zeolite content are shown in Fig. 13 (20 K min−1 heating rate, N2 atmosphere). The samples had different degrees of dehydration below 300 °C. M6 had the most dehydration due to the highest 3A zeolite content, M0 was just the opposite. Around 300 °C, samples began to degrade. With the increasing amount of the 3A zeolite, the slope of degradation decreased gradually. After 500 °C, the weight loss curves became flat when all the PVA decomposed and only the zeolite was left as residual.
 |
| Fig. 13 Thermogravimetric curves of PVA/3A zeolite composite membranes. | |
Conclusions
The addition of a zeolite had changed the gas molecule transferring mechanism by changing the structure of PVA membranes. When the zeolite/PVA weight ratio was lower than 25% by weight, as shown M1 and M2, small molecules, to avoid the tight PVA matrix, tended to diffuse through the free volume created by the pores inside the zeolitic particles. Strong molecular sieving effects relating to the pore sizes of zeolites were observed in the performance of the formed membranes. The addition of the nonporous zeolite barred the transfer of both CO2 and H2O, the addition of the 4A zeolite accelerated the transfer of both while the addition of the 3A zeolite accelerated the transfer of H2O and barred the transfer of CO2. When the zeolite/PVA weight ratio was greater than 40% by weight, small molecules tended to transfer through interphase gaps and cracks, as shown from M4 to M6, resulting in high total heat exchange efficiency but poor CO2 barring performance and mechanical properties.
The molecule sieving effect has rendered sample M2, with 25% (by weight) of the 3A zeolite content, with high potential for practical applications in energy saving ventilation systems by breaking the trade-off between the energy recovering efficiency and gas barrier property. Given the tolerance of the ventilation industry, PVA/zeolite composite membranes, containing 25–40% by weight of the 3A or 4A zeolitic content may have potential for application as total heat exchange membranes in energy recovered ventilation systems.
Acknowledgements
We are grateful for the financial supports from the Chinese Ministry of Science and Technology (No. 2014BAJ02B02), the Science and Technology Bureau of Ningbo (No. 2014B81004), the Chinese National Spark Program (No. 2014GA701029), the coherence innovation center of “Membrane separation and water treatment” of Zhejiang Province and the Zhejiang Preferred Postdoctoral Fund (No. BSH1402075).
Notes and references
- L. X. Xue, B. X. Liu, J. Chen and A. C. Shao, Structure and properties of total-heat exchange membranes for energy saving heat exchange ventilation processes, Adv. Mater. Res., 2012, 374, 568–571 Search PubMed
. - B. Liu, J. Chen, X. Du and L. X. Xue, Poly(vinylchloride)/montmorillonite composite membranes for total-heat recovery ventilation, J. Membr. Sci., 2013, 443, 83–92 CrossRef CAS
. - A. Mardiana-Idayu and S. B. Riffat, Review on heat recovery technologies for building applications, Renewable Sustainable Energy Rev., 2012, 16, 1241–1255 CrossRef
. - S. B. Riffat, A. P. Warren and R. A. Webb, Rotary heat pumps driven by natural gas, Heat Recovery Syst. CHP, 1995, 15, 545–554 CrossRef CAS
. - W. Anthapanichakoon and A. Prawarnpit, New simple mathematical model of a honeycomb rotary absorption-type dehumidifier, Chem. Eng. J., 2002, 86, 11–15 CrossRef
. - Y. Hamamoto and J. Okajima, Analysis of the performance in rotary dehumidifier/humidifier and systems, in. Proceedings of the International Sorption Heat Pump Conference, Shanghai, 2002 Search PubMed
. - C. J. Simonson and R. W. Besant, Energy wheel effectiveness: part II-correlation, Int. J. Heat Mass Transfer, 1999, 42, 2171–2185 CrossRef CAS
. - B. R. Lamb, Plate heat exchangers—a low cost route to heat recovery, J. Heat Recovery Syst., 1982, 2, 247–255 CrossRef CAS
. - A. Vali, C. J. Simonson, R. W. Besant and G. Mahmood, Numerical model and effectiveness correlations for a run-around heat recovery system with combined counter and cross flow exchangers, Int. J. Heat Mass Transfer, 2009, 52, 5827–5840 CrossRef
. - R. M. Lazzarin and A. Gasparella, Technical and economical analysis of heat recovery in building ventilation systems, Appl. Therm. Eng., 1998, 18, 47–67 CrossRef
