DOI:
10.1039/C6RA09651D
(Paper)
RSC Adv., 2016,
6, 59242-59249
Novel magnetic iron oxide nanoparticles coated with sulfonated asphaltene as crude oil spill collectors
Received
14th April 2016
, Accepted 13th June 2016
First published on 15th June 2016
Abstract
This work aims to apply modified asphaltene for capping of magnetite to form dispersed hydrophobic magnetic nanomaterials for environmental applications. In this respect, asphaltene was separated from heavy crude oil and functionalized by sulfonation in order to apply as a capping agent for magnetic materials to collect the heavy crude oil that was not easily collected or dispersed by using different oil spill control techniques. The coprecipitation method was used to prepare magnetite in the presence of sulfonated asphaltene as a capping agent. The magnetite nanomaterials were confirmed by thermogravimetric analysis, X-ray powder diffraction, transmittance electron microscopy and a vibrating sample magnetometer. Finally, sulfonated asphaltene magnetite nanomaterials were applied at different concentrations to collect a heavy crude oil spill after application of an external magnetic field as novel magnetic materials. They achieved remarkable oil spill collection efficiency with a reduction in the overall cost of the materials when compared with available techniques.
1. Introduction
Oil spills produced from tanker collisions, platform and ship accidents caused several marine environmental damages in addition to economic resources losses.1–3 Immediate and long-term damage to aquatic, coastal, and land habitats, as well as humans are resulting from crude oil spill accidents all over the world. Several tools have been widely used to control oil spill pollution including mechanical tools (booms and skimmers), bioremediation and chemical tools.4–8 The oil spill chemicals are the most common response to an oil spill and they can be used to disperse the oil into a water column to be biodegraded with time. These chemicals may also have adverse effects and may increase environmental damages.9–11 Oil sorbers are an alternative tool to collect oil spills by fast oil collection over water and have a high oil uptake rate in a short time although they cannot be easily reused for high viscous heavy crude oils.12 It was reported that the combination of all methods based on mechanical and chemical tools used to control an oil spill has limited applications to control the heavy crude oil spills.12 Recently, an oil spill cleanup chemical was modified by using super hydrophobic materials having specific super hydrophobic surface properties.13–15 The literature and review surveys on the oil spill tools showed that the tools still need to be improved or new applicable and cheap tools are required to be more effective tools for heavy crude oils. It is possible to use a novel technique that leads to highly efficient and inexpensive oil cleanup as shown in the present work.
Magnetic fluids are advanced chemicals can be used to collect oil spills by applying an external magnetic field.16–18 The hydrophobic magnetic particles having strong magnetic properties are the main target to apply these materials to collect the oil spill.19 The disadvantages of these materials are caused from low dispersability of magnetic nanomaterials and collection of magnetic materials to reuse.20 In previous works,21–24 the hydrophobic magnetic nanomaterials were prepared and applied as a powder through spreading on the oil spill surface without applying toxic solvents or capping agents. In the present work, our aims are directed to separate asphaltene from heavy crude oil and modify its chemical structure in order to apply it as capping agents for magnetic nanoparticles. The formation of sulfonated asphaltene (SAS) is believed to serve as the nanoreactor to apply as a soft template because of its hydrophilic group (–SO3H) and the hydrophobic polycondensed aromatic, cycloparaffin and linear alkyl group assisting the formation of micelles and stabilizing colloids in an aqueous solution. It is expected that the particle size of magnetite can be controlled by the micelle “reactor” sizes.25 The advantage of this method is its offer to use cheap materials produced from crude oils to increase the dispersion of magnetic materials into crude oil. Moreover, it is expected that the performance of prepared magnetic nano-powder materials to collect the heavy crude oil will increase due to superior surface properties of the nanomaterials. The separation and reuse of these materials to collect the oil spill are another objective of the present work.
2. Experimental
2.1. Materials
The reagents used to prepare magnetite are based on ferric chloride hexahydrate (FeCl3·6H2O), sodium sulfite (Na2SO3), potassium iodide (KI), and ammonium hydroxide (25%) and were purchased from Aldrich Chemical Co. Asphaltene was precipitated from Arabian heavy crude oil produced from Ras Gara oilfield, Ras Tannora, Saudi Arabia by dilution of crude oil and precipitation of asphaltene using n-heptane. Oleum sulfuric acid, used as a reagent for sulfonation of the asphaltene fraction, was also produced by Aldrich Chemical Co. Solvents such as hexane, heptane, ethanol, acetone and dichloromethane were used without further purification. Sea water was collected from Western Arabian Gulf at the Saudi coast.
