DOI:
10.1039/C6RA09264K
(Paper)
RSC Adv., 2016,
6, 47698-47711
Influence of CrB2 target current on the microstructure, mechanical and tribological properties of Cr–B–C–N coatings in water
Received
11th April 2016
, Accepted 8th May 2016
First published on 9th May 2016
Abstract
Cr–B–C–N coatings with different boron contents (24.6–27.2 at%) were deposited on Si(100) wafers and 316L stainless steels by using closed-field unbalanced magnetron sputtering via adjusting the CrB2 target current. The microstructures, mechanical and tribological properties of Cr–B–C–N coatings in water were characterized by using XRD, XPS, SEM, nano-indentation and ball-on-disk tribometers. The results showed that the soft amorphous BN phases were formed when boron elements were introduced into CrCN coatings. With an increase in the CrB2 target current, the B content increased from 24.9 to 27.2 at%, which caused the a-BN content in CrBCN coatings to increase gradually, and then the hardness of CrBCN coatings decreased from 18.6 ± 0.86 GPa to 16.3 ± 0.28 GPa. Due to the formation of H3BO3, the friction coefficient and wear rates of CrBCN/SiC tribopairs were lower than those of CrCN/SiC tribopairs. When the CrBCN coatings (25.1 at% B) were deposited at the CrB2 target current of 3 A, the lowest values of friction coefficient (0.16) and wear rate (2.1 × 10−7 mm3 N−1 m−1) were obtained simultaneously.
1. Introduction
In comparison to the conventional oil lubrication systems, water lubrication systems are an effective way to save energy sources and to protect the natural environment. However, water possesses a relatively low viscosity and a strong corrosion ability, which restrains its wide application for metallic materials. A promising approach to solve this technical problem is to modify the surface properties of metallic materials by depositing hard coatings with a good lubricity in water. Currently, carbon-based and transition metal nitride-based coatings have been deemed to be promising surface coatings on the sliding parts in water hydraulic systems.1,2 Although carbon-based coatings, such as diamond, DLC, a-CNx and GLC, have exhibited low friction coefficients and wear rates in water, their practical applications have been limited owing to the poor adhesion to the substrates originating from their high internal stress.3–6 But for transition metal nitride-based coatings such as TiN-based coatings, due to their high hardness, strong wear resistance, good chemical inertness, thermal stability and great adhesion to the substrate, they have been widely utilized as protective hard coatings.7,8 As compared with the TiN coatings, TiCN coatings, as a combination of TiN and TiC, showed superior wear resistance due to their high hardness and the formation of self-lubricating carbon, while TiBN coatings, consisting of TiN, TiB2, c-BN and a-BN phases, exhibited advantageous properties for cutting applications due to their high hardness, strong toughness and great thermodynamic stability at high temperatures.9–14 To meet the demand for advanced coatings, quaternary TiBCN coatings have attracted researchers' concerns due to their super hardness, good tribological properties and strong corrosion resistance and oxidation resistance.15–17 In comparison to the TiN-based coatings, the CrN-based coatings exhibited a superior corrosion and oxidation resistance and a lower internal stress.18–20 As seen in Table 1 in ref. 21, it is clear that the friction coefficients of the CrN-based coatings sliding against different balls in water environment varied in the range of 0.079–0.56, while the specific wear rates of the CrN-based coatings changed from the magnitude of 10−9 to 10−5 mm3 N−1 m−1. This indicates that the CrN-based coatings could be used in water environment. However, pure CrN coatings could not be qualified under some extreme conditions because of a relatively low hardness in comparison to pure TiN coatings. Thus, carbon or boron element has been proposed being added into CrN coatings to improve their mechanical properties, so as to enhance the corresponding tribological properties in water.22–24 It has been demonstrated that the hardness and wear resistance of CrN coatings were enhanced after carbon incorporation.25,26 Wang et al.26 have reported that the hardness of CrCN coatings with 15.35 at% C reached 22.5 GPa, higher than that (18.5 GPa) of CrN coatings. Moreover, when CrCN coatings slid against Si3N4 ceramic balls in water, a low friction coefficient (0.197) was obtained due to the formation of amorphous carbon or carbon nitride layer acting as a solid lubricant at contact area. On the other hand, when boron element was added into the CrN coatings, the CrBN coatings presented a nanocomposite structure consisting of CrN crystallite and amorphous BN phase.27,28 Due to a smaller grain size and the interaction between crystallite phase and amorphous phase, CrBN coatings exhibited higher hardness than CrN coatings. Zhang et al.28 reported that the CrBN coatings with 6.1 at% B exhibited a maximum hardness (∼24 GPa) and a minimum wear rate (0.04 μm3 N−1 m−1) in comparison with the other coatings. Furthermore, the self-lubricating B(OH)3 films resulted from a spontaneous chemical reaction between water molecules and B2O3 could contribute to a low friction coefficient when the boride coatings were under friction tests in high humidity environment.29 Thus, quaternary CrBCN coatings are expected to present better tribological performance in water than CrCN and CrBN coatings. However, up to now, the friction and wear behaviors of CrBCN coatings in water environment have not yet been studied.
Table 1 Deposition parameters of Cr–B–C–N coatings
Parameter |
Figure |
Chamber pressure |
0.17 Pa |
Temperature |
150 °C |
Bias voltage |
−80 V |
Rotating speed of holder |
10 rpm |
Optical emission monitor (OEM) |
50% |
Current of Cr target |
4 A |
Current of C target |
4 A |
Current of CrB2 target |
0–4 A |
In here, Cr–B–C–N coatings with various boron contents were deposited on Si wafers and 316L stainless steels using unbalanced magnetron sputtering system by way of adjusting the CrB2 target current. The microstructures, mechanical and water-lubricated tribological properties of Cr–B–C–N coatings were investigated, and then the influences of boron content on the microstructure, mechanical and tribological properties were systematically discussed.
2. Experimental details
2.1 Deposition of Cr–B–C–N coatings
Cr–B–C–N coatings were deposited on Si(100) wafers and 316L stainless steel disks using closed-field unbalanced magnetron sputtering system (UDP-650, Teer Coatings Limited, UK), where two pure chromium targets (99.95%), one pure carbon target (99.95%) and one CrB2 target (99.9%) were mounted. The coatings deposited on Si(100) wafers were used for the characterizations of EDS, XRD, XPS and SEM, while those on 316L disks were used for the friction tests in tap water. At first, 316L stainless steel disks with dimensions of Φ 30 mm × 4 mm were polished to a surface roughness (Ra) of 30 nm by using a metallographic polishing machine (UNIPOL-820). Then the as-received Si(100) wafers and the polished 316L disks were ultrasonically cleaned in ethanol and deionized water for 20 minutes before being fixed on substrate holder. Then the background pressure of deposition chamber was pumped down to about 4 × 10−4 Pa and the substrate holder was kept rotating continuously around its central axis at a speed of 10 rpm. Prior deposition, Ar sputtering plasma generated at a bias voltage of −450 V was conducted for 30 minutes to further clean the residual contaminations and oxide layers on the substrate surface, and then a Cr adhesive layer (∼0.2 μm) was deposited in advance to enhance the adhesive strength between the substrate and coating. All the coatings were deposited by sputtering in a mixture of Ar and N2 gases without shielding to restrict the interaction of gas and plasma streams. The inert Ar gas was used as working gas (0.17 Pa), while N2 was introduced as the reactive gas (∼0.06 Pa) to form nitride coatings. During the sputtering process, the N+ ion energy was mainly determined by the bias voltage, and the closed filed configuration contributed to the increase of ion density in the plasma. If the bias voltage was too high, coating delamination would be induced due to higher inert stress. If the bias voltage was less than −40 V, the microstructure of coatings would become softer and porous because of the insufficient ion bombardment. Thus, the bias voltage was set as −80 V, and the flow rate of argon was fixed at 50 sccm, while the nitrogen flow rate was controlled by optical emission monitor (OEM) of a constant value of 50% controlled through a piezo-value driven by a computer. Because the substrates were biased, which could attract the ions and then the resulting ion bombardment during coating deposition could cause the temperature rise of the substrates, and there was no extra heater to heat the substrates. A floating thermocouple was placed near the rotating substrate holder. The substrate temperature was approximately 150 °C due to ion bombardment. The chamber pressure applied on the holder was maintained at 0.23 Pa. The sputtering current of Cr and C targets was set as 4 A for each target, while the CrB2 target current varied in the range of 0–4 A to adjust the addition amount of B in the coatings. If the CrB2 target current was higher than 4 A, the CrB2 target began to crack due to overheating caused by higher sputtering power. According to CrB2 target current, the Cr–B–C–N coatings in the next section were denoted as CrCN, CrBCN-1, CrBCN-2, CrBCN-3 and CrBCN-4, respectively. The total depositing time was about one and a half hours, and the detailed parameters of Cr–B–C–N coatings were listed in Table 1.
