DOI:
10.1039/C6RA09500C
(Paper)
RSC Adv., 2016,
6, 54322-54330
Adsorption of naphthalene onto high-surface-area nanoparticle loaded activated carbon by high performance liquid chromatography: response surface methodology, isotherm and kinetic study†
Received
12th April 2016
, Accepted 31st May 2016
First published on 1st June 2016
Abstract
Naphthalene removal from aqueous solution was investigated using zinc sulfide nanoparticle loaded activated carbon (ZnS-NPs-AC). The concentration of naphthalene was determined using high performance liquid chromatography (HPLC). The prepared ZnS-NPs-AC was characterized by X-ray diffraction (XRD), Fourier transform infrared spectroscopy (FT-IR), field emission scanning electron microscopy (FE-SEM), scanning electron microscopy (SEM), particle size distribution (PSD), transmission electron microscopy (TEM) and BET surface area. A four-factor central composite design (CCD) combined with response surface modeling (RSM) was employed for the maximization of naphthalene adsorption in the batch mode of operation. Four independent variables viz. pH (1.0–9.0), initial naphthalene concentration (5–45 mg L−1), adsorbent dose (0.005–0.025 g) and contact time (5–25 min) were coded in a quadratic model to predict the response. The ZnS-NPs-AC was found to have a Langmuir monolayer adsorption capacity of 142.68 mg g−1, while the pseudo-second-order kinetic model in combination with the Elovich equation described the said process (R2 > 0.998) successfully.
1. Introduction
Water pollution due to the presence of aromatic compounds coming out from various industries cause adverse health effects and its remedial action is highly recommended.1,2 Polycyclic aromatics hydrocarbons are chemical species with fused benzene rings and are toxic, hazardous, and highly carcinogen. They may generate tumors in some organisms.3–5 Naphthalene originates from natural and anthropogenic sources, while such a material is present in engine exhaust, industrial outlets, crude oil, urban run-off, domestic heating systems, incinerators and smoke emitted from natural sources viz. terrestrial coal deposits, volcanic eruptions and forest fires.6 The main sources of naphthalene entrance to water resources are atmospheric deposition, runoff from contaminated soils and deposition from sewage discharge. Naphthalene as a hydrophobic compound with high boiling and melting points and electrochemical stability has potential to accumulate in soils or water for a long time.7–10
A variety of treatment techniques including chemical oxidation, biodegradation, photo catalytic degradation and adsorption are efficient methods for the removal of organic compound from wastewater.11–15 Adsorption is proven to be one of the most attractive and effective techniques to remove trace organic contaminants from aqueous solutions in water treatment.6,16,17
Carbon-based nano-materials having unique π-electronic structure is an excellent adsorbent for the removal of aromatic compounds.18 Loading of appropriate nanomaterials over the activated carbon causes enhancement in reactive sites and adsorption capacity of pollutants. Composite nanoparticles such as zinc sulfide loaded on activated carbon (ZnS-NP-AC) is a promising material with two soft reactive atoms in cooperation with the AC reactive center and functional groups which improve the diversity and capacity to remove organic and inorganic pollutants.19
Recently, several types of experimental design methods are employed in multivariable chemical process optimization to understand the interactions among the process variables. This method requires less number of experiments and minimizes time, as well as cost.20–27
Response surface methodology (RSM) as collective of experimental design techniques is conventional and more useful to avoid experimental time. This assembly enable the researchers for simultaneous evaluation of the effects of several factors to achieve optimum conditions for desirable responses with limited number of experiments24,27 The RSM simultaneously find the optimum response and give information to realize how the response changes in a given direction by modulating the design variables. RSM classifications like central composite design (CCD) and the Box–Behnken design (BBD)22 benefit from advantage like extensive reduction of number of experimental trials needed to evaluate multiple parameters and their interactions. Therefore, it is less laborious and time-consuming than other methodologies.
The aim of the present study was to investigate the effects of pH, initial naphthalene concentration, adsorbent doses and contact time on the adsorption efficiency of zinc sulfide nanoparticles loaded activated carbon. Optimized conditions were determined using the statistical experimental design method. The first step was to approximate the adsorption efficiency in terms of experimental variables by considering five levels of the independent variables using central composite design (CCD). This statistical design was used to determine the quantitative relationship between the response and the levels of the experimental factors. The kinetic data were analyzed by pseudo-first and pseudo-second order, intraparticle diffusion and Elovich models. Equilibrium isotherm data were fitted to Langmuir, Freundlich, Temkin, Dubinin–Radushkevich (D–R) and Harkins–Jura (H–J) models and constants of isotherm equations were determined.
2. Experimental
2.1. Materials
All chemicals were used as received without further decontamination. Hydrochloric acid (HCl), sodium hydroxide (NaOH), zinc acetate dehydrate (Zn(CH3COO)2·2H2O), acetic acid (CH3COOH) and activated carbon were purchased from Merck company. Thioacetamide (CH3CSNH2) was purchased from Sigma-Aldrich Company. Here, zinc acetate and thioacetamide were used as the source of Zn2+ and S2− ions, respectively, whereas acetic acid was employed as a complexing agent for Zn2+ ions and adjustment of pH. Activated carbon was used as a support material for ZnS-NPs.