Asphaltene was sulfonated using a modified reported method26 as follows: 10 g of asphaltene was dissolved in 100 mL of dichloromethane and cooled to 10 °C using an ice bath. Oleum (10.5 g) was added dropwise under vigorous stirring for a period of 2 h. The reaction mixture was stirred for another 3 h at room temperature and extracted three times with n-hexane. The remaining solid was washed several times with acetone to produce sulfonated asphaltene (SAS).
2.2. Preparation of NIPAm copolymer nanogels
The procedure for capping the magnetite with SAS is summarized as follows: FeCl3·6H2O (7.21 g) was dissolved in distilled water (50 mL) and added to an aqueous solution of Na2SO3 (1.12 g dissolved in 15 mL of water). The reaction temperature was raised up to 50 °C under nitrogen atmosphere. The SAS solution (2.3 g dissolvent in 20 mL ethanol) was added dropwise at the same time with an ammonia solution (50 mL) to the reaction mixture during a period of 1 h until the pH of solution reached 10. The reaction mixture was stirred for another 3 h under nitrogen atmosphere. The black colloids were separated by ultracentrifugation of the solution at 8000 rpm and washed with water and ethanol several times. The precipitate was dried in air to produce magnetite nanoparticles capped with SAS which are designated as SAS-MPNS.
The same procedure was repeated to prepare magnetite capped with SAS except KI was used as a reagent instead of Na2SO3.27 The dissolved weights of FeCl3·6H2O, KI and SAS are 5, 1.02 and 1.3 g, respectively. The produced magnetite can be designated as SAS-MPKI.
2.3. Characterization
The chemical structure of the prepared magnetite nanomaterials was verified by using Fourier transform infrared analysis (FTIR; model Nexus 6700 FTIR).
The crystal lattice structure and diffraction pattern of magnetite were analyzed by X-ray powder diffraction (XRD; BDX-3300 diffractometer using CuKα radiation of wavelength, λ = 1.5406 Å).
The magnetite and SAS contents were determined from thermogravimetric analysis (TGA; Shimadzu, DSC-60 and heating the samples from room temperature to 800 °C under N2 atmosphere at a heating rate of 10 °C min−1).
The surface morphology of magnetite was confirmed by high resolution transmission electron microscopy (HR-TEM; JEOL JEM-2100F).
The particle diameter and dispersity index were analyzed by dynamic light scattering (DLS; Zetasizer 3000HS; Malvern Instruments, Malvern, U.K.) with a 633 nm He–Ne laser using ethanol/water/(4/1) as the solvent. Zeta potentials of magnetite suspensions were evaluated at 25 °C using a zeta potential analyzer.
Contact angle measurements between magnetite dispersions in ethanol and crude oil were determined using the sessile drop technique drop shape analyser (model DSA-100). The crude oil layer with a thickness of 1 cm was spread on a glass plate and the drop of dispersed magnetite ethanol solution contacted the crude oil layer to measure the advancing and receding contact angles.
The magnetic parameters of the prepared magnetite were determined at room temperature using vibrating sample magnetometer (VSM; LDJ9600 in a field of 20
000 Oe).
2.4. Application of magnetite as a crude oil spill collector
The Arabian heavy crude oil (1 mL) was stirred over 250 mL of sea water and the magnetic powder was spread on the oil surface with different magnetite to oil ratios ranging from 1
:
25 to 1
:
1 after mixing with a 1 cm deep vortex obtained by slow stirring for 1 minute. The dispersed crude oil spill stood for 5 min and a permanent magnet of Nd–Fe–B (4300 Gauss) was applied to collect the crude oil layer from the water surfaces. The remained oil volume was extracted by chloroform and determined after evaporation of chloroform using a rotary evaporator. The efficiency for collection of crude oil can be calculated as: CE (%) = (volume of removed oil/volume of original spill) × 100%. The magnetite powders were separated by dilution of the separated oil and using an external magnetic field and reused three times.