. - Y. H. Yau and M. Ahmadzadehtalatapeh, A review on the application of horizontal heat pipe heat exchangers in air conditioning systems in the tropics, Appl. Therm. Eng., 2010, 30, 77–84 CrossRef
. - J. C. Min, T. Hu and X. W. Liu, Evaluation of moisture diffusivities in various membranes, J. Membr. Sci., 2010, 357, 185–191 CrossRef CAS
. - J. C. Min, T. Hu and Y. Z. Song, Experimental and numerical investigations of moisture permeation through membranes, J. Membr. Sci., 2011, 367, 174–181 CrossRef CAS
. - J. C. Min and L. N. Wang, Coupled heat and mass transfer during moisture exchange across a membrane, J. Membr. Sci., 2013, 430, 150–157 CrossRef CAS
. - L. Z. Zhang, X. R. Zhang and Q. Z. Miao, Selective permeation of moisture and VOCs through polymer membranes used in total heat exchangers for indoor air ventilation, Indoor Air, 2012, 22, 321–330 CrossRef CAS PubMed
. - R. X. Yan, Water-soluble Polymer, Beijing, Chemistry Industry Press, 2002 Search PubMed
. - X. X. Zhou, The Manual of Practical Plastic Packaging Product, Beijing, Chinese light industry press, 2000 Search PubMed
. - G. Clarizia, C. Algieri and E. Drioli, Filler-polymer combination: a route to modify gas transport properties of a polymeric membrane, Polymer, 2004, 45, 5671–5681 CrossRef CAS
. - S. Khoonsap and S. Amnuaypanich, Mixed matrix membranes prepared from poly(vinyl alcohol) (PVA) incorporated with zeolite 4A-graft-poly(2-hydroxyethyl-methacrylate) (zeolite-g-PHEMA) for the pervaporation dehydration of water–acetone mixtures, J. Membr. Sci., 2011, 367, 182–189 CrossRef CAS
. - M. S. Rao, S. R. Kanatt and S. P. Chawla, Chitosan and guar gum composite films: preparation, physical, mechanical and antimicrobial properties, Carbohydr. Polym., 2010, 82, 1243–1247 CrossRef CAS
. - M. Shah, M. C. McCarthy and S. Sachdeva, Current status of metal–organic framework membranes for gas separations: promises and challenges, Ind. Eng. Chem. Res., 2012, 51, 2179–2199 CrossRef CAS
. - Z. Lai, G. Bonilla and I. Diaz, Microstructural Optimization of a Zeolite Membrane for Organic Vapour Separation, Science, 2003, 300, 456–460 CAS
. - Z. Gao, Y. Yue and W. Li, Zeolites, 1996, 16, 70 CrossRef
. - M. Matsukata and E. Kikuchi, Zeolitic membranes: synthesis, properties, and prospects, Bull. Chem. Soc. Jpn., 1997, 70, 2341–2356 CrossRef CAS
. - Y. S. Lin, I. Kumakiri and H. Alsyouri, Microporous inorganic membranes, Sep. Purif. Methods, 2002, 31, 229–379 CrossRef CAS
. - J. Caro and M. Noack, Zeolite membranes-recent developments and progress, Microporous Mesoporous Mater., 2008, 115, 215–233 CrossRef CAS
. - J. Caro, M. Noack and P. Kolsch, Zeolite membranes – state of their development and perspective, Microporous Mesoporous Mater., 2000, 38, 3–24 CrossRef CAS
. - X. D. Yu and A. L. Wang, Water vapor permeability of polyvinyl alcohol films, Desalination, 1987, 62, 293–297 CrossRef CAS
. - J. G. Wijmans and R. W. Baker, The solution-diffusion model: a review, J. Membr. Sci., 1995, 107, 1–21 CrossRef CAS
. - H. K. Lonsdale, U. Merten and R. I. Riley, Transport Properties of Cellulose Acetate Osmotic Membranes, J. Appl. Polym. Sci., 1965, 9, 1341–1362 CrossRef CAS
. - L. Z. Zhang, Fabrication of a lithium chloride solution based composite supported liquid membrane and its moisture permeation analysis, J. Membr. Sci., 2006, 276, 91–100 CrossRef CAS
. - C. L. Aitkan, L. W. Koros and D. R. Paul, Effect of structure and symmetry on gas transport properties of poly-sulfone, Macromolecules, 1992, 25, 3424–3434 CrossRef
. - M. Aguilar-Vega and D. R. Paul, Gas transport properties of polycarbonates and polysulfones with aromatic substitutions on the bisphenol connector group, J. Polym. Sci., Part B: Polym. Phys., 1993, 31, 1599–1610 CrossRef CAS
. - D. N. Theodorou, Diffusion in Polymers, Marcel Dekker, New York, 1996 Search PubMed
. - K. Zeng and Y. P. Bai, Improve the gas barrier property of PET film with montmorillonite by in situ interlayer polymerization, Mater. Lett., 2005, 59, 3348–3351 CrossRef
. - B. Sarkar, K. Sunitha and S. Sridhar, Bioethanol Dehydration through Polyvinyl alcohol (PVA) and 3A Zeolite Mixed Matrix Composite Pervaporation Membrane, J. Polym. Mater., 2013, 30, 131 CAS
.
|
This journal is © The Royal Society of Chemistry 2016 |