2.2. Instrumentation and characterization
The pH was measured using pH/ion meter (model-686, Metrohm, Switzerland, Swiss), while morphology of ZnS-NPs was identified by field emission scanning electron microscopy (FESEM: Hitachi S-4160) under an acceleration voltage of 15 kV. The morphology and electron diffraction pattern of the ZnS-NPs were determined by a Hitachi H-800 TEM at an operating voltage of 200 kV. X-ray diffraction (XRD) pattern was recorded by an automated Philips X'Pert X-ray diffractometer (Philips, Holland, Netherland, 40 kV and 40 mA). The chromatographic analyses were carried out via the use of an Agilent Technologies (Wilmington, DE, USA) 1100 HPLC system equipped with Micro Vacuum Degasser (model G 1379A), Quaternary Pump (model G 1311A), a ZORBAX SB-C8 (Agilent) column and series multiple wavelength detector (model G13658). The chromatographic calculations were performed by ChemStation data handling system and Fourier transformation infrared (FTIR) spectrum was recorded using Perkin Elmer Spectrum RX-IFTIR spectrometer in the range of 300–4000 cm−1 at specified conditions. N2 adsorption–desorption isotherms were measured at 77 K to evaluate the pore structures.
2.3. Synthesis of ZnS-NPs-AC
The reaction solution for synthesis of ZnS nanoparticles (ZnS-NPs) was prepared as follows: aqueous solutions consisting of 15 mL of 1 mol L−1 zinc acetate and 15 mL of 11 mol L−1 acetic acid were mixed thoroughly in 100 mL glass beaker. Then, 37.5 mL of 0.4 mol L−1 thioacetamide solution was adjusted at pH = 5.2 and deionized water was added to the above mixture to make a total volume of 250 mL in a volumetric flask. 2.5 g activated carbon (AC) was added to 250 mL of the prepared reaction solution in an Erlenmeyer flask. Following the addition of AC to the reaction solution, the Erlenmeyer flask was transferred to microwave oven with power of 180 watt. Deposition of ZnS-NPs on activated carbon (AC) was carried out during 40 min in the microwave oven. The deposition solution was stirred strongly for 2 min at intervals of 10 min. After 40 min, the reaction solution was removed from the microwave oven to obtain a homogenous mixture of ZnS-NPs-AC. The suspended solution of the obtained ZnS-NPs-AC was stirred strongly at room temperature for 5 h. The prepared ZnS-NPs-AC was washed several times by distilled water and dried in a microwave oven with power of 180 watt for 50 min. The prepared adsorbent was used for adsorption experiments discussed in subsequent sections.
2.4. Adsorption experiments
Different naphthalene concentrations (5–50 mg L−1) were prepared from stock solution (200 mg L−1) for the equilibrium studies. 50 mL of each solution in conical flasks was mixed thoroughly with 0.02 g of ZnS-NPs-AC at pH around 5 and mixtures was shaken in a stirrer at room temperature until equilibrium was reached. Then, the mixture was centrifuged for 5 min at 3500 rpm to facilitate phase separation and simply the adsorbent was sediment at bottom of the tube and aliquot was decanted. In the next step, 25 μL of this solution was injected into HPLC-UV system for the analysis. The amount of adsorbed naphthalene was determined by subtraction from the initial concentration taken from the calibration curve and adsorption efficiency was calculated using eqn (1): |
 | (1) |
where A0 and A are the absorbance of naphthalene before and after adsorption, respectively. The amount of adsorption at equilibrium (qe (mg g−1)) was calculated using eqn (2). |
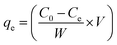 | (2) |
where, C0 and Ce (mg L−1) are the liquid phase concentrations of naphthalene at initial and equilibrium, respectively. W is ZnS-NPs-AC amount (g) and V is solution volume (mL). The applicability of each model is justified based on error function and calculation of chi-square (χ2) test. The coefficient of determination (R2) were employed as criteria to obtain the best isotherm and kinetic models for describing the experimental equilibrium data in a non-linear regression analysis.24 The following non-linear chi-square test (χ2)25 was carried out on the best-fitted isotherm: |
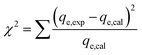 | (3) |
where, qe,exp and qe,cal are experimental and calculated adsorption capacities, respectively. A small value of χ2 indicates model efficiency and also suggests contribution to the acceptable experimental error.
2.5. Experimental design
A design of 30 experiments was formulated for central composite design (CCD) technique, while the range and level of experimental variables (coded at five levels −α, −1, 0, +1 and +α) are shown in Table S1.† The pH (A: 1.0, 3.0, 5.0, 7.0 and 9.0), initial naphthalene concentrations (B: 5, 15, 25, 35 and 45 mg L−1), absorbent doses (C: 0.005, 0.010, 0.015, 0.020 and 0.025 g) and contact time (5, 10, 15, 20 and 25 min) were chosen according to common and preliminary experiments. Data from CCD were subjected to the following quadratic equation model to predict the system response and estimate the coefficients by the least-squares regression:26 |
 | (4) |
where y, β0, βi, βii, and βij are the predicted response, the constant coefficient (intercept term), the linear coefficients, the quadratic coefficients and the interaction coefficients, respectively. The parameters xi and xj are independent variables, where k and e are number of factors and the residual term, allowing uncertainties between observed and predicted values, respectively.23,27 A half normal plot was used for choosing the important process variables and subsequently, the analysis of variance (ANOVA) gives knowledge about the validity of the model and significant contribution of each model. However, the related models are somewhat limited to only two levels in these types of designs and encourage to apply second-order model (response surface design). For the usage of more than two levels for fitting full quadratic model is necessary to find the best conditions for adsorption of naphthalene.28,29 Finally, some similar experiment was again performed under the obtained optimal conditions to validate the defined model.
3. Results and discussion
3.1. Characterization of adsorbent
The BET surface area of activated carbon (AC) and ZnS-NPs-AC were valuated to be 209.7 and 996.5 m2 g−1, respectively (Table 1). The measured total pore volume for ZnS-NPs-AC and AC were 0.543 and 0.160 cm3 g−1, while the microspore volume was 0.243 and −0.017 cm3 g−1, respectively. It is concluded from nitrogen adsorption/desorption isotherms and pore volume measurement that sample have porous structure and this evidence supports the enhancement of the surface area excellent sorption capacity of such materials.