3. Results and discussions
Iron oxide nanomaterials such as maghemite and magnetite have been widely used for environmental purposes due to their superior magnetic properties and particle size distribution which were altered by methods of preparation. Different methods were used to prepare magnetic nanoparticles such as coprecipitation, hydrothermal, thermal, sonochemical and sol–gel.28–30 The disadvantages of these methods are based on agglomeration and particle size distribution and chemical stability to various environments.28–30 In this respect, the objective of the present work is based on using new capping agents derived from asphaltene as a natural surfactant to prepare and apply a monodisperse magnetic material as an oil spill collector. Asphaltene was selected as a capping agent due to its strong ability to form colloidal particles in crude oil using different interactions such as hydrogen bond forces, aromatic π–π* stacking forces, polarity induction forces, and electrostatic attractions between the molecules.31 Accordingly, the application of modified asphaltene as capping agents for magnetite nanoparticles will increase their dispersability in crude oil rather than water and will facilitate the collection of crude oil from the surface of water. It is necessary to modify the chemical structure of asphaltene to easily disperse in the polar solvent water or ethanol that is used to prepare iron oxide nanoparticles by the coprecipitation method. The asphaltene molecules which consist of a fused polycondensed aromatic and naphthenic unit core can be modified by the sulfonation reaction.26,32
The chemical structure of sulfonated asphaltene was confirmed from the FTIR spectrum that is represented in Fig. 1a. It was noticed that the spectrum of asphaltene (Fig. 1a) is similar to data reported previously for asphaltene32 except the appearance of a new band observed at 1074 cm−1 (S
O stretching vibration) which confirms the sulfonation of asphaltene. The presence of aromatic groups was confirmed by the appearance of bands at 3095, 1602 and 860, 800, 750 cm−1 which was attributed to
CH stretching, C
C stretching and aromatic out of plane bending vibrations, respectively. The presence of CH2 cyclic and CH3 aliphatic is confirmed from bands at 2950, 2850, 1450 and 1375 cm−1 that referred to aliphatic CH stretching and bending vibrations. The polar functionalities of asphaltene were confirmed by the appearance of stretching vibrations of –OH and/or –NH at 3450 cm−1; and stretching vibrations of C
O or C
N in heterocyclic rings at 17
010 cm−1.
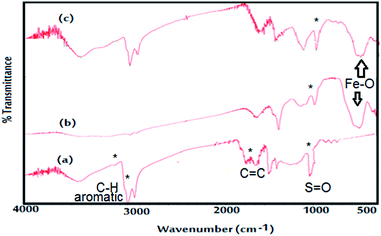 |
| Fig. 1 FTIR spectra of (a) SAS, (b) SAS-MPKI and (c) SAS-MPNS. | |
The magnetite can be formed under oxidizing and reducing conditions using KI or Na2SO3 as illustrated in eqn (1) and (2):21,27
|
3Fe3+ + I− → 2Fe3+ + Fe2+ + ½I2
| (1) |
|
6Fe3+ + SO32− + ½O2 → 4Fe3+ + 2Fe2+ + SO42−
| (2) |
Ferric chloride was oxidized to ferrous and ferric cations (Fe2+ and Fe3+) which hydrolyzed in basic conditions to form magnetite. The mechanism for preparation and capping of magnetite nanoparticles is illustrated in Scheme 1.
 |
| Scheme 1 Synthesis of SAS magnetite nanomaterials. | |
In this respect, the Fe2+ and Fe3+ that were produced from the reaction of ferric chloride with KI or Na2SO3 interacted with sulfonated asphaltene as described in the experimental section. The capped iron cations were hydrolyzed with ammonia solution at pH 10 to form capped magnetite iron oxide. Magnetite can interact with sulfonated asphaltene by complexation with sulfonate groups and hetero nitrogen, oxygen or sulfur groups of asphaltene moieties. It was expected that sulfonated asphaltene can form a physical cap with magnetite by hydrogen bond forces, polarity induction forces, and electrostatic attractions between the magnetite and sulfonated asphaltene molecules.31
3.1. Chemical structure of magnetite nanomaterials
The chemical structure of iron oxide nanoparticles capped with sulfonated asphaltene can be elucidated from the FTIR spectra (Fig. 1a–c). The appearance of a new band at 580 cm−1 (Fe–O stretching) without additional new bands indicates the formation of pure magnetite nanoparticles without other iron oxides.33 This data confirm the stability of magnetite against environmental oxidation.33 The presence of sulfonated asphaltene was confirmed by the appearance of broad bands at 3, 450, 1630 and 1070 cm−1 which are attributed to OH, COOH and S
O stretching which elucidated the functionalization of magnetite nanoparticles with the sulfonate, carboxylic or hydroxyl groups of sulfonated asphaltene as illustrated in Scheme 1. The low intensity of SAS of magnetite prepared by the KI method (Fig. 1b) indicates that the concentration of the magnetite content was increased more than SAS. The higher intensity of the band at 3450 cm−1 (Fig. 1c) indicated an increase of the hydroxyl group content in the case of the magnetite of SAS-MPNS more than that of SAS-MPKI. This observation confirms that the magnetite surfaces were modified with hydroxyl groups in the case of magnetite of SAS-MPNS more than that of SAS-MPKI.21,27 The shift of the C
O and S
O vibration (symmetric stretching) to a broad intense band confirms the chemisorption between Fe–O and sulfonated asphaltene.33 It was also noticed that the intensity of sulfonated asphaltene bands was highly increased for magnetite formed from the reaction of FeCl3 with Na2SO3 (Fig. 1b and c) more than that formed from the reaction with the KI reagent. This observation confirms that more magnetite nanoparticles were capped in the presence of KI than that of Na2SO3. This means that KI is more effective in the redox reaction that produces ferric and ferrous cations than Na2SO3 as illustrated in eqn (1) and (2) which was confirmed from the high yield of magnetite that was produced by using KI in previous works.21,22,27