2.2 Characterization of Cr–B–C–N coatings
The morphology of Cr–B–C–N coatings was examined using a field emission scanning electron microscope (FESEM) (JEOL-JSM-7001F) equipped with an additional EDS (Inca Energy 350, Oxford, UK). The crystallite phase of Cr–B–C–N coatings was identified by X-ray diffraction (XRD, Ultima IV, Japan) with a Cu Kα (λ = 0.15404 nm) radiation source from 20° to 80° at a scanning speed of 5° min−1. The bonding structures of coatings were characterized by X-ray photoelectron spectroscopy (ESCALAB 250, Thermo Scientic, UK) using a Al Kα (1486.6 eV) source at 184 W (10.8 mA and 15.2 kV), and the spot size was about 0.5 mm in diameter, meanwhile, the spectrometer resolution was less than 0.62 eV. The XPS analysis was performed in the depth of 3–10 nm from surface of coatings under a base pressure of 3 × 10−8 Pa by using the Thermo Avantage software. Before measurement, the sample surface was sputtered and etched by Ar ion bombardment for 30 s for surface cleaning. The C1s binding energy line at 284.6 eV was taken as a reference for calibration and the composition was calculated from the peaks areas taking into account the sensitivity factors. In order to get the detailed volume fraction of bonds, Cr 2p, B 1s, C 1s and N 1s fractal spectra were deconvoluted using XPS 4.1 software based on a Lorentzian (20%) and Gaussian (80%) function with nonlinear Shirley-type background.
The hardness and reduced elastic modulus of coatings were measured using a nanoindentor equipped with a Berkovich tip (ENT-1100a, Elionix Co. Ltd., Japan) based on Oliver and Pharr method. Each sample has been measured for 36 times with a penetration depth of 100 nm to ensure the reliability of data and minimize the substrate effect. To assure the reliability of measured data, the corresponding systematic errors were also calculated.
|
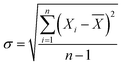 | (1) |
where
σ,
Xi,
![[X with combining macron]](https://www.rsc.org/images/entities/i_char_0058_0304.gif)
and
n are the standard deviation, the
ith data point, the average value of all the
Xi, and the number of data points, respectively. Then, the final results (as shown in
Table 3) could be quoted according to the following equation:
|
 | (2) |
where
α is the confidence level, here
α = 1–0.95, and
tα/2 could be found in the relative reference book.
30
The friction tests of Cr–B–C–N coatings sliding against SiC balls in tap water were conducted using a ball-on-disk tribometer at room temperature. The diameter of SiC ball was 8 mm, and its surface roughness, hardness and elastic modulus were 88.5 nm, 22 GPa and 430 GPa, respectively. During the friction tests, the experiments of Cr–B–C–N/SiC tribopairs at the normal load of 3 N and 6 N were conducted, and the sliding velocity and total sliding length were set at 0.1 m s−1, and 500 m, respectively. The friction tests were carried out three times to ensure the reliability of data, and to get the mean value of friction coefficient and wear rate. Then, the wear scars of SiC balls and the wear tracks of coatings were observed using an optical microscope (XJZ-6, China), while the cross-section area (A) of wear tracks on the coatings was measured using a 3D profilometer (NanoMap-1000WLI, USA). The specific wear rates of SiC balls and Cr–B–C–N coatings were calculated according to the following eqn (1) and (2):
|
 | (3) |
|
 | (4) |
where
R is the radius of SiC ball,
d is the diameter of the wear scar on the ball,
r is the radius of the wear trace on the coating,
F is the normal load and
L is the total sliding distance.
3. Results and discussion
3.1 Composition, microstructure and morphology of Cr–B–C–N coatings
According to the SEM observation and the EDS analysis, the thickness and chemical composition of Cr–B–C–N coatings are listed in Table 2. It is obvious that the thickness of coatings increased gradually from 1.40 μm to 1.61 μm as the CrB2 target current varied from 0 A to 4 A. This indicated that the deposition rate of coatings increased with an increase in the CrB2 target current. When the CrB2 target current increased from 0 A to 1 A, the B concentration increased sharply to 24.6 at%, while the concentrations of Cr, C and N elements all decreased rapidly. With further increasing the CrB2 target current from 1 A to 4 A, the B element content increased slightly, while the concentrations of Cr, C and N elements all fluctuated slightly. Fig. 1 shows the XRD patterns of Cr–B–C–N coatings deposited at the different CrB2 target currents. As seen in Fig. 1, all of the coatings consisted of three peaks centered at 37.5°, 44.5° and 69°. The diffraction peak at 69° was assigned to the Si(100) substrate, while the diffraction peaks at 37.5° and 44.5° were related to CrN/Cr2N(111) and Cr(110)/CrN/Cr2N(200) (JCPDS 11-0065, JCPDS 19-0323), respectively, and the Cr(110) was thought to be originated from Cr interlayer.31 With increasing CrB2 target current, the diffraction peak at 37.5° became broad with a decreasing intensity. Furthermore, no diffraction peaks related to borides were detected in the XRD pattern, this indicated that the more incorporation of B element could inhibit the crystalline growth of CrN/Cr2N(111), and the incorporated B might exist as the form of amorphous phase.