Table 1 BET parameter for AC and ZnS-NPs-AC
|
AC |
ZnS-NPs-AC |
Unit |
Surface area |
BET surface area |
209.7 |
996.5 |
m2 g−1 |
Langmuir surface area |
297.9 |
1354.3 |
m2 g−1 |
t-Plot micropore area |
564.4 |
535.6 |
m2 g−1 |
t-Plot external surface area |
504.0 |
460.9 |
m2 g−1 |
BJH adsorption cumulative surface area of pores between 17.000 Å and 3000.000 Å width |
240.7 |
126.1 |
m2 g−1 |
BJH desorption cumulative surface area of pores between 17.000 Å and 3000.000 Å width |
301.2 |
146.8 |
m2 g−1 |
![[thin space (1/6-em)]](https://www.rsc.org/images/entities/char_2009.gif) |
Pore volume |
Single point adsorption to pore volume of pores less than 1298.012 Å width at p/p° = 0.98486 |
0.160 |
0.543 |
cm3 g−1 |
t-Plot micropore volume |
−0.017 |
0.243 |
cm3 g−1 |
BJH adsorption cumulative volume of pores between 17.000 Å and 3000.000 Å width |
0.404 |
0.146 |
cm3 g−1 |
BJH desorption cumulative volume of pores between 17.000 Å and 3000.000 Å width |
0.402 |
0.153 |
cm3 g−1 |
![[thin space (1/6-em)]](https://www.rsc.org/images/entities/char_2009.gif) |
Pore size |
Adsorption average pore width (4V/A by BET) |
74.60 |
21.80 |
Å |
BJH adsorption average pore width (4V/A) |
67.14 |
46.27 |
Å |
BJH desorption average pore width (4V/A) |
53.41 |
41.58 |
Å |
![[thin space (1/6-em)]](https://www.rsc.org/images/entities/char_2009.gif) |
Nanoparticle size |
Average particle size |
— |
60.21 |
Å |
The surface morphology and size of AC, ZnS-NPs and ZnS-NPs-AC were investigated by FESEM, SEM and shown in Fig. 1a–d. It may be seen that the shape is spherical with unvarying and smooth surface and size was estimated in the range of 50–150 nm. A typical TEM image of the AC and ZnS-NPs (Fig. 2) shows relatively narrow particle size distribution (50–150 nm range).
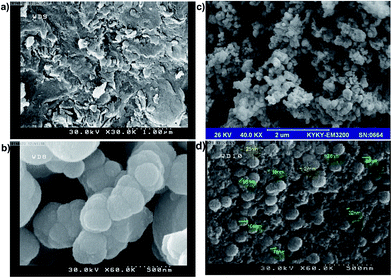 |
| Fig. 1 FE-SEM images of the (a) AC, (b) ZnS-NPs, (c) SEM of the ZnS-NPs and (d) FE-SEM of the ZnS-NPs-AC. | |
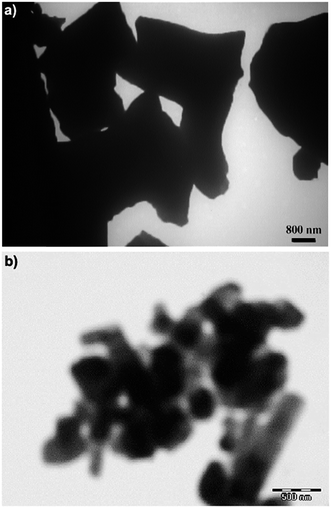 |
| Fig. 2 TEM of the (a) AC and (b) ZnS-NPs. | |
The particle size distribution of ZnS-NPs, determined by the laser particle size analyzer is shown in Fig. 3a. It shows that the sizes of the ZnS-NPs particles are in the range of 20–110 nm. More than 90% particles are of below 80 nm. Average particle size of the adsorbent is 62 nm.
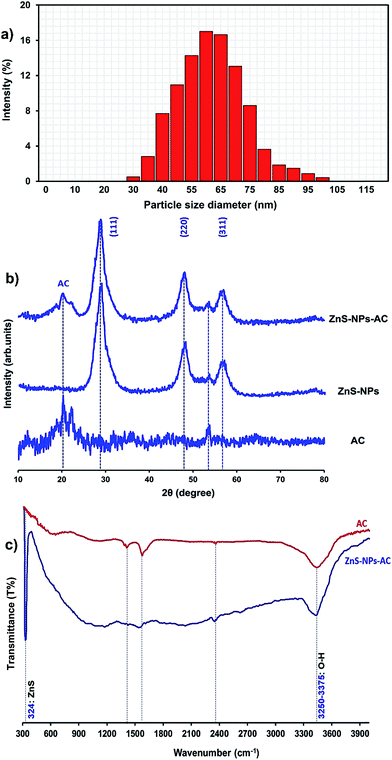 |
| Fig. 3 (a) Particle size distribution of the ZnS-NPs, (b) XRD pattern of the AC, ZnS-NPs and ZnS-NPs-AC and (c) FTIR of the AC and ZnS-NPs-AC. | |
The XRD pattern of AC, ZnS-NPs and ZnS-NPs-AC (Fig. 3b) shows three strong peaks at 2θ = 28.75, 47.88, and 56.65° assigned to (111), (220), and (311) lattice planes of ZnS cubic zinc blend and its polycrystalline pure structure with good concurrence with FTIR spectrum results (JCPDS no. 00-005-0566), respectively.