XRD diffractograms of magnetite nanoparticles capped with sulfonated asphaltene are illustrated in Fig. 2a and b. The diffraction patterns confirm the presence of magnetite without contamination of other iron oxides. There are characteristic peaks at 2θ = 30.17°, 35.53°, 43.16°, 53.51°, 57.27°, 62.77°, and 73.79° confirming peaks for Fe3O4 marked by their indices (220), (311), (400), (422), (511), (440), and (622) as confirmed with the database in the Joint Committee on Powder Diffraction Standards (JCPDS) file (PDF no. 65-3107). The presence of sulfonated asphaltene was elucidated from the appearance of broad diffraction peaks at about 2θ = 20° that confirms the capping of magnetite with amorphous sulfonated asphaltene without distortion of magnetite crystals due to functionalization of magnetite with reactive functional groups of sulfonated asphaltene.34 It can also be observed that the presence of a broad intense peak at 35.53° confirms the formation of magnetite nanospheres due to capping with sulfonated asphaltene.35
 |
| Fig. 2 XRD of (a) SAS-MPNS and (b) SAS-MPKI. | |
The concentration of magnetite encapsulated into asphaltene colloid nanoparticles can be determined from TGA as illustrated from thermograms represented in Fig. 3a and b. It was previously reported36 that magnetite lost approximately 8% (wt%) from its weight due to the presence of bound water and functionalization of magnetite surfaces with hydroxyl groups. In the present work, it was found from Fig. 3a and b that magnetite nanoparticles lost 4 and 8 wt%, at a temperature ranging from 80 to 150 °C, from their initial weights when KI and Na2SO3 were used to form magnetite. This observation indicates that more hydroxyl groups were functionalized on the magnetite surfaces when Na2SO3 was used to form magnetite as confirmed from the FTIR spectrum (Fig. 1b and c). Moreover, the surface of magnetite can be easily encapsulated with sulfonated asphaltene when KI was used to form magnetite nanoparticles. The asphaltene begins to degrade at 300 °C (Fig. 3a and b) followed by a significant weight loss between 450 °C and 550 °C. The thermal degradation and pyrolysis of asphaltene are similar to the data reported for modification of asphaltene.37 The magnetite content, determined at 800 °C, are 68.5 and 48% when KI and Na2SO3 reagents are used to form magnetite nanoparticles, respectively. This means that the magnetite content increased inside sulfonated asphaltene when their surfaces contain less hydroxyl groups on their surfaces. These data confirmed from the results obtained from FTIR analysis (Fig. 1a–c).
 |
| Fig. 3 TGA thermograms of (a) SAS-MPKI and (b) SAS-MPNS. | |
3.2. Morphology and magnetic characteristics of magnetite nanomaterials
The surface morphology of magnetite coated with sulfonated asphaltene can be determined from TEM micrographs that are represented in Fig. 4a and b. It was found that magnetite nanoparticles formed as nano-chains as represented in Fig. 4a. The TEM micrographs showed the formation of iron oxide nanoparticles that have an irregular spherical shape with an average diameter of 10.8 ± 3.0 nm and they were arranged in clusters because of their magnetic nature.38 The clusters increased for magnetite prepared in the presence of Na2SO3 which was attributed to the hydroxyl group content at the surface of magnetite as proven from the TGA data (Fig. 2a and b). It was previously reported that the morphology of nanoparticles was affected by the presence of functional groups at their surfaces.22,27 The high resolution TEM micrographs at the top of Fig. 4a and b confirm the formation of uniformly arranged planes inside the magnetite crystallite as black dots and suggested an inverse spinel structure of the magnetite crystal lattice.38 The formation of clusters can also be referred to by the formation of magnetite inside SAS micelles which was stabilized by the –SO3– group, while the absence of long alkyl hydrophobic chains and the presence of polycondensed aromatic cannot protect the agglomeration of the magnetite particles.39
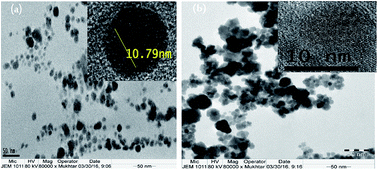 |
| Fig. 4 TEM micrographs of (a) SAS-MPKI and (b) SAS-MPNS. | |
The particle size diameter and their distribution were determined from DLS measurements as represented in Fig. 5a–c. The data showed that the sulfonated asphaltene was dispersed in water as a colloid with an average diameter of 1075 nm and polydispersity index (PDI) of 0.377. The magnetites coated with sulfonated asphaltene have considerably larger diameters than that determined from TEM data which referred to the inclusion of regions of agglomerations. It was also noticed that the PDI increased with the formation of magnetite nanoparticles using Na2SO3 instead of the KI reagent. Moreover, the size of agglomerates decreased for magnetite capped with sulfonated asphaltene in the presence of KI as the reagent to form magnetite nanoparticles. It was previously reported that poor control of shape and particle size distribution are drawbacks of magnetite nanoparticles prepared using the aqueous coprecipitation method.40 The distribution width is still in an acceptable range in this work when compared with reported coprecipitation methods. The zeta potential measurements represented in Fig. 6a and b confirm the more negative values for the zeta potential of magnetite prepared in the presence of KI than that prepared in the presence of Na2SO3. The zeta potential of magnetite prepared in the presence of KI and in the presence of Na2SO3 are −7.37 and −20.26 respectively which confirms the high content of SAS-MPNS more than that prepared in the presence of KI as confirmed from FTIR and TGA data.