Table 2 Thickness and element concentration of Cr–B–C–N coatings deposited at different CrB2 target currents
Coatings |
Thickness (μm) |
B (at%) |
C (at%) |
N (at%) |
Cr (at%) |
CrCN |
1.40 |
0 |
29.8 |
26.5 |
43.7 |
CrBCN-1 |
1.50 |
24.6 |
20.4 |
20.6 |
34.4 |
CrBCN-2 |
1.50 |
24.9 |
22.0 |
19.8 |
33.3 |
CrBCN-3 |
1.59 |
25.1 |
20.9 |
19.1 |
34.9 |
CrBCN-4 |
1.61 |
27.2 |
22.7 |
18.0 |
32.1 |
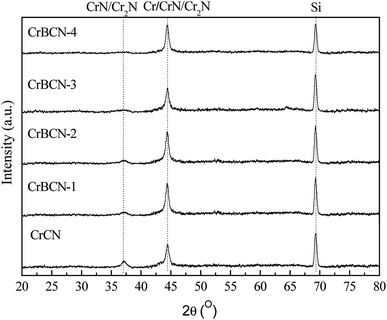 |
| Fig. 1 X-ray diffraction spectra of Cr–B–C–N coatings deposited at different CrB2 currents. | |
Fig. 2 shows XPS spectra of Cr 2p, B 1s, C 1s and N 1s for Cr–B–C–N coatings deposited at different CrB2 target currents, while Fig. 3 shows their corresponding fitted results. As seen in Fig. 2a, the Cr 2p spectra was deconvoluted into six peaks, which located at 574.4 eV, 575.3 eV, 576.4 eV, 577.8 eV, 584.6 eV and 586.4 eV, respectively. The peak located at 574.4 eV was assigned to Cr–Cr bonds, while the peaks located at 575.3 eV and 576.4 eV were originated from Cr–N bonds.32–34 The peaks at 577.8 eV, 684.6 eV and 586.4 eV were attributed to Cr–O bonds, this is because that although the deposition chamber pressure was pumped to as low as 4 × 10−4 Pa before deposition, there was still residual oxygen in chamber and that would react with composition elements of Cr–B–C–N coatings.26 As seen in Fig. 3a, the volume fractions of Cr–Cr and Cr–O bonds both increased to 20.1% and 46.3%, while the volume fraction of Cr–N bonds decreased to 33.6% when the CrB2 target current increased to 3 A. But as the CrB2 target current was 4 A, the volume fraction of Cr–Cr bonds decreased to 17.2%, while the volume fraction of Cr–N bonds increased to 36.4%. This indicated that the critical CrB2 target current for the transformation of bonding structure was 3 A. As seen in Fig. 2b, the B 1s spectra could be deconvoluted into three peaks centered at 189.5 eV, 190.2 eV and 192.3 eV, which were corresponded to B–C, B
N and B–O bonds, respectively.35–38 When the CrB2 target current increased from 1 A to 4 A, the volume fraction of B–C bonds decreased from 24.3% to 5.2%, while that of B–N bonds increased from 55.5% to 81.0% (Fig. 3b). This pointed out that the incorporated B was prone to react with N than C, and then the amorphous BN was gradually formed in Cr–B–C–N coatings. It is clear in Fig. 2c that the deconvoluted C 1s spectra consisted of three peaks located at 284.7 eV, 286.1 eV and 288.3 eV. The dominant peak at 284.7 eV was assigned to sp2C–N/sp2C–C bonds, while the peak located at 286.1 eV was corresponded to sp3C–N/sp3C–C bonds.39–41 Moreover, the peak at 288.3 eV was contributed to C–O bonds.42 When CrB2 target current was beyond 1 A, a new peak appeared at 283.5 eV, which was attributed to C–B bonds.43 When the CrB2 target current was 0 A, C atoms were mostly existed as sp2C–N/sp2C–C bonds with the corresponding volume fraction of 94.9%, which was expected to present a low friction coefficient. When the CrB2 target current varied in the range of 1 A to 4 A, the volume fraction of sp2C–N/sp2C–C bonds fluctuated around 77%, whilst the volume fractions of sp3C–N/sp3C–C and C–B bonds increased to 8.0% and 5.0% (Fig. 3c), which were beneficial to increasing the hardness of Cr–B–C–N coatings. As seen in Fig. 2d, all N 1s spectra consisted of three peaks which were located at 396.8 eV, 398.2 eV and 399.3 eV, respectively. The dominant peak located at 396.8 eV was attributed to N–Cr bonds, and the peak located at 399.3 eV was attributed to N–C bonds.44,45 According to ref. 46 and 47, the peak at 398.2 eV was assigned to N–C and N–B bonds for CrCN coatings and CrBCN coatings accordingly. Based on the above analysis, it is known that the CrCN coatings mainly consisted of Cr–N and C
C bonds, but when the CrB2 target current was beyond 1 A, the incorporated boron atoms mainly existed as B–N bonds, then the CrBCN coatings mainly contained Cr–N, B–N, C–N and B–C bonds.
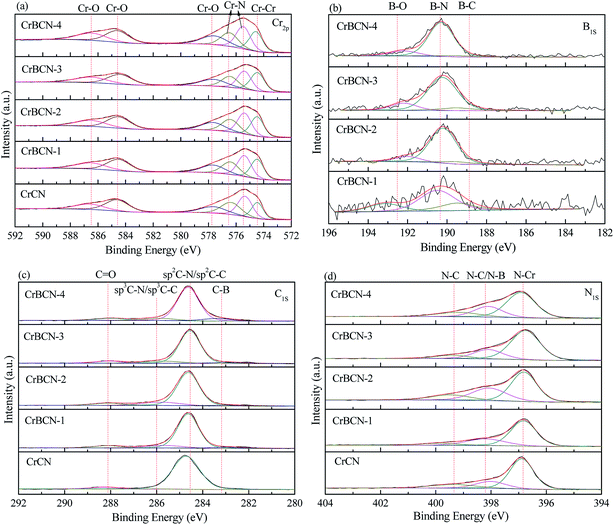 |
| Fig. 2 XPS spectra of Cr–B–C–N coatings deposited at different CrB2 target currents. | |
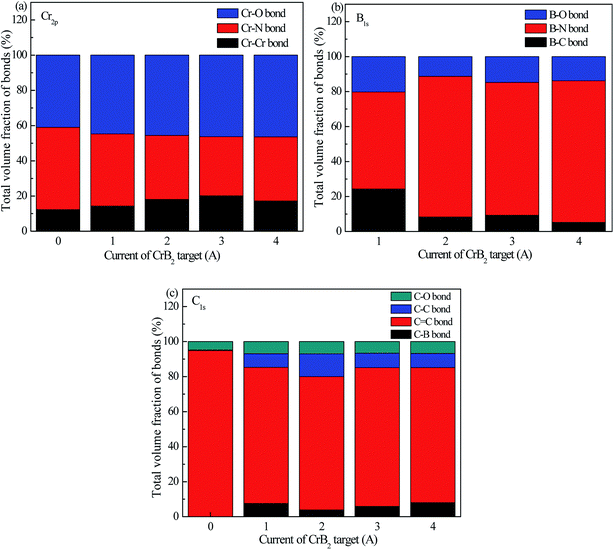 |
| Fig. 3 XPS analysis results of B1s (a), N1s (b) and C1s (c) of Cr–B–C–N coatings at different CrB2 target currents. | |
The surface topographies and the corresponding surface roughness of Cr–B–C–N coatings deposited at different CrB2 target currents are shown in Fig. 4. As seen in Fig. 4a, the surface of CrCN coatings exhibited a clear cauliflower-like morphology and the nanograins were easily distinguished. It was reported that the grain size growth of nanocomposite nitride coatings could be limited when the carbon, silicon or boron was incorporated.31,48 As a result, with an increase in the CrB2 target current, the coatings surface became more compact resulting from a decreasing grain size of cauliflower-like particle. The corresponding average surface roughness (Ra) of CrCN and CrBCN-1 coatings were around 31 nm, with an increasing in the CrB2 target current to 4 A, the surface roughness decreased monotonically to 16 nm at 4 A. Fig. 5 shows the cross-sectional micrographs of Cr–B–C–N coatings, it is obvious that all coatings consisted of Cr interlayer and Cr–B–C–N top layer, and the CrCN coating exhibited typical columnar structure. When the CrB2 target current varied in the range of 1 A to 4 A, the typical columnar structure gradually disappeared. It is deduced that the formation of amorphous phase would interrupt the columnar growth and then the coatings structure became dense.31
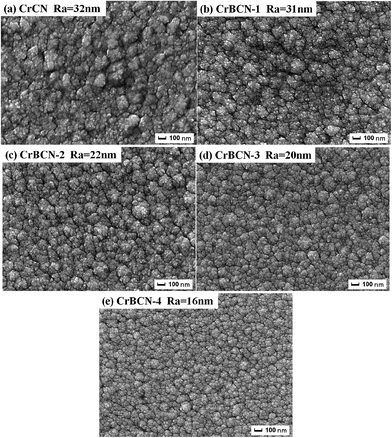 |
| Fig. 4 SEM images of topography and the corresponding surface roughness of Cr–B–C–N coatings. | |
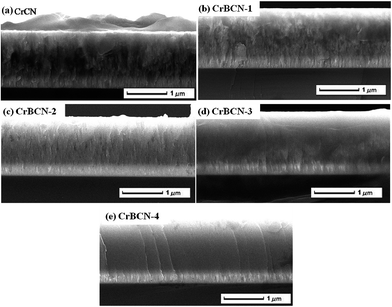 |
| Fig. 5 Cross-sectional microstructure of Cr–B–C–N coatings deposited at different CrB2 target currents. | |
3.2 Mechanical properties of Cr–B–C–N coatings
The hardness (H) and reduced elastic modulus (E) of Cr–B–C–N coatings deposited at different CrB2 target currents are tabulated in Table 3. As seen in Table 3, the hardness and elastic modulus of CrCN coating were 17.7 ± 1.03 GPa and 258 ± 9.59 GPa, respectively. When the CrB2 target current varied in the range of 1–2 A, the hardness of Cr–B–C–N coatings increased slightly to 17.9 ± 0.79 GPa and 18.6 ± 0.86 GPa, respectively. On the one hand, the increasing grain boundary intensities with finer grain size would increase the resistance of dislocation glide, which made a contribution to the increase in hardness of Cr–B–C–N coatings.45 On the other hand, solid solution effect also contributed to the increase of hardness.28 However, the gradual formation of soft amorphous BN in CrBCN coatings deposited at the CrB2 target current of 3 A and 4 A, led to a decrease in the hardness, and then the hardness of CrBCN-4 coating dropped to 16.3 ± 0.28 GPa eventually. The elastic modulus of CrBCN coatings also increased slightly to 261 ± 10.40 GPa when the current of CrB2 target increased to 2 A at first, and then decreased to 228 ± 2.53 GPa with further increasing the CrB2 target current to 4 A. As is known, the plasticity index (H/E) and the plastic deformation resistance (H3/E2) provide a close correlation with the wear resistance of coatings.39,49 As seen in Table 3, the values of H/E and H3/E2 increased monotonously when the current of CrB2 target increased from 0 A to 3 A, and the maximum values of H/E (0.079) and H3/E2 (0.113) were all obtained at the CrB2 target current of 3 A. When the current of CrB2 target reached 4 A, the values of H/E and H3/E2 both decreased. Thus, the CrBCN-3 coating is expected to present a superior tribological performance in water.