The strong XRD peaks (Fig. 3b) indicates the well crystalline structure of ZnS nanoparticles. On the basis of the full width at half-maximum (FWHM) of (111) peak and applying the Debeye–Scherrer equation: D = 0.9λ/β
cos
θ, where λ is the X-ray wavelength of 0.1542 nm, β is the full width at half maximum, and θ is the Bragg diffraction angle, the average nanocrystallite size of ZnS nanoparticles was estimated to be 40.0 nm.28,30
FTIR spectrum of AC and ZnS-NPs-loaded on AC was recorded in the range of 4000–300 cm−1 and shown in Fig. 3c. In the obtained FT-IR spectrum, any peak corresponding to impurities such as Zn–O and Zn–OH was not observed. The peak at 324 cm−1 (inset of Fig. 2b) is due to Zn–S vibrational mode of ZnS-NPs.31 The broad absorption peak in the range of 3300–3600 cm−1 is accredited to H–O–H bending vibration mode of H2O or hydroxyl or carboxylic group present in AC structure. These peaks also may come up from the presence of a trace amount of adsorbed water in the surface of the ZnS-NPs.
3.2. Chemometric analysis
In the CCD, 30 random experiments were preferred to minimize the effect of uncontrolled variables (Table S1†) including pH (A), naphthalene concentration (B), adsorbent mass (C) and contact time (D) into three levels (low, central, high) as coded value (−1, 0, +1) and the star points of +2 and −2 for +a and −a, respectively. The response values corresponding to all 30 experiments are presented in Table S1.† The main, interaction and quadratic effects were evaluated to find the most important effects and their interactions. Analysis of variance (ANOVA) was performed by STATISTICA 10 (Table 2). The significance of linear or interaction terms was investigated at a confidence level of 95%. According to Table 2, the pH, naphthalene concentration, adsorbent mass and contact time significantly affect the response in a linear trend with no significant interaction. F-Test was used to estimate the statistical connotation of all terms in the polynomial equation within 95% confidence level.
Table 2 Analysis of variance for response surface quadratic model for optimization of naphthalene adsorption onto ZnS-NPs-AC
Source of variation |
SSa |
Dfb |
MSc |
F-Valued |
P-Valuee |
|
Sum of squares. Degree of freedom. Mean of squares error. F test. Probability value. Standard deviation. Coefficient of variation. R-Squared. Adjusted R-squared. Predicted R-squared. Adequate precision. |
Model |
9974.40 |
14 |
712.46 |
78.34 |
<0.0001 |
Significant |
A |
411.96 |
1 |
411.96 |
45.30 |
<0.0001 |
Significant |
B |
1897.73 |
1 |
1897.73 |
208.67 |
<0.0001 |
Significant |
C |
5671.35 |
1 |
5671.35 |
623.61 |
<0.0001 |
Significant |
D |
706.31 |
1 |
706.31 |
77.66 |
<0.0001 |
Significant |
AB |
254.36 |
1 |
254.36 |
27.97 |
<0.0001 |
Significant |
AC |
14.20 |
1 |
14.20 |
1.56 |
0.2306 |
Not significant |
AD |
120.04 |
1 |
120.04 |
13.20 |
0.0025 |
Significant |
BC |
286.91 |
1 |
286.91 |
31.55 |
<0.0001 |
Significant |
BD |
92.61 |
1 |
92.61 |
10.18 |
0.0061 |
Significant |
CD |
28.34 |
1 |
28.34 |
3.12 |
0.0979 |
Not significant |
A2 |
4.17 |
1 |
4.17 |
0.46 |
0.5085 |
Not significant |
B2 |
21.79 |
1 |
21.79 |
2.40 |
0.1425 |
Not significant |
C2 |
436.41 |
1 |
436.41 |
47.99 |
<0.0001 |
Significant |
D2 |
99.21 |
1 |
99.21 |
10.91 |
0.0048 |
Significant |
Residual |
136.41 |
15 |
9.09 |
|
|
|
Lack of fit |
117.81 |
10 |
11.78 |
3.17 |
0.1076 |
Not significant |
Pure error |
18.60 |
5 |
3.72 |
|
|
|
Cor total |
10 110.81 |
29 |
|
|
|
|
Quadratic summary statistics |
Response |
SDf |
Mean |
CVg% |
R2h |
Adj-R2i |
Pred-R2j |
APk |
R% naphthalene |
3.016 |
78.51 |
3.841 |
0.9865 |
0.9739 |
0.9302 |
33.36 |
A final empirical model for the prediction of naphthalene in adsorption in terms of coded factors was described by the following equation:
|
R% naphthalene = −7.2 + 6.6A − 1.44B + 6071C + 4.3D − 0.2AB − 0.3AD + 84.7BC + 0.05BD − 159600C2 − 0.1D2
| (5) |
Lack of fit (LOF) is a criterion to test the adequacy of the model. Table 2 shows the P-value for LOF to be 0.1076 that indicates high suitability of model for well-fitting the experimental data.32 In addition, the quality of fitting was revealed by making an allowance for the coefficient of determination (R2 0.98, adj-R2 0.97 and pred-R2 0.93). These large values indicate a good relationship between the experimental data and the fitted model.33 High correlation between observed and predicted (Fig. S1a†) data indicates their low discrepancies and also rationalize predictions of the experimental data by quadratic models were satisfactory.
The plot of percentage naphthalene adsorption versus raw residual (Fig. S1b†) did not show any outliers so that all the points were found to fall in the range of −5.0 to +4.0. These reasonable and acceptable distributions of results confirm minimal deviation of the fitted value from the observed value and also suggest model suitability.
Fig. S1c† shows normal probability plot of residual values as the difference between predicted values (model) and the observed values (experimental).34 Experimental points were reasonably aligned suggesting normal distribution. All the points of the normal probability plot are found to fall in the range of −2.3 to +1.6 for the adsorption of naphthalene onto ZnS-NPs-AC.
The Pareto analysis indicates the extent of the influence of each variable on the response factor and quantified percentage effect of each term on the response.35 The length of each bar in the chart indicates its standardized effect on the response. The remaining (A2, B2, AC and CD) signals inside the reference line in Fig. S1† suggest their least and non-significant contribution on the response. The negative coefficients for the model components (A and B) indicated an unfavorable or antagonistic effect on the adsorption of naphthalene, while the positive coefficients for the model components (C and D) showed a favorable or synergistic effect on the adsorption of naphthalene.
