DOI:
10.1039/C6RA08888K
(Paper)
RSC Adv., 2016,
6, 69196-69205
Synthesis of carbazole-based copolymers containing carbazole-thiazolo[5,4-d]thiazole groups with different dopants and their fluorescence and electrical conductivity applications
Received
6th April 2016
, Accepted 4th July 2016
First published on 4th July 2016
Abstract
To improve the electrical conductivity of poly(carbazole-thiazolo[5,4-d]thiazole) (p-CzTT), five different dopants, such as HCl, Fe(III), Cu(II), H3BO3, and I2, were introduced into its framework through protonation of a nitrogen atom or by complex formation. Carbazole-thiazolo[5,4-d]thiazole (CzTT) and poly(carbazole-thiazolo[5,4-d]thiazole) (p-CzTT) were synthesized by the reactions of carbazole aldehydes and dithiooxamide. The synthesized monomer and polymer were confirmed by FT-IR, UV-vis, 1H, 13C-NMR, and GPC studies. The doped polymers were examined by FT-IR spectroscopy, UV-visible absorption, fluorescence, thermogravimetric analysis (TGA), cyclic voltammetry (CV), and electrical conductivity. The optical band gap (Eoptg) of CzTT and p-CzTT were found to be 2.74 eV and 1.95 eV, respectively. The thermal stability of the polymer showed 5% weight loss at 358 °C. Based on CV analysis, the HOMO and LUMO energy levels and the electrochemical band gap (Ecvg) of p-CzTT were obtained as −5.86 eV, −3.19 eV, and 2.67 eV, respectively. The CzTT and p-CzTT exhibited fluorescence emission at 461 nm and 448 nm, respectively. Among the dopants introduced, Fe(III)- and I2-doped polymer showed excellent electrical conductivities of 6.8 × 10−5 S cm−1 and 5.9 × 10−5 S cm−1, respectively.
1. Introduction
Conducting polymers play a vital role in the conversion of light energy into electrical energy (solar cell) and in the operation of energy storage devices (e.g., batteries, supercapacitors, and electrical double layer capacitors).1,2 Electric devices consist of organic materials that absorb sunlight and convert photons into electrons (e.g., solar cells) or liquid electrolyte reactions (e.g., power tools).3–5 In a particular series of organic electronic devices, conjugated polymers exhibit the presence of delocalized π-electrons as the carriers for an electron moving character. Polythiophene, polyacetylene, polyaniline, polypyrrole and polyphenylene polymers cover a portion of such conducting polymers.6–8
Over the past few years, π-conjugated fused ring systems have attracted the attention of many researchers due to their potential usage in the areas of optics, electronics, and solar energy technologies.9 The carbazole is a tricyclic fused ring system containing a single nitrogen atom, which increases the electron-rich nature and hole-transporting property of the system.10 Carbazole-based polymer and small molecules have been considered as an organic semiconductor. Carbazole derivatives have a deep-lying highest occupied molecular orbital (HOMO) energy level that is attributed to a high open-circuit voltage (Voc). Furthermore, they have high hole mobility due to the more preferential π–π stacking, which can enhance the short-circuit current density (Jsc) and fill factor (FF) of devices. Other than the conducting properties, carbazole-based polymers have fluorescence, electro-optical, and hole-transporting properties too.11–15 Nowadays careful design of donor–acceptor (D–A) type molecules is known to be crucial due to their effective photoinduced intramolecular charge-transfer characteristics between the donor and acceptor moieties. The thiazolo[5,4-d]thiazole (TTz) moiety is commonly employed as an acceptor in the system of donor–acceptor (D–A) type polymers owing to its good electron-withdrawing properties, planar structure, and easy synthesis.16 The TTz moiety has a rigid coplanar ring, which facilitates intermolecular ordering with high charge-carrier mobility.17–19 Therefore, the introduction of the thiazolo[5,4-d]thiazole moiety into electron-poor alternating copolymers may generate efficient photovoltaic materials with both a low band gap and high charge-carrier mobility.20,21 The structural rigidity of a TTz unit fused with the coplanar ring shows a low solubility in organic solvents. The solubility of the polymer can, however, be increased by introducing an alkyl side chain in the π-conjugated fused ring system. Hence, the polymers will have a fused coplanar as the main backbone with good planarity for intermolecular packing and high charge mobility.22,23 Olgun et al. reported a series of copolymer dyes involving the thiazolo[5,4-d]thiazolo acceptor moiety and different benzene, triphenylamines as the donor units.24–28 They described a TTz acceptor moiety-containing polymer, which showed a low band gap of 1.36 eV and 2.50 eV. The polymer and their doped polymers exhibited a maximum electrical conductivity of 0.35 μS cm−1. The phenylene- and thiazolo[5,4-d]thiazoles-containing compounds were investigated for fluorescence, electrical conductivity, and solar cell applications. Previous reports revealed that the molecules of poly(phenylene-thiazolo[5,4-d]thiazole),26 thiophene-thiazolo[5,4-d]thiazole,29 dithiophene-co-thiazolo[5,4-d]thiazole30 and poly[{4,4-bis(2-ethylhexyl)-4H-cyclopenta[2,1-b:3,4-b′]dithiophene-2,6-diyl}-alt-{2,5-di(thiophen-2-yl)thiazolo-[5,4-d]thiazole-5,5′-diyl}],31 have been used for solar cells and electrical applications.