Table 3 Hardness, elastic modulus, H/E and H3/E2 of Cr–B–C–N coatings deposited at different CrB2 target currents
Coatings |
Hardness, H (GPa) |
Elastic modulus, E (GPa) |
H/E |
H3/E2 (GPa) |
CrCN |
17.7 ± 1.03 |
258 ± 9.59 |
0.068 |
0.083 |
CrBCN-1 |
17.9 ± 0.79 |
256 ± 7.37 |
0.070 |
0.088 |
CrBCN-2 |
18.6 ± 0.86 |
261 ± 10.40 |
0.071 |
0.094 |
CrBCN-3 |
18.2 ± 0.56 |
231 ± 4.89 |
0.079 |
0.113 |
CrBCN-4 |
16.3 ± 0.28 |
228 ± 2.53 |
0.071 |
0.083 |
3.3 Tribological properties of Cr–B–C–N coatings sliding against SiC balls in tap water
Fig. 6 shows the friction behaviors of Cr–B–C–N coatings sliding against SiC balls at 3 N and 6 N in tap water, and Fig. 7 shows the contact pressure of Cr–B–C–N/SiC tribopairs at 3 N and 6 N. As seen in Fig. 6a, the initial friction coefficient of the CrCN/SiC tribopairs at 3 N was 0.24, higher than those of all CrBCN/SiC tribopairs. In principle, the initial friction coefficient is proportional to the original contact surface roughness, and the highest initial friction coefficient of CrCN/SiC tribopairs was well consistent with the coarse columnar structure of CrCN coatings (Fig. 4a). In addition, the initial friction coefficient was also closely related to the initial contact pressure. According to Hertzian's ball-on-disk mode, the initial contact pressures for the Cr–B–C–N/SiC tribopairs were calculated. As shown in Fig. 7a, the CrCN/SiC tribopair showed the highest initial contact pressure of 0.69 GPa (Fig. 7a), which also contributed to the higher initial friction coefficient of CrCN/SiC tribopairs. With increasing the sliding distance, the friction coefficient of the CrCN/SiC tribopairs decreased monotonously to 0.18 within 102 m, and then increased gradually to 0.24. When the B element was incorporated into the CrCN coatings, the variation of friction coefficient for the CrBCN/SiC tribopairs was dependent on the CrB2 target current. Specifically, the friction coefficient of CrBCN-1/SiC tribopairs increased from 0.17 to 0.2 within the first 32 m, while the friction coefficient of CrBCN-2/SiC tribopairs increased from 0.18 to 0.2 within 101 m. When the CrB2 target current increased to 3 A, the CrBCN-3/SiC tribopairs exhibited the lowest friction coefficient of 0.15 and the friction coefficient fluctuated around 0.16. When the CrB2 target current reached 4 A, the friction coefficient of CrBCN-4/SiC tribopairs decreased from 0.2 to 0.18 within the first 9 m, and then increased to 0.23 when the sliding distance increased to 163 m. Finally, the friction coefficient increased slightly to 0.25. After the friction tests, the ultimate contact pressure could be calculated by using the formula P = F/S, where F is the normal load and S is the wear contact area of mating balls. As seen in Fig. 7a, the ultimate contact pressure ranged from 0.031 GPa to 0.074 GPa. In order to know the tribological properties of Cr–B–C–N coatings at higher loads, the friction tests sliding against SiC balls at 6 N were conducted in tap water, and the corresponding friction behavior is showed in Fig. 6b. As seen in Fig. 6b, the friction behavior of CrCN and CrBCN-1 coatings showed the similar tendency, increased during the running-in period, and then decreased in the next 40 m and 80 m, respectively. With further sliding to 500 m, the friction coefficient showed an increasing tendency, while CrBCN-2, CrBCN-3 and CrBCN-4 coatings fluctuated slightly around 0.15 during the whole sliding process. As seen in Fig. 7b, the initial contact pressures for Cr–B–C–N/SiC tribopairs under 6 N ranged from 0.83 GPa to 0.87 GPa. After the friction tests, the ultimate contact pressures varied from 0.45 GPa to 0.107 GPa.
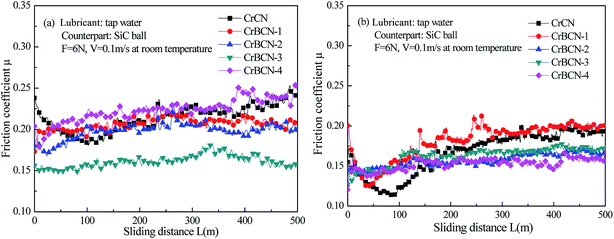 |
| Fig. 6 Influence of CrB2 target currents on the friction behavior of Cr–B–C–N/SiC tribopairs at the normal load of 3 N (a) and 6 N (b) in tap water. | |
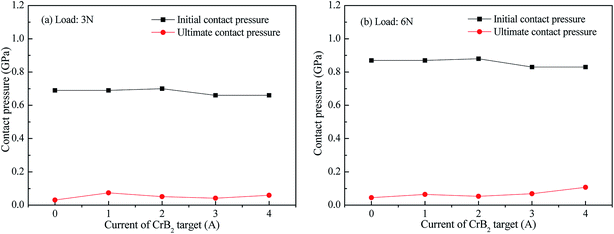 |
| Fig. 7 Contact pressure of the Cr–B–C–N/SiC tribopairs at 3 N (a) and 6 N (b) in tap water. | |
Fig. 8 shows the variation of the friction coefficient and specific wear rates for the Cr–B–C–N/SiC tribopairs at the normal load of 3 N with the CrB2 target current. As seen in Fig. 8a, with an increase in the CrB2 target current, the mean-steady friction coefficient of Cr–B–C–N/SiC tribopairs first decreased from 0.21 to 0.16 at the CrB2 target current of 3 A, and then increased to 0.22. This indicated that the B incorporation could improve the lubrication properties of coatings to a certain extent. As is known, the specific wear rate of coatings is closely related to the friction coefficient and hardness of coatings. As seen in Fig. 8b, the specific wear rate of CrCN coatings was 5.7 × 10−7 mm3 N−1 m−1, while the specific wear rate of CrBCN-1 coatings was 3.9 × 10−7 mm3 N−1 m−1. With further increasing the CrB2 target current to 3 A, the specific wear rate of CrBCN coatings first increased to 4.1 × 10−7 mm3 N−1 m−1, and then declined to the lowest value of 2.1 × 10−7 mm3 N−1 m−1. When the CrB2 target current was 4 A, the specific wear rate of CrBCN-4 coating increased quickly to 6.3 × 10−7 mm3 N−1 m−1. It was obvious that the CrBCN coatings deposited at the CrB2 target current of 3 A exhibited the best tribological properties in water. Moreover, due to the protection of transferred films from coatings to SiC balls, the specific wear rates of SiC balls were all lower than those of Cr–B–C–N coatings.39 Fig. 9 shows the mean-steady friction coefficient of Cr–B–C–N/SiC tribopairs at the normal load of 6 N. As seen in Fig. 9, it is clear that the friction coefficients of Cr–B–C–N/SiC tribopairs at 3 N were higher than those at 6 N. As compared with the CrCN and CrBCN-1 coatings deposited at the CrB2 target current of 0 A and 1 A, the Cr–B–C–N coatings deposited at the CrB2 target current beyond 1 A showed a lower friction coefficient (∼0.15), this phenomena indicated that incorporated boron could still play a role in decreasing friction coefficient at the normal load of 6 N.