3.3. Three-dimensional (3D) response surfaces and contour plots
The explanation of multiple inputs and output method was simply obtained by their geometrical representation (especially surface diagram) best approach. Fig. 4 shows the response surface curves of naphthalene adsorption efficiency, influenced by the independent variables. Fig. 4a presents the interaction of pH with sonication time. The high removal percentage of naphthalene was observed in high pH for early sonication times because of the fact that at high pH, ZnS-NPs-AC surface gets a negative charge due to protonation of the functional groups. The contact time strongly affect the naphthalene adsorption efficiency. A maximum adsorption of naphthalene could be achieved in 15 min contact time, which confirms a high contribution of interaction in mass transfer. Mixing and dispersion of adsorbent in a solution, the diffusion coefficient of naphthalene onto ZnS-NPs-AC, mass transfer of aggregate to the surface area and vacant sites of ZnO-NR-AC are also enhanced using contact time. The effect of ZnS-NPs-AC dose (g) and initial concentration of naphthalene (mg L−1) on naphthalene adsorption efficiency is depicted in Fig. 4b. The obtained results showed that the removal efficiency of naphthalene increases slightly as the doses of ZnS-NPs-AC increases. In addition, increasing the initial naphthalene concentration led to a decrease in naphthalene adsorption efficiency. At high concentrations of naphthalene, a significant small adsorption is possibly due to the saturation of surface active sites with the adsorbate molecules. At higher naphthalene ion concentrations, the available adsorption sites decreased compared to the molecules of the naphthalene present in low concentration, and thus adsorption of naphthalene decreased.
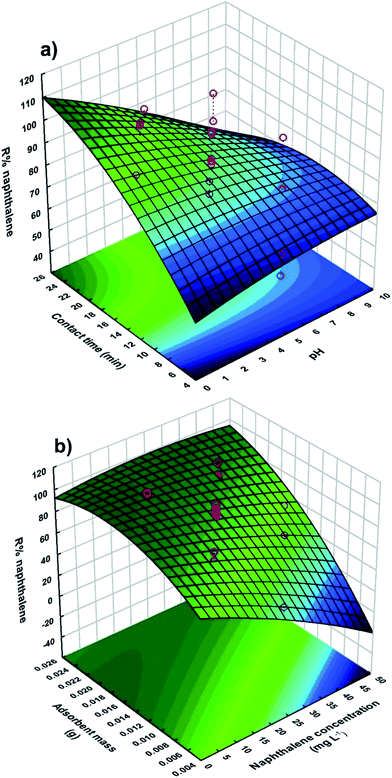 |
| Fig. 4 The response surface plots of R% naphthalene versus: (a) contact time–pH and (b) adsorbent mass–naphthalene concentration. | |
3.4. Optimization of the adsorption method using desirability function
The adsorption performance can be evaluated in term of adsorption efficiency, which largely varies with changes in variables. Thus, optimization of variables is important factor for the process. The compromises between the variables were made depending upon the responses and desirability from model for economic viability. The numerical optimization found a point that maximizes the desirability function. The numerical optimization indicates that the optimum naphthalene adsorption (99.39%) can be obtained at ZnS-NPs-AC dose of 0.02 g, initial naphthalene concentration of 15 mg L−1, contact time of 15 min, and initial solution pH of 5.0 (Fig. S2†). The comparison in the optimal conditions between predicted and observed values of response were investigated. Observed values were the measured data from experiments, and the predicted values were calculated by using eqn (5). In this run, the adsorption efficiency reached 98.89% as observed value, while predicted value was 99.39%. The results confirmed that the predicted value was in good agreement with the observed value and the model performance was validated.
3.5. Adsorption isotherm
In this section, widely used Langmuir (Fig. S3a†), Freundlich (Fig. S3b†), Temkin (Fig. S3c†), Dubinin–Radushkevich (Fig. S3d†) and Harkins–Jura (H–J) isotherm models are applied to simulate and understand the adsorption mechanism of naphthalene onto ZnS-NPs-AC at different adsorbent dose. Several isotherm equations are available and four important isotherms are applied to fit the equilibrium data. The Langmuir, Freundlich, Temkin, Dubinin–Radushkevich (D–R) and Harkins–Jura isotherms are listed in Table 3.36–40 From a comparison of the correlation coefficients and non-linear χ2 values of the five-parameter isotherms, the Langmuir isotherm with a high correlation coefficient (>0.990) and lower χ2 values (0.02–0.07) seemed to be the best fitting model for adsorption of naphthalene onto ZnS-NPs-AC, which indicated that adsorption sites were energetically homogeneous.