 |
| Fig. 5 DLS data of (a) SAS-MPNS, (b) SAS-MPKI and (c) SAS at 25 °C. | |
 |
| Fig. 6 Zeta potential of data of (a) SAS-MPKI and (b) SAS-MPNS at 25 °C. | |
Magnetic properties of iron oxide nanoparticles are important parameters that affect their environment applications. In this respect, there are three different parameters based on saturation magnetization (Ms), coercive force (Hc) and magnetic remanence (Mr) used to determine the magnetic properties of particles. The Ms, Hc and Mr reflect the magnetizability of magnetic materials, the ability of magnetic particles to retain magnetization and the remaining magnetization of magnetic materials after removing an external magnetic field. These parameters were determined from the magnetic hysteresis loop which characterizes the response ability (magnetization, M) of magnetic materials to an external magnetic field (denoted by the magnetic field strength, H). In this work, the magnetic hysteresis loop of SAS-MPs was measured at room temperature by a vibrating sampling magnetometer (VSM) and represented in Fig. 7a and b. Moreover, the Ms, Hc, Mr and squareness ratio (Mr/Ms) values of SAS-MPs were determined and listed in Table 1. The data represented in Fig. 7 and Table 1 showed that SAS-MPs have low Ms values compared to bulk magnetite values that ranged from 75 to 92 emu g−1.41 The magnetization curve confirms the low values of Hc, Mr and the squareness ratio (Mr/Ms) which indicates the absence of remanence or coercivity at room temperature, and suggests the superparamagnetic characteristics of the prepared samples. It can also be observed that the Ms values of SAS-MPs were decreased by increasing the content of SAS capped on Fe3O4 nanoparticles. This means that the higher concentration of magnetite increases the Ms value and reduces the interparticle spacing due to the presence of more intense interactions between particles.42 It was also reported that the magnetic materials have superparamagnetic behaviour when their size decreases below the critical value, generally around 20 nm.42 In this work, although the formed magnetite nanoclusters have sizes above 20 nm as determined from DLS measurements (Fig. 5) the particles showed superparamagnetic rather than ferromagnetic characteristics. This can be attributed to the formation of nano-chain clusters that resist the transition from superparamagnetism to ferromagnetism.43,44 The superparamagnetism of the prepared SAS-MPs supports the application of these materials as oil spill collectors after dispersion in an oil layer followed by application of an external magnetic field.
 |
| Fig. 7 VSM hysteresis loop of (a) SAS-MPKI and (b) SAS-MPNS. | |
Table 1 Magnetic parameters for SAS magnetite nanoparticles tested at room temperature
Sample |
Ms (emu g−1) |
Mr (emu g−1) |
Mr/Ms |
Hc (Oersted; Oe) |
SAS-MPKI |
58.3 |
0.0085 |
0.00014 |
3.469 |
SAS-MPNS |
43.2 |
0.0170 |
0.00039 |
5.17 |
3.3. Application of magnetite nanomaterials as oil spill collectors
It is necessary to evaluate the dispersability of the magnetite nanomaterials capped with SAS-MP in crude oil without dispersion or precipitation in sea water to apply the magnetic materials as oil spill collectors.45 The advancing and preceding contact angle were determined between dispersed 1 wt% of SAS-MPNS or SAS-MPKI in ethanol and crude oil on the crude oil as illustrated in the experimental section and represented in Fig. 8 and Table 2. The prepared SAS-MPs were not dispersed in water and well dispersed in ethanol, toluene and non-polar solvent. Ethanol was selected as the polar solvent (like water), which has low toxicity compared to a toxic non-polar solvent, to investigate the wetting characteristics of SAS-MPs between crude oil and a polar ethanol solvent. The photo of the contact angle of ethanol SAS-MPNS and SAS-MPKI dispersion on a crude oil layer and blank are illustrated in Fig. 8. The blank measurement was determined between ethanol without SAS-MP and crude oil. The data confirm that the SAS-MPKI molecules were highly dispersed in crude oil more than SAS-MPNS although the hydrophobicity of crude oil was increased in the presence of SAS-MPNS. The low value of the contact angle between crude oil and ethanol dispersion of 18.5° confirms the good dispersability of SAS-MPNS and SAS-MPKI nanomaterials in crude oil more than the dispersion in a polar solvent or sea water and reflects the hydrophobicity of SAS-MPNS and SAS-MPKI. The stability of the contact angle over time (Fig. 8) elucidates the good surface properties and surface activity of SAS-MPNS and SAS-MPKI nanomaterials.