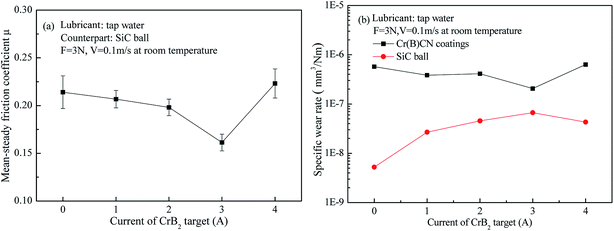 |
| Fig. 8 Influence of CrB2 target currents on mean-steady friction coefficient (a) and specific wear rate (b) of Cr–B–C–N/SiC tribopairs at the normal load of 3 N in tap water. | |
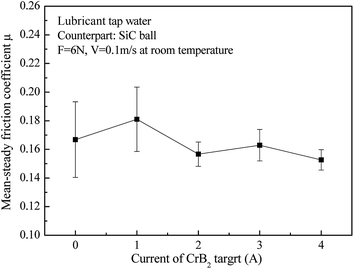 |
| Fig. 9 Influence of CrB2 target currents on mean-steady friction coefficient of Cr–B–C–N/SiC tribopairs at the normal load of 6 N in tap water. | |
The cross-sectional profiles and optical images with the wear width of the wear tracks on the Cr–B–C–N coatings at the normal load of 3 N are illustrated in Fig. 10 and 11. As seen in Fig. 10a and 11a, the depth and width of wear track on the CrCN coatings were higher, and some adhering wear debris could be observed on the wear track, meanwhile, there were some grooves parallel to the sliding direction because of abrasive wear. When the CrB2 target current increased from 1 A to 3 A, the depth and width of wear track increased firstly and then decreased (Fig. 10b–d), and the shallowest and narrowest wear track was observed on the Cr–B–C–N coatings deposited at the CrB2 target current of 3 A (Fig. 10d and 11d). But when the CrB2 target current was 4 A, the wear track on the CrBCN-4 coatings became deeper and wider. As compared with the CrCN coatings, a significant wear could be observed on the wear track of CrBCN-4 coatings (Fig. 10e). It is necessary to indicate that when the CrB2 target current was beyond 0 A, there were fewer grooves on the wear tracks of coatings, and the wear surface became very smooth, thus, the wear mechanism was mainly tribochemical wear. Fig. 12 shows the SEM images of the wear tracks and the corresponding EDS analyses of the Cr–B–C–N coatings at 3 N. As seen in Fig. 12, the wear track of the CrCN coatings was wide and only Cr, C and N elements appeared in the EDS analysis, while the wear tracks of the CrBCN-1, CrBCN-3 and CrBCN-4 coatings were shallower, and also only Cr, C and N elements were found in the EDS analyses. Furthermore, a clear partial delamination was observed on the wear track of CrBCN-2 coatings (Fig. 12e), which was proved by the Fe peaks in the EDS analysis (Fig. 12f). Thus, although the CrBCN-2 coatings exhibited lower friction coefficient in comparison to the CrBCN-1 coatings, the corresponding specific wear rate was higher.
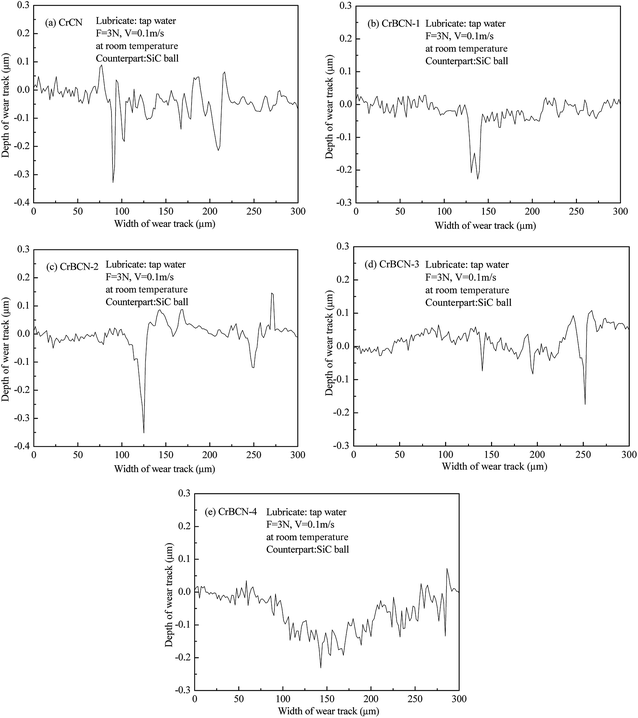 |
| Fig. 10 Influence of CrB2 target currents on the cross sectional profiles of wear track on Cr–B–C–N coatings sliding against SiC balls at 3 N in tap water. | |
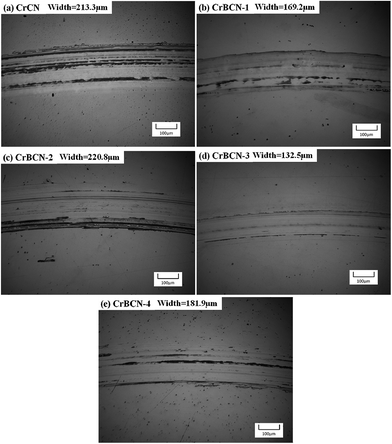 |
| Fig. 11 Optical images of wear track for Cr–B–C–N coatings sliding against SiC balls at the normal load of 3 N in tap water. | |
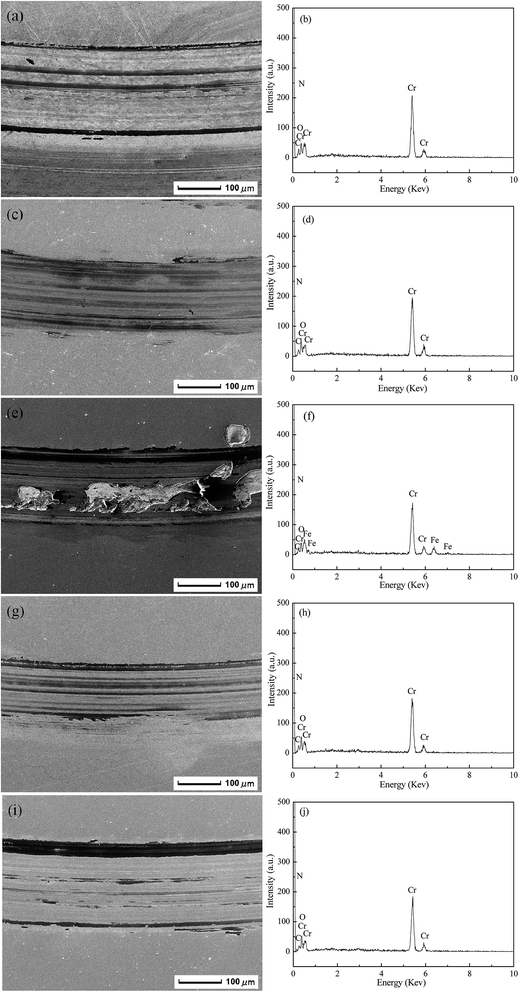 |
| Fig. 12 SEM images and corresponding EDS analysis of wear track for CrCN (a and b), CrBCN-1 (c and d), CrBCN-2 (e and f), CrBCN-3 (g and h), CrBCN-4 (i and j) coatings sliding against SiC balls at the normal load of 3 N. | |
The optical images with the wear width of the wear tracks on the Cr–B–C–N coatings at the normal load of 6 N are illustrated in Fig. 13. As seen in Fig. 13a, the widths of wear tracks of CrCN and CrBCN-1 coatings were 354.1 μm, and there were obvious abrasive grooves on the wear track. However, when the CrB2 target current was in the range of 1–4 A, a serious delamination could be observed on the wear track (Fig. 13b–e), and this failure might be caused by relatively high inert stress and inadequate adhesive strength between CrBCN coatings and substrates.23 Thus, although the incorporated boron could still contribute to lowering the friction coefficient of Cr–B–C–N/SiC tribopairs at higher loads (Fig. 9), the wear resistance of CrBCN coatings was poor.