Table 3 Model parameters estimated for the adsorption isotherms of naphthalene onto ZnS-NPs-AC
Isotherm |
Parameters |
Plot |
Value of parameters |
0.01 g |
0.02 g |
Langmuir Ce/qe = (1/KaQm) + Ce/Qm |
Qm (mg g−1) |
Ce/qe vs. Ce |
142.68 |
96.950 |
Ka (L mg−1) |
0.110 |
0.275 |
R2 |
0.9935 |
0.9916 |
χ2 |
0.0200 |
0.076 |
Freundlich ln qe = ln KF + (1/n)ln Ce |
1/n |
ln qe vs. ln Ce |
0.6485 |
0.589 |
KF (L mg−1) |
2.870 |
2.230 |
R2 |
0.9438 |
0.959 |
χ2 |
0.210 |
0.206 |
Temkin qe = B1 ln KT + B1 ln Ce |
B1 |
qe vs. ln Ce |
17.46 |
6.490 |
KT (L mg−1) |
1.20 |
2.690 |
R2 |
0.990 |
0.989 |
χ2 |
0.060 |
0.0934 |
Dubinin–Radushkevich (D–R) ln qe = ln Qs −Kε2, ε = RT ln(1 + 1/Ce) |
Qs (mg g−1) |
ln qe vs. ε2 |
122.9 |
87.630 |
K × 10−7 |
4.00 |
3.00 |
E (kJ mol−1) |
1.118 |
1.291 |
R2 |
0.902 |
0.952 |
χ2 |
2.502 |
1.960 |
Harkins–Jura (H–J) 1/qe2 = (B2/A) − (1/A)log Ce |
A |
1/qe2 vs. log Ce |
12.340 |
27.70 |
B2 |
0.100 |
8.220 |
R2 |
0.593 |
0.663 |
χ2 |
4.90 |
4.560 |
The maximum monolayer adsorption capacity of ZnS-NPs-AC for the adsorption of naphthalene was compared with those of other adsorbents reported in the literature and the values are shown in Table 4. It is clear from Table 4 that the adsorption capacities of ZnS-NPs-AC adsorbents were greater than the previously reported values which suggested that these are promising adsorbents for the adsorption of naphthalene from aqueous solutions. It was also noted that contact time for adsorption using the said adsorbent was lower than other adsorbents reported in the literature.5,17,41
Table 4 Comparison for the adsorption of naphthalene onto ZnS-NPs-AC by different methods and adsorbents
Adsorbent |
Adsorption capacity (mg g−1) |
Contact time (min) |
Ref. |
ZnS-NPs-AC |
142.68 |
15 |
This work |
Graphene oxide (GO) nanosheets |
0.167 |
1440 |
47 |
Graphene oxide (GO) nanosheets-Paecilomyces cateniannulatus |
112.3 |
1440 |
47 |
Sewage sludge (SS) |
84.56 |
60–100 |
48 |
Reed straw-based carbons (RSC) |
110.22 |
60–100 |
48 |
Sewage sludge-based activated carbon (SSC) |
157.52 |
60–100 |
48 |
Coal-based activated carbon (CAC) |
75.76 |
20 |
49 |
Coal-based activated carbons-anthracite coal (ACAC) |
165.67 |
60 |
50 |
Coal-based activated carbons-bituminous coal (BCAC) |
161.21 |
60 |
50 |
Coal-based activated carbons-long flame coal (LCAC) |
147.10 |
60 |
50 |
3.6. Kinetic studies
The kinetics of adsorption data was analyzed to understand the dynamics of adsorption process in terms of order of the rate constant. Kinetic models were applied to the experimental adsorption data to investigate the behavior of adsorption process of naphthalene onto ZnS-NPs-AC. These models are the pseudo-first and pseudo-second order kinetics, intraparticle diffusion and Elovich models.42–45 The adsorption kinetic data were described by the Lagergren pseudo-first-order model, which differential equation is generally expresses as follows:41 |
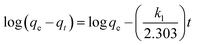 | (6) |
The pseudo-second-order equation is expressed as
|
 | (7) |
where,
qe and
qt are the amount of naphthalene adsorbed (mg g
−1) at equilibrium and at time ‘
t’, respectively. The product
k2qe2 is the initial sorption rate, represented as
h =
k2qe2. The kinetic parameters of these models were calculated from the slope and intercept of the linear plots of log(
qe −
qt)
versus t and
t/
qt versus t and are given in
Table 5.
Table 5 Kinetic parameters for naphthalene adsorption onto ZnS-NPs-AC at different initial concentrations and adsorbent doses
Model |
Parameters |
Value of parameters |
0.01 g |
0.02 g |
10 mg L−1 |
20 mg L−1 |
30 mg L−1 |
40 mg L−1 |
10 mg L−1 |
20 mg L−1 |
30 mg L−1 |
40 mg L−1 |
First-order kinetic |
k1 (min−1) |
9.47 |
9.01 |
6.54 |
4.74 |
9.03 |
9.26 |
8.19 |
5.39 |
qe (calc) (mg g−1) |
37.84 |
66.16 |
106.07 |
145.21 |
19.07 |
32.15 |
59.77 |
104.52 |
R2 |
0.946 |
0.955 |
0.924 |
0.815 |
0.847 |
0.834 |
0.89 |
0.821 |
Pseudo-second-order kinetic |
k2 (min−1) |
0.01 |
0.01 |
0.003 |
0.003 |
0.02 |
0.01 |
0.01 |
0.004 |
qe (calc) (mg g−1) |
123.46 |
163.93 |
232.56 |
285.71 |
92.59 |
123.46 |
181.82 |
222.22 |
R2 |
0.999 |
0.998 |
0.998 |
0.999 |
0.999 |
0.999 |
0.999 |
0.999 |
h |
152.42 |
268.73 |
292.25 |
344.89 |
171.46 |
192.42 |
230.59 |
297.53 |
Intraparticle diffusion |
Kdif (mg g−1 min−1/2) |
9.48 |
15.53 |
23.91 |
30.93 |
5.77 |
9.57 |
16.17 |
24.33 |
C (mg g−1) |
76.82 |
85.33 |
111.57 |
132.65 |
65.42 |
77.87 |
103.21 |
104.03 |
R2 |
0.891 |
0.935 |
0.899 |
0.822 |
0.775 |
0.819 |
0.801 |
0.811 |
Elovich |
β (g mg−1) |
0.083 |
0.052 |
0.033 |
0.025 |
0.129 |
0.079 |
0.047 |
0.031 |
α (mg g−1 min−1) |
11 293.7 |
2818.6 |
1964.5 |
1690.9 |
55 270.8 |
9519.5 |
4182.2 |
1242.7 |
R2 |
0.985 |
0.977 |
0.985 |
0.946 |
0.938 |
0.956 |
0.949 |
0.946 |
|
qe (exp) (mg g−1) |
124.29 |
165.37 |
245.17 |
322.06 |
93.32 |
124.24 |
185.62 |
243.31 |
For porous adsorbents, the diffusion of the adsorbate molecules or ions into the pores is also to be taken into account to find a suitable kinetic model for the process. Weber and Morris46 introduced a simple expression to obtain the diffusion rate coefficient (Kdif) which is given as:
The values of rate coefficient, Kdif (mg g−1 min−0.5) could be obtained from the slope of the plot, qt versus t1/2. The values of Kdif and C (mg g−1) are summarized in Table 5 along with respective correlation coefficient (R2). The Elovich equation has been used in the following form:
|
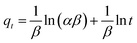 | (9) |
It is postulated that the Elovich constants α and β, represent the initial adsorption rate (g mg−1 min−2) and the desorption constant (mg g−1 min−1), respectively. The Elovich constants could be computed from the plots of qt versus ln
t (Table 5).