In this work, carbazole-thiazolo[5,4-d]thiazole (CzTT) and poly(carbazole-thiazolo[5,4-d]thiazole) (p-CzTT) were synthesized by reacting 9-hexyl-9H-carbazole-3-carbaldehyde (or) 9-hexyl-9H-carbazole-3,6-dicarbaldehyde with dithiooxamide. The synthesized p-CzTT was doped with HCl, I2, Cu(II), Fe(III) and H3BO3. The monomer (CzTT) and polymer (p-CzTT) were confirmed by 1H, 13C-NMR and GPC. The thermal stability of the p-CzTT was examined by TGA. The doped and undoped forms of p-CzTT polymer were characterized by UV-vis, FT-IR and electrical conductivity. The HOMO and LUMO energy levels and the band gap of CzTT and p-CzTT were calculated by cyclic voltammetry (CV).
2. Results and discussion
2.1. Synthesis and doping of p-CzTT polymer
Carbazole-thiazolo[5,4-d]thiazole and poly(carbazole-thiazolo[5,4-d]thiazole) (p-CzTT) were synthesized from 9-hexyl-9H-carbazole-3-carbaldehyde, 9-hexyl-9H-carbazole-3,6-dicarbaldehyde and dithiooxamide. An outline of the synthetic procedure adopted is shown in Scheme 1. The carbazole was alkylated by using hexyl bromide. The purpose of introducing a hexyl chain in the carbazole was to reduce the steric hindrance and increase solubility. Further, the carbazole was formylated in the 3,6-position by Vilsmeier–Haack formylation reaction. The obtained single and double side aldehyde product was treated with dithiooxamide to obtain the monomer (CzTT) and polymer (p-CzTT). The electron-rich carbazole moiety acted as a p-type material while the electron-deficient thiazolo[5,4-d]thiazole acted as an n-type material. This alternating copolymer has a greater push–pull property due to the D–A type polymer structure. The p-CzTT polymer was doped with different dopants, such as HCl, Fe(III), Cu(II), H3BO3, and I2. The possible interaction of the dopants with the polymer is either partial oxidation or reduction. These effects may enhance the electrical conductivity and binding with metals to form a complex. This was studied by using cyclic voltammetry and fluorescence.
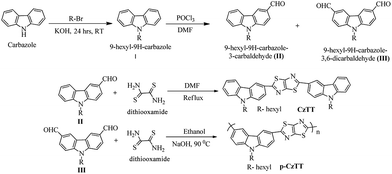 |
| Scheme 1 Synthesis of CzTT and p-CzTT. | |
The UV-visible absorption spectrum of the monomer (CzTT) exhibited three absorption bands at λmax of 284, 326 and 403 nm. Whereas, the polymer (p-CzTT) followed the same pattern at λmax 282, 338 and 557 nm. The polymer (p-CzTT) is stable up to 356 °C, as interpreted from TGA curve. The p-CzTT shows a fluorescence emission peak at 448 nm with the corresponding excitation at 338 nm. The undoped and doped polymers were examined for the HOMO and LUMO energy level and their band gap using CV.
In the present work, the polymer p-CzTT was doped with different dopants, such as HCl, Fe(III), Cu(II), H3BO3 and I2. All the dopants effectively reacted with the TTz moiety to form either complex or N-substituted bonds without any effects on the carbazole moiety. Dopants such as strong and weak acids can protonate nitrogen atoms of the TTz moiety by forming N–H bond or nitrogen–boron bonds. Fe(III) and Cu(II) ions showed a redox doping behaviour by favouring a complex formation with the TTz moiety. Iodine is also one of the redox dopants that may form a complex with N and S atoms. The effect of the dopants and their spectroscopic results are discussed.
2.2. GPC molecular weight and thermal stability