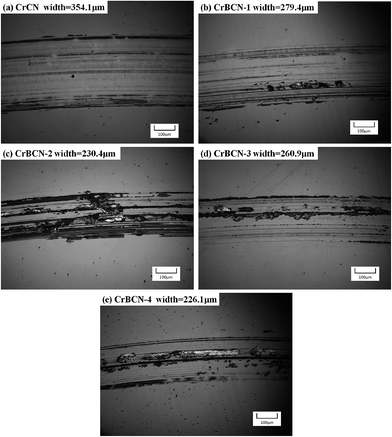 |
| Fig. 13 Optical images of wear track for Cr–B–C–N coatings sliding against SiC balls at the normal load of 6 N in tap water. | |
3.4 Discussion
As is known, the tribological performances of coatings were strongly determined by the coating composition, bonding structures and the formation of tribo-layers. By increasing the CrB2 target current from 0 A to 4 A, the boron concentration of Cr–B–C–N coatings increased monotonically from 0 to 27.2%, while the concentrations of Cr, C and N elements all decreased sharply when the CrB2 target current increased to 1 A, and then fluctuated slightly with further increasing CrB2 target. Based on the analyses of XRD and XPS in Fig. 1 and 2, the incorporated B mainly existed as amorphous B–N, B–O and B–C bonds. When the CrCN coating slid against SiC ball at the normal load of 3 N in tap water, the initial contact pressure was in the range of 0.66–0.70 GPa, and the tribochemical reactions between tribomaterials and water could occur easily and then there were a few grooves on the wear track of CrCN coating (Fig. 11). When the CrB2 target current increased to 1–4 A, fewer grooves could be observed on the worn surfaces of coatings. As seen in Fig. 14a, the logarithmic value of specific wear rate increased linearly with the friction coefficient, which was expressed as: |
ln(ws,d) = −18.3 + 17.9μm
| (5) |
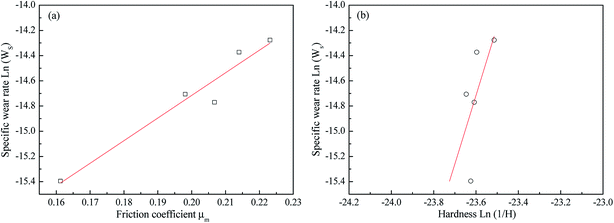 |
| Fig. 14 (a) Logarithmic relationship of specific wear rate against friction coefficient (μm), (b) logarithmic relationship between specific wear rate and 1/H (H is the hardness of coatings). | |
This indicated that the abrasive wear was a main wear mechanism for Cr–B–C–N coatings. However, when the CrB2 target current was beyond 1 A, the wear tracks on Cr–B–C–N coatings became smooth and flat in Fig. 11b–e. Thus, besides abrasive wear, the dominated wear mechanism for the Cr–B–C–N coatings also included tribochemical wear, and the transfer layer (Cr2O3, hydrated Si(OH)4 and B(OH)3) was formed at the sliding interface. The tribochemical reactions of CrCN coating and CrBCN coatings with water occurred as follows:26,40
|
SiC + H2O → Si(OH)4 + CH4
| (6) |
|
CrxCNy + H2O → Cr2O3 + NH3 + CH4
| (7) |
|
CrxBCyNz + H2O → (NH4)H2BO3 + Cr2O3 + CO2
| (8) |
It is clear that Si(OH)4 gels and H3BO3 formed on the wear surface acting as a lubricating layer, while Cr2O3 particles played a role of polishing. Moreover, a small amount of Ca2+ and Mg2+ in tap water could form lubricating CaCO3 and Mg(OH)2 on the sliding interface.50,51 As seen in Fig. 8, with increasing CrB2 target current to 3 A, the friction coefficients and the specific wear rates of Cr–B–C–N/SiC tribopairs decreased from 0.21 and 5.7 × 10−7 mm3 N−1 m−1 to 0.16 and 2.1 × 10−7 mm3 N−1 m−1, respectively. This phenomenon might be attributed to the lubrication effect of reaction product of borides and water. Sanchez et al.52 indicated that there was a lubricant layer at contact area which was assumed to boron acid (H3BO3). Since the lubricating effect of boron acid (H3BO3) film with layered-crystal structure on the sliding surface, the lubrication condition was improved effectively, and then the friction coefficient and wear rate of Cr–B–C–N/SiC tribopairs decreased.53–57 In principle, the specific wear rate of coatings is closely related to their mechanical properties. According to Archard's equation,58 the specific wear rates of coatings were influenced by their hardness. As seen in Fig. 14b, the logarithmic values of specific wear rate and 1/H displayed a linear relationship as:
|
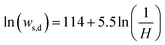 | (9) |
It is obvious that the specific wear rates of coatings decreased rapidly with the increase of hardness. As a result, the specific wear rates of Cr–B–C–N/SiC tribopairs were lower when the current of CrB2 target varied in the range of 2–3 A, because the hardness of CrBCN-2 and CrBCN-3 coatings was relatively higher compared to the rest coatings. In particular, CrBCN-3 showed the best wear resistance due to its lower friction coefficient and better mechanical properties. Furthermore, CrBCN-3 coating exhibited the maximum values of H/E and H3/E2, which contributed to its strong wear resistance as well. However, when the Cr–B–C–N coatings slid against SiC ball at 6 N, the initial contact pressure varied in the range of 0.83 to 0.88 GPa, and the wear resistance was poor (Fig. 13) due to relatively lower load-bearing capacity in water environment.59 Thus, although incorporated B still played a role in lowering friction coefficient at higher loads, the wear resistance of CrBCN coatings was poor due to inadequate adhesive strength to substrates, and the delamination became the main wear mechanism.
4. Conclusions
The Cr–B–C–N coatings with different boron contents were deposited on Si(100) wafers and 316L stainless steels using unbalanced magnetron sputtering system by way of adjusting the CrB2 target currents, and their microstructure, mechanical and tribological properties of Cr–B–C–N coatings in water have been investigated, and the results are summarized as follows:
(1) With increasing the CrB2 target current to 4 A, the boron content of Cr–B–C–N coatings increased from 0 at% to 27.2 at%, and the incorporated boron mainly existed as soft a-BN phases.
(2) When the CrB2 target current varied in the range of 0–3 A, the hardness of Cr–B–C–N coatings fluctuated around 18 GPa, but the CrBCN-3 coatings' elastic modulus decreased to 231 ± 4.89 GPa. With further increasing the CrB2 target current to 4 A, the hardness and elastic modulus of coatings both decreased slightly.
(3) When the normal load was 3 N, the lowest friction coefficient of 0.16 and the lowest of specific wear rate of 2.1 × 10−7 mm3 N−1 m−1 were obtained simultaneously for the CrBCN-3/SiC tribopairs. The wear mechanism of Cr–B–C–N coatings was mainly tribochemical wear and abrasive wear.
(4) When the normal load increased to 6 N, the wear resistance of Cr–B–C–N coatings was poor due to the serious delaminations of coatings.