The R2 values for pseudo-second order kinetic model at all the concentrations studied are higher than those for pseudo-first order model (Table 5), indicating that the pseudo-second order model is more suitable for describing the adsorption behavior of naphthalene onto ZnS-NPs-AC. It is also shown in Table 5 that the initial adsorption rate (h) increases, while the rate constant (k2) decreases with the increase in initial naphthalene concentration. The high values of correlation coefficient (R2) ascertained the suitability of this model for the adsorption of naphthalene onto ZnS-NPs-AC adsorbents (Table 5).
4. Conclusion
In this work, ZnS-NPs-AC was synthesized and characterized by FTIR, XRD, FESEM, and BET analysis. The said adsorbent material was loaded with AC and applied for the adsorption of naphthalene from aqueous medium. Response surface methodology was used to optimize the process variables, such as pH, naphthalene concentration, adsorbent doses and contact time. The quadratic model was developed for process optimization and statistical experimental designs were found to be a useful tool for the prediction and understanding the interaction effects among the parameters. The optimal condition of naphthalene adsorption on ZnS-NPs-AC was determined by RSM as: 0.02 g adsorbent dose, 15 min contact time, 5.0 pH and 15 mg L−1 naphthalene leading to 98.8% removal. The experimental results were in good agreement with that predicted from the model. Moreover, the kinetic studies showed the best fit with pseudo-second order rate equation. Besides, the adsorption equilibrium showed the best fitting with the Langmuir isotherm equation, signifying monolayer adsorption process.
Acknowledgements
The authors grateful from the Iranian national sciences foundation (INSF for grant number of 93014889) and Research Council of the University of Yasouj for their financial support.
References
- J.-J. Sauvain, T. V. Duc and M. Guillemin, Int. Arch. Occup. Environ. Health, 2003, 76, 443–455 CrossRef CAS PubMed.
- K. Yang, W. Wu, Q. Jing and L. Zhu, Environ. Sci. Technol., 2008, 42, 7931–7936 CrossRef CAS PubMed.
- H. Richter and J. B. Howard, Prog. Energy Combust. Sci., 2000, 26, 565–608 CrossRef CAS.
- S. K. Samanta, O. V. Singh and R. K. Jain, Trends Biotechnol., 2002, 20, 243–248 CrossRef CAS PubMed.
- M. O. Ansari, R. Kumar, N. Parveen, M. A. Barakat and M. H. Cho, New J. Chem., 2015, 39, 7004–7011 RSC.
- X. Ge, F. Tian, Z. Wu, Y. Yan, G. Cravotto and Z. Wu, Chem. Eng. Process., 2015, 91, 67–77 CrossRef CAS.
- S. D. Chakarova-Käck, E. Schröder, B. I. Lundqvist and D. C. Langreth, Phys. Rev. Lett., 2006, 96, 146107 CrossRef PubMed.
- M. Anbia and S. E. Moradi, Chem. Eng. J., 2009, 148, 452–458 CrossRef CAS.
- C. Ania, B. Cabal, C. Pevida, A. Arenillas, J. Parra, F. Rubiera and J. Pis, Water Res., 2007, 41, 333–340 CrossRef CAS PubMed.
- M. Anbia and S. E. Moradi, Appl. Surf. Sci., 2009, 255, 5041–5047 CrossRef CAS.
- F. J. Rivas, J. Hazard. Mater., 2006, 138, 234–251 CrossRef CAS PubMed.
- A. Haritash and C. Kaushik, J. Hazard. Mater., 2009, 169, 1–15 CrossRef CAS PubMed.
- Y. Zhang, Z.-R. Tang, X. Fu and Y.-J. Xu, ACS Nano, 2010, 4, 7303–7314 CrossRef CAS PubMed.
- K. Yang, L. Zhu and B. Xing, Environ. Sci. Technol., 2006, 40, 1855–1861 CrossRef CAS PubMed.
- F. Villacañas, M. F. R. Pereira, J. J. Órfão and J. L. Figueiredo, J. Colloid Interface Sci., 2006, 293, 128–136 CrossRef PubMed.
- C. Long, A. Li, H. Wu and Q. Zhang, Colloids Surf., A, 2009, 333, 150–155 CrossRef CAS.
- R. Kumar, M. O. Ansari, N. Parveen, M. A. Barakat and M. H. Cho, RSC Adv., 2015, 5, 61486–61494 RSC.
- A. Asfaram, M. Ghaedi, M. H. A. Azqhandi, A. Goudarzi and M. Dastkhoon, RSC Adv., 2016, 6, 40502–40516 RSC.
- E. A. Dil, M. Ghaedi, A. M. Ghaedi, A. Asfaram, A. Goudarzi, S. Hajati, M. Soylak, S. Agarwal and V. K. Gupta, J. Ind. Eng. Chem., 2016, 34, 186–197 CrossRef CAS.