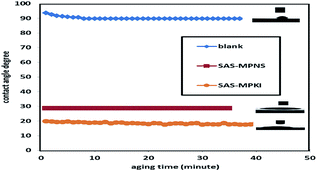 |
| Fig. 8 Contact angle measurements of 1 wt% of dispersed SAS-MP ethanol solution and crude oil. | |
Table 2 Contact angle and efficiency data of SAS-MPs as oil spill collectors for Arabian heavy crude oil magnetic parameters at room temperature
Sample |
Contact angle of crude oil dispersion |
Contact angle of ethanol dispersion |
CE% at different ratios |
1 |
10 |
25 |
50 |
SAS-MPKI |
98 ± 4 |
18.5 ± 3 |
92 |
90 |
88 |
80 |
SAS-MPNS |
110 ± 3 |
29.3 ± 2 |
98 |
94 |
90 |
85 |
The crude oil collection efficiency (CE%) of SAS-MPNS and SAS-MPKI as oil spill collectors for Arabian heavy crude oil at different magnetic materials to crude oil ratios (1
:
1 to 1
:
50) was determined and listed in Table 2. The photos of oil spill collection by applying an external magnetic field are represented in Fig. 9. The data listed in Table 2 indicate that the best magnetite to crude oil ratio of 1
:
25 succeeded to remove 90% of crude oil, which means that each gram of SAS-MPNS and SAS-MPKI is able to remove around 22.5 g of the heavy crude oil from the water. This value is very high when compared with the maghemite composite which collects 10 g of crude oil per g of magnetic materials.22–24,45 These results indicate also that the increase of the magnetite oil ratio to 1
:
1 was not effective to collect crude oil due to aggregation of magnetite clusters that affect the magnetic attraction between the magnetic particles and the magnet. It was also found that the presence of asphaltene and magnetite in SAS-MPNS and SAS-MPKI increases the removal efficiency of the oil from the water due to the larger dispersion of the prepared materials in heavy crude oil. The high dispersion of magnetite and the larger magnetic attraction of SAS-MPNS and SAS-MPKI are responsible for the formation of a strongly bonded network, which acts as a trap for crude oil. Therefore, the oil removal collection efficiency can be improved by increasing the hydrophobicity of magnetite nanomaterials.
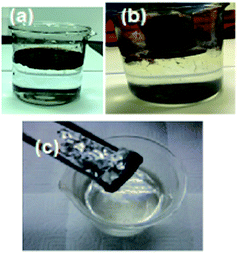 |
| Fig. 9 Oil collector photos of SAS-MPKI (a) blank crude oil, (b) application of magnetic material and (c) collection of oil by an external magnetic field. | |
Finally the feasibility study showed that the cost for preparing 1 kg of magnetic materials based on SAS-MPNS was 2 US $ including the material and production costs (25% from material cost). Moreover, the yield on a semi-pilot scale was 95%. This mean that the cost for collection of 1 crude oil barrel was 12 US $ based on CE% of the effective magnetic material crude oil ratio (25 as listed in Table 2). Moreover, depending on the thickness of crude oil, the total volume of crude oil can be estimated, and it was found that 1 kg of magnetic materials can recover 60 m3 of crude oil spill with a thickness of 1 cm. The application of the magnetic material to collect the crude oil spill has great advantages over other methods to clean-up the oil spill in which the crude oil was collected and reused without loss in water through the use of oil spill dispersants that pollute the water. Moreover, the recovery of magnetic materials and using a predominant external magnet requires more research work to apply these materials in offshore and onshore oil spill fields.