Acknowledgements
This work was supported by National Natural Science Foundation of China (Grant No. 51375231), The Research Fund for the Doctoral Program of Higher Education (Grant No. 20133218110030). Foundation of Graduate Innovation Center in NUAA (kfjj20160512). A Project Funded by Priority Academic Program Development of Jiangsu Higher Education Institutions (PAPD). We would like to acknowledge them for their financial support.
References
- D. Martínez-Martínez, L. Kolodziejczyk, J. C. Sánchez-López and A. Fernández, Tribological carbon-based coatings: an AFM and LFM study, Surf. Sci., 2009, 603(7), 973–979 CrossRef.
- Q. Z. Wang, F. Zhou, C. D. Wang, M. F. Yuen, M. L. Wang, T. Qian, M. Matsumoto and J. W. Yan, Comparison of tribological and electrochemical properties of TiN, CrN, TiAlN and a-C: H coatings in simulated body fluid, Mater. Chem. Phys., 2015, 158(5), 74–81 CrossRef CAS.
- R. Hauert, A review of modified DLC coatings for biological applications, Mater. Chem. Phys., 2003, 12(3–7), 583–589 CAS.
- W. Dai, P. L. Ke and A. Y. Wang, Microstructure and property evolution of Cr-DLC films with different Cr content deposited by a hybrid beam technique, Vacuum, 2011, 85(8), 792–797 CrossRef CAS.
- F. Zhou, K. Adachi and K. Kato, Sliding friction and wear property of a-C and a-CNx coatings against SiC balls in water, Thin Solid Films, 2006, 514(1–2), 231–239 CrossRef CAS.
- Y. J. Wang, H. X. Li, X. H. Liu, L. Ji, Y. X. Wu, Y. H. Lv, Y. Y. Fu, H. D. Zhou and J. M. Chen, Friction and wear properties of graphite-like carbon films deposited on different substrates with a different interlayer under high Hertzian contact stress, Tribol. Lett., 2012, 46(3), 243–254 CrossRef CAS.
- Y. Tanno and A. Azushim, Effect of counter materials on coefficients of friction of TiN coatings with preferred grain orientations, Wear, 2009, 266(11–12), 1178–1184 CrossRef CAS.
- S. Wilson and A. T. Alpas, Dry sliding wear of a PVD TiN coating against Si3N4 at elevated temperatures, Surf. Coat. Technol., 1996, 86–87(1), 75–81 CrossRef CAS.
- I. Dreiling, C. Raisch, J. Glaser, D. Stiens and T. Chassé, Characterization and oxidation behavior of MTCVD Ti–B–N coatings, Surf. Coat. Technol., 2011, 206(2–3), 479–486 CrossRef CAS.
- P. K. P. Rupa, P. C. Chakraborti and S. K. Mishra, Structure and indentation behavior of nanocomposite Ti–B–N films, Thin Solid Films, 2014, 564, 160–169 CrossRef CAS.
- I. Dreiling, C. Raisch, J. Glaser, D. Stiens and T. Chassé, Temperature dependent tribooxidation of Ti–B–N coatings studied by Raman spectroscopy, Wear, 2012, 288, 62–71 CrossRef CAS.
- T. Polcar, T. Kubart, R. Novák, L. Kopecký and P. Široký, Comparison of tribological behaviour of TiN, TiCN and CrN at elevated temperatures, Surf. Coat. Technol., 2005, 193(1–3), 192–199 CrossRef CAS.
- T. Polcar, R. Novák and P. Široký, The tribological characteristics of TiCN coating at elevated temperatures, Wear, 2006, 260(1–2), 40–49 CrossRef CAS.
- L. F. Senn, C. A. Achetea, T. Hirsch and F. L. Freire, Structural, chemical, mechanical and corrosion resistance characterization of TiCN coatings prepared by magnetron sputtering, Surf. Coat. Technol., 1997, 91–95, 390–397 CrossRef.
- K. H. Kim, J. T. Ok, S. Abraham, Y. R. Ch, I. W. Park and J. J. Moore, Syntheses and mechanical properties of Ti–B–C–N coatings by a plasma-enhanced chemical vapor deposition, Surf. Coat. Technol., 2006, 201(7), 4185–4189 CrossRef CAS.
- J. L. Lin, J. J. Moore, B. Mishra, M. Pinkas and W. D. Sproul, The structure and mechanical and tribological properties of TiBCN nanocomposite coatings, Acta Mater., 2010, 58(5), 1554–1564 CrossRef CAS.
- A. Vyas, Y. H. Lu and Y. G. Shen, Mechanical and tribological properties of multicomponent Ti–B–C–N thin films with varied C contents, Surf. Coat. Technol., 2010, 204(9–10), 1528–1534 CrossRef CAS.
- J. L. Lin, N. Y. Zhang, W. D. Sproul and J. J. Moore, A comparison of the oxidation behavior of CrN films deposited using continuous dc, pulsed dc and modulated pulsed power magnetron sputtering, Surf. Coat. Technol., 2012, 206(14), 3283–3290 CrossRef CAS.
- J. L. Lin, W. D. Sproul and J. J. Moore, Tribological behavior of thick CrN coatings deposited by modulated pulsed power magnetron sputtering, Surf. Coat. Technol., 2012, 206(8–9), 2474–2483 CrossRef CAS.
- Y. C. Chim, X. Z. Ding, X. T. Zeng and S. Zhang, Oxidation resistance of TiN, CrN, TiAlN and CrAlN coatings deposited by lateral rotating cathode arc, Thin Solid Films, 2009, 517(17), 4845–4849 CrossRef CAS.
- Z. W Wu, F. Zhou, Q. Z Wang, Z. F. Zhou, J. W. Yan and L. K. Y Li, Influence of trimethylsilane flow on the microstructure, mechanical and tribological properties of CrSiCN coatings in water lubrication, Appl. Surf. Sci., 2015, 355, 516–530 CrossRef.
- F. Zhou, K. M. Chen, M. L. Wang, X. J. Xu, H. Meng, M. Yu and Z. D. Dai, Friction and wear properties of CrN coatings sliding against Si3N4 balls in water and air, Wear, 2008, 265(7–8), 1029–1037 CrossRef CAS.
- P. F. Hu and B. L. Jiang, Study on tribological property of CrCN coating based on magnetron sputtering plating technique, Vacuum, 2011, 85(11), 994–998 CrossRef CAS.
- J. W. Lee, C. H. Cheng, H. W. Chen, Y. C. Chand, J. G. Duhd and L. W. Ho, Effects of boron and nitrogen contents on the microstructures and mechanical properties of Cr–B–N nanocomposite thin films, Procedia Eng., 2012, 36, 360–367 CrossRef CAS.
- Y. W. Ye, Y. X. Wang, H. Chen, J. L. Li, Y. R. Yao and C. T. Wang, Doping carbon to improve the tribological performance of CrN coatings in seawater, Tribol. Int., 2015, 90, 362–371 CrossRef CAS.
- Q. Z. Wang, F. Zhou, X. D. Ding, Z. F. Zhou, C. D. Wang, W. J. Zhang, L. K. Y. Li and S. T. Lee, Microstructure and water-lubricated friction and wear properties of CrN(C) coatings with different carbon contents, Appl. Surf. Sci., 2013, 268, 579–587 CrossRef CAS.
- C. H. Cheng, J. W. Lee, L. W. Ho, H. W. Chen, Y. C Chan and J. G. Duh, Microstructure and mechanical property evaluation of pulsed DC magnetron sputtered Cr–B and Cr–B–N films, Surf. Coat. Technol., 2011, 206(7), 1711–1719 CrossRef CAS.
- G. A. Zhang, L. P. Wang, P. X. Yan and Q. J. Xue, Structure and mechanical properties of Cr–B–N films deposited by reactive magnetron sputtering, J. Alloys Compd., 2009, 486(1–2), 227–232 CrossRef CAS.
- C. Higdon, B. Cook, J. Harringa, A. Russell, J. Goldsmith, J. Qu and P. Blau, Friction and wear mechanisms in AlMgB14–TiB2 nanocoatings, Wear, 2011, 271(9), 2111–2115 CrossRef CAS.