- A. Asfaram, M. Ghaedi and A. Goudarzi, Ultrason. Sonochem., 2016, 32, 407–417 CrossRef CAS PubMed.
- A. Asfaram, M. Ghaedi, F. Yousefi and M. Dastkhoon, Ultrason. Sonochem., 2016, 33, 77–89 CrossRef CAS PubMed.
- A. Asfaram, M. Ghaedi, A. Goudarzi and M. Soylak, RSC Adv., 2015, 5, 39084–39096 RSC.
- A. Asfaram, M. Ghaedi, A. Goudarzi and M. Rajabi, Dalton Trans., 2015, 44, 14707–14723 RSC.
- F. N. Azad, M. Ghaedi, A. Asfaram, A. Jamshidi, G. Hassani, A. Goudarzi, M. H. A. Azqhandi and A. Ghaedi, RSC Adv., 2016, 6, 19768–19779 RSC.
- A. Asfaram, M. Ghaedi, S. Hajati and A. Goudarzi, RSC Adv., 2015, 5, 72300–72320 RSC.
- M. Ghaedi, H. Z. Khafri, A. Asfaram and A. Goudarzi, Spectrochim. Acta, Part A, 2016, 152, 233–240 CrossRef CAS PubMed.
- E. A. Dil, M. Ghaedi, A. Ghaedi, A. Asfaram, M. Jamshidi and M. K. Purkait, J. Taiwan Inst. Chem. Eng., 2016, 59, 210–220 CrossRef CAS.
- A. Asfaram, M. Ghaedi, S. Agarwal, I. Tyagi and V. Kumar Gupta, RSC Adv., 2015, 5, 18438–18450 RSC.
- F. Nasiri Azad, M. Ghaedi, K. Dashtian, S. Hajati, A. Goudarzi and M. Jamshidi, New J. Chem., 2015, 39, 7998–8005 RSC.
- M. Ghaedi, Z. Rozkhoosh, A. Asfaram, B. Mirtamizdoust, Z. Mahmoudi and A. Bazrafshan, Spectrochim. Acta, Part A, 2015, 138, 176–186 CrossRef CAS PubMed.
- A. Goudarzi, A. D. Namghi and C.-S. Ha, RSC Adv., 2014, 4, 59764–59771 RSC.
- A. Asfaram, M. Ghaedi, S. Hajati and A. Goudarzi, Ultrason. Sonochem., 2016, 32, 418–431 CrossRef CAS PubMed.
- M. Dastkhoon, M. Ghaedi, A. Asfaram, A. Goudarzi, S. M. Langroodi, I. Tyagi, S. Agarwal and V. K. Gupta, Sep. Purif. Technol., 2015, 156(2), 780–788 CrossRef CAS.
- S. Satyro, M. Race, F. Di Natale, A. Erto, M. Guida and R. Marotta, Chem. Eng. J., 2016, 283, 1484–1493 CrossRef CAS.
- A. Asfaram, M. Ghaedi, A. Goudarzi, M. Soylak and S. Mehdizadeh Langroodi, New J. Chem., 2015, 39, 9813–9823 RSC.
- Z. Yu, S. Peldszus and P. M. Huck, Environ. Sci. Technol., 2009, 43, 1467–1473 CrossRef CAS PubMed.
- K. Yang, L. Zhu and B. Xing, Environ. Sci. Technol., 2006, 40, 1855–1861 CrossRef CAS PubMed.
- M. Temkin and V. Pyzhev, Acta Physicochim. URSS, 1940, 12, 217–222 Search PubMed.
- M. Dubinin and L. Radushkevich, Chem. Zentralbl., 1947, 1, 875–889 Search PubMed.
- H. Tahermansouri, Z. Dehghan and F. Kiani, RSC Adv., 2015, 5, 44263–44273 RSC.
- R. Kumar, M. O. Ansari and M. Barakat, Ind. Eng. Chem. Res., 2014, 53, 7167–7175 CrossRef CAS.
- S. Lagergren, K. Sven. Vetenskapsakad. Handl., 1898, 24, 1–39 Search PubMed.
- R. Ocampo-Perez, F. Orellana-Garcia, M. Sanchez-Polo, J. Rivera-Utrilla, I. Velo-Gala, M. V. Lopez-Ramon and M. A. Alvarez-Merino, J. Colloid Interface Sci., 2013, 401, 116–124 CrossRef CAS PubMed.
- J. Yu, L. Lv, P. Lan, S. Zhang, B. Pan and W. Zhang, J. Hazard. Mater., 2012, 225–226, 99–106 CrossRef CAS PubMed.
- A. Reguer, S. Sochard, C. Hort and V. Platel, Environ. Technol., 2011, 32, 757–766 CrossRef CAS PubMed.
- W. J. Weber and J. C. Morris, J. Sanit. Eng. Div., Am. Soc. Civ. Eng., 1964, 90, 79–108 CAS.
- X. Li, F. Li and L. Fang, J. Nanopart., 2015, 1, 1–7 CrossRef.
- L. Gu, H. Guo, P. Zhou, N. Zhu, D. Zhang, H. Yuan and Z. Lou, Environ. Sci. Pollut. Res., 2014, 21, 2043–2053 CrossRef CAS PubMed.
- X. Ge, X. Ma, Z. Wu, X. Xiao and Y. Yan, Res. Chem. Intermed., 2015, 41, 7327–7347 CrossRef CAS.
- D. Liu, Z. Wu, X. Ge, G. Cravotto, Z. Wu and Y. Yan, J. Taiwan Inst. Chem. Eng., 2016, 59, 563–568 CrossRef CAS.
Footnote |
† Electronic supplementary information (ESI) available. See DOI: 10.1039/c6ra09500c |
|
This journal is © The Royal Society of Chemistry 2016 |