4. Conclusions and outlook
Hydrophobic magnetic nanomaterials based on magnetite nanoparticles capped with sulfonated asphaltene are natural and cheap materials proposed to apply as oil spill collectors for Arabian heavy crude oil. New stable colloid magnetite nanoparticles capped with SAS have been prepared at low temperature using one salt of ferric chloride as the precursor. The chain magnetite nanoparticles have been observed and were responsible for the magnetic characteristics of magnetite capped with SAS. The main objective of this work was achieved because sulfonated asphaltene/magnetite nanomaterials were able to collect heavy crude oil with good magnetic properties. The TEM and DLS results showed the aggregation of magnetite in chain clusters in asphaltene molecules. The prepared SAS magnetite nanomaterials presented high dispersion in crude oil more so than water, allowing their easy dispersion and flotation in crude oil without precipitation in sea water. The prepared materials achieved a high crude oil collection efficiency; each gram of the nanomaterial is able to collect around 22.5 g of the crude oil from the sea water surface. It was also found that the presence of asphaltene and magnetite in SAS-MPNS and SAS-MPKI increases the removal efficiency of the oil from the water due to the larger dispersion of the prepared materials in heavy crude oil. These materials have great wetting characteristics in crude oil, making them able to alter the wettability of reservoir rocks to be useful in the future to apply as enhanced oil recovery oilfield chemicals. Therefore, the prepared SAS magnetite nanomaterials as cheap available renewable resources can be applied to collect the crude oil spill without dispersion of the oil spill in water.
Acknowledgements
This project was supported by King Saud University, Deanship of Scientific Research, Research Chair.
Notes and references
- P. Burgherr, J. Hazard. Mater., 2007, 140, 245–256 CrossRef CAS PubMed.
- P. T. Anastas, C. Sonich-Mullin and B. Fried, Environ. Sci. Technol., 2010, 44, 9250–9251 CrossRef CAS PubMed.
- P. D. Boehm and D. S. Page, Exposure elements in oil spill risk and natural resource damage assessments: a review, Hum. Ecol. Risk Assess., 2007, 13, 418–448 CrossRef CAS.
- J. Whitfield, Nature, 2003, 422, 464–466 CrossRef CAS PubMed.
- M. Radetic, V. Ilic, D. Radojevic, R. Miladinovic and D. Jovancic, Chemosphere, 2008, 70, 525–530 CrossRef CAS PubMed.
- C. Teas, S. Kalligeros, F. Zanikos, S. Stournas, E. Lois and G. Anastopoulos, Desalination, 2001, 140, 259–264 CrossRef CAS.
- R. M. J. Atlas, J. Chem. Technol. Biotechnol., 2007, 52, 149–156 CrossRef.
- M. Fingas, Chem. Ind., 1995, 24, 1005–1008 Search PubMed.
- S. S. Cameotra and R. S. Makkar, Pure Appl. Chem., 2010, 82, 97–116 CrossRef CAS.
- H. Chapman, K. Purnell, R. J. Law and M. F. Kirby, The use of chemical dispersants to combat oil spills at sea: a review of practice and research needs in Europe, Mar. Pollut. Bull., 2007, 54, 827–838 CrossRef CAS PubMed.
- C. M. Couillard, K. Lee, B. Legare and T. L. King, Environ. Toxicol. Chem., 2005, 24, 1496–1504 CrossRef CAS PubMed.
- F. L. Franklin and R. Lloyd, Oil Chem. Pollut., 1987, 3, 37–52 CrossRef.
- N. Zhao, F. Shi, Z. Q. Wang and X. Zhang, Langmuir, 2005, 21, 4713–4716 CrossRef CAS PubMed.
- Y. G. Jiang, Z. Q. Wang, X. Yu, X. F. Shi, H. P. Xu and X. Zhang, Langmuir, 2005, 21, 1986–1990 CrossRef CAS PubMed.
- L. Zhang, M. Zheng, X. K. Liu and J. Q. Sun, Langmuir, 2011, 27, 1346–1352 CrossRef CAS PubMed.
- C. Mengjiao, G. Yongfeng, G. Xianpeng, S. Zhaoyuan, C. Jian-feng and S. Feng, Langmuir, 2011, 27, 7371–7375 CrossRef PubMed.
- C. Chun and J. Park, J. Environ. Eng., 2001, 127, 443–449 CrossRef CAS.
- J. D. Orbell, L. Godhino, W. S. Bigger, T. M. Nguyen and L. M. Ngeh, J. Chem. Educ., 1997, 74, 1446–1448 CrossRef CAS.
- L. Yuan, C. Tao, W. Cuichen, Q. Liping, H. Rong, L. Juan, C. Sena, Z. Liqin, C. Cheng, Z. Guizhi, Y. Mingxu, Z. Tao and T. Weihong, J. Am. Chem. Soc., 2014, 136, 12552–12555 CrossRef PubMed.
- E. Berkowitz and J. L. Walter, J. Magn. Magn. Mater., 1983, 39, 75–78 CrossRef.