- Z. Sheng, S. Q. Xie and C. Y. Pan, Probability theory and mathematical statistics, Higher Education Press, Beijing, 4th edn, 2008 Search PubMed.
- J. W. Lee, C. H. Cheng, H. W. Chen, L. W. Ho, J. G. Duh and Y. C. Chan, The influence of boron contents on the microstructure and mechanical properties of Cr–B–N thin films, Vacuum, 2013, 87, 191–194 CrossRef CAS.
- J. L. Lin, W. D. Sproul, J. J. Moore, S. Lee and S. Myers, High rate deposition of thick CrN and Cr2N coatings using modulated pulse power (MPP) magnetron sputtering, Surf. Coat. Technol., 2011, 205(10), 3226–3234 CrossRef CAS.
- Y. Birol, Sliding wear of CrN, AlCrN and AlTiN coated AISI H13 hot work tool steels in aluminium extrusion, Tribol. Int., 2013, 57, 101–106 CrossRef CAS.
- H. C. Barshilia, N. Selvakumar, B. Deepthi and K. S. Rajam, A comparative study of reactive direct current magnetron sputtered CrAlN and CrN coatings, Surf. Coat. Technol., 2006, 201(16), 2193–2201 CrossRef CAS.
- X. R. Deng, H. Kousaka, T. Tokoroyama and N. Umehara, Deposition and tribological behaviors of ternary BCN coatings at elevated temperatures, Surf. Coat. Technol., 2014, 259, 2–6 CrossRef CAS.
- Q. Yang, C. B. Wang, S. Zhang, D. M. Zhang, Q. Shen and L. M. Zhang, Effect of nitrogen pressure on structure and optical properties of pulsed laser deposited BCN thin films, Surf. Coat. Technol., 2010, 204(11), 1863–1867 CrossRef CAS.
- P. C. Tsai, W. J. Chen, J. H. Chen and C. L. Chang, Deposition and characterization of TiBCN films by cathodic arc plasma evaporation, Thin Solid Films, 2009, 517(17), 5044–5049 CrossRef CAS.
- K. P. Budna, J. Neidhardt, P. H. Mayrhofer and C. Mitterer, Synthesis–structure–property relations for Cr–B–N coatings sputter deposited reactively from a Cr–B target with 20 at% B, Vacuum, 2008, 82(8), 771–776 CrossRef CAS.
- Z. W. Wu, F. Zhou, K. M. Chen, Q. Z. Wang, Z. F. Zhou, J. W. Yan and L. K. Y. Li, Friction and wear properties of CrSiCN coatings with low carbon content as sliding against SiC and steel balls in water, Tribol. Int., 2016, 94, 176–186 CrossRef CAS.
- F. Zhou, K. Adachi and K. Kato, Friction and wear behavior of BCN coatings sliding against ceramic and steel balls in various environments, Wear, 2006, 261, 301–310 CrossRef CAS.
- Y. M. Chen, Z. X. Zen, S. R. Yang and J. Y. Zhang, The tribological performance of BCN films under ionic liquids lubrication, Diamond Relat. Mater., 2009, 18(1), 20–26 CrossRef CAS.
- J. L. Xiao, C. B. Wang, Q. Shen and L. M. Zhang, Influence of nitrogen pressure on bonding structure and mechanical properties of pulsed laser deposited BCN thin films, Surf. Coat. Technol., 2015, 276, 141–144 CrossRef CAS.
- S. Nakao, T. Sonoda, K. Tsugawa, J. Choi and T. Kato, Effects of nitrogen gas ratio on composition and microstructure of BCN films prepared by RF magnetron sputtering, Vacuum, 2010, 84(5), 642–647 CrossRef.
- Y. Sakamaotoa, M. Nose, T. Mae, E. Honbo, M. Zhou and K. Nogi, Structure and properties of Cr–B, Cr–B–N and multilayer Cr–B/Cr–B–N thin films prepared by r.f.-sputtering, Surf. Coat. Technol., 2003, 174–175, 444–449 CrossRef.
- X. Y. Chen, Z. H. Wang, S. L. Ma and V. Ji, Microstructure, mechanical and tribological properties of Ti–B–C–N films prepared by reactive magnetron sputtering, Diamond Relat. Mater., 2010, 19(10), 1336–1340 CrossRef CAS.
- M. A. Mannan, H. Noguchi, T. Kida, M. Nagano, N. Hirao and Y. Baba, Growth and characterization of stoichiometric BCN films on highly oriented pyrolytic graphite by radiofrequency plasma enhanced chemical vapor deposition, Thin Solid Films, 2010, 518(15), 4163–4169 CrossRef.
- J. Lin, B. Mishra, J. J Moore, M. Pinkas and W. D. Sproul, Structure and properties of Ti–B–C–N nanocomposite coatings synthesized using pulsed closed field unbalanced magnetron sputtering (P-CFUBMS), Surf. Coat. Technol., 2008, 203(5–7), 588–593 CrossRef CAS.
- L. Chekour, C. Nouveau, A. Chala, C. Labidi, N. Rouag and M. A. Djouadi, Growth mechanism for chromium nitride films deposited by magnetron and triode sputtering methods, Surf. Coat. Technol., 2005, 200(1–4), 241–244 CrossRef CAS.
- A. M. A. El-Rahman and R. H. Wei, Effect of ion bombardment on structural, mechanical, erosion and corrosion properties of Ti–Si–C–N nanocomposite coatings, Surf. Coat. Technol., 2014, 258, 320–328 CrossRef.
- M. Uchidate, H. Liu, K. Yamamoto and A. Iwabuchi, Effects of hard water on tribological
properties of DLC rubbed against stainless steel and brass, Wear, 2013, 308(1–2), 79–85 CrossRef CAS.
- M. A. Tofighy and T. Mohammadi, Permanent hard water softening using carbon nanotube sheets, Desalination, 2011, 268(1–3), 208–213 CrossRef CAS.
- C. M. T. Sanchez, H. D. Fonseca-Filho, M. E. H. M. D. Costa and F. L. Freire, Nitrogen incorporation into titanium diboride films deposited by dc magnetron sputtering: structural modifications, Thin Solid Films, 2009, 517(19), 5683–5688 CrossRef CAS.
- J. L. He, S. Miyake, Y. Setsuhara, I. Shimizu, M. Suzuki, K. Numata and H. Saito, Improved anti-wear performance of nanostructured titanium boron nitride coatings, Wear, 2001, 249(5–6), 498–502 CrossRef CAS.
- D. C. Reigada, R. Prioli, L. G. Jacobsohn and F. L. Freire Jr, Boron carbide films deposited by a magnetron sputter-ion plating process: film composition and tribological properties, Diamond Relat. Mater., 2000, 9(3–6), 489–493 CrossRef CAS.
- A. Erdemir, C. Bindal and G. R. Fenske, Formation of ultralow friction surface films on boron carbide, Appl. Phys. Lett., 1996, 68(12), 1637–1639 CrossRef CAS.
- X. D. Ma and W. N. Unertl, The boron oxide–boric acid system: nanoscale mechanical and wear properties, J. Mater. Res., 1999, 14(8), 3455–3466 CrossRef CAS.
- Q. Ma, F. Zhou, S. Gao, Z. W. Wu, Q. Z. Wang, K. M. Chen, Z. F. Zhou and L. K. Y. Li, Influence of boron content on the microstructure and tribological properties of Cr–B–N coatings in water lubrication, Appl. Surf. Sci., 2016, 377, 394–405 CrossRef CAS.
- J. F. Archard, Contact and Rubbing of Flat Surfaces, J. Appl. Phys., 1953, 24(8), 981–988 CrossRef.
- Y. X. Wang, J. B. Pu, J. F. Wang, J. L. Li, J. M. Chen and Q. J. Xue, Interlayer design for the graphite-like carbon film with high load-bearing capacity under sliding-friction condition in water, Appl. Surf. Sci., 2014, 311, 816–824 CrossRef CAS.
|
This journal is © The Royal Society of Chemistry 2016 |