- A. M. Atta, O. E. El-Azabawy and H. S. Ismail, Corros. Sci., 2011, 53, 1680–1689 CrossRef CAS.
- A. M. Atta, H. A. Al-Lohedan and S. A. Al-Hussain, Molecules, 2014, 19, 11263–11278 CrossRef PubMed.
- A. M. Atta, H. A. Al-Lohedan and S. A. Al-Hussain, Digest Journal of Nanomaterials and Biostructures, 2015, 10, 745–758 Search PubMed.
- A. M. Atta, H. A. Al-Lohedan and S. A. Al-Hussain, Int. J. Mol. Sci., 2015, 16, 6911–6931 CrossRef CAS PubMed.
- P. J. Kinlen, J. Liu, Y. Ding, C. R. Graham and E. E. Remsen, Macromolecules, 1998, 31, 1735–1744 CrossRef CAS.
- M. A. Francisco, M. Siskin, S. R. Kelemen, D. J. Moser, J. S. Szobota, K. E. Edwards and J. S. Lyng, Energy Fuels, 2010, 24, 5038–5047 CrossRef CAS.
- A. M. Atta and A. K. F. Dyab, Coated magnetite nanoparticles, method for the preparation thereof and their uses, EP 2804186, 2014.
- A.-H. Lu, E. L. Salabas and F. Schüth, Angew. Chem., Int. Ed., 2007, 46, 1222–1244 CrossRef CAS PubMed.
- N. A. Frey, S. Peng, K. Cheng and S. Sun, Chem. Soc. Rev., 2009, 38, 2532–2542 RSC.
- O. Veiseh, J. W. Gunn and M. Zhang, Adv. Drug Delivery Rev., 2010, 62, 284–304 CrossRef CAS PubMed.
- M. A. Anisimov, I. K. Yudin, V. Nikitin, G. Nikolaenko, A. Chernoutsan, H. Toulhoat, D. Frot and Y. Briolant, J. Phys. Chem., 1995, 99, 9576–9580 CrossRef CAS.
- S. Chiaberge, G. Guglielmetti, L. Montanari, M. Salvalaggio, L. Santolini, S. Spera and P. Cesti, Energy Fuels, 2009, 23, 4486–4495 CrossRef CAS.
- A. M. Atta, H. A. Al-Lohedan and S. A. Al-Hussain, Molecules, 2014, 19, 11263–11278 CrossRef PubMed.
- D. Larcher, G. Sudant, R. Patrice and J. M. Tarascon, Chem. Mater., 2003, 15, 3543–3551 CrossRef CAS.
- L. Shen, Y. Qiao, Y. Guon, S. Meng, G. Yang, M. Wu and J. Zhao, Ceram. Int., 2014, 40, 1519–1524 CrossRef CAS.
- A. M. Atta, H. A. Al-Lohedan and S. A. Al-Hussain, Int. J. Mol. Sci., 2015, 16, 6911–6931 CrossRef CAS PubMed.
- H. Wu and M. R. Kessler, RSC Adv., 2015, 5, 24264–24273 RSC.
- C. Schweiger, C. Pietzonka, J. Heverhagen and T. Kissel, Int. J. Pharm., 2011, 408, 130–137 CrossRef CAS PubMed.
- H. Hagerstrand, J. Bobacka, M. B. Hagerstrand, V. K. Iglic, M. Fosnaric and A. Iglic, Cell. Mol. Biol. Lett., 2001, 6, 161–165 CAS.
- W. W. Yu, J. C. Falkner, C. T. Yavuz and V. L. Colvin, Chem. Commun., 2004, 306–2307 CAS.
- J. M. D. Coey, A. H. Morrish and G. A. Sawatzky, J. Phys., 1971, 32, 271–273 Search PubMed.
- Y. F. Zhu, W. R. Zhao, H. R. Chen and J. Shi, J. Phys. Chem., 2007, 111, 5281–5285 CAS.
- P. Gambardella, A. Dallmeyer, K. Maiti, M. C. Malagoli, W. Eberhardt, K. Kern and C. Carbone, Nature, 2002, 416, 301–304 CrossRef CAS PubMed.
- H. Wang, Q.-W. Chen, L.-X. Sun, H.-P. Qi, X. Yang, S. Zhou and J. Xiong, Langmuir, 2009, 25, 7135–7139 CrossRef CAS PubMed.
- A. Varela, G. Oliveira, F. G. Souza, C. H. M. Rodrigues and M. A. S. Costa, Polym. Eng. Sci., 2013, 53, 44–51 Search PubMed.
|
This journal is © The Royal Society of Chemistry 2016 |
Click here to see how this site uses Cookies. View our privacy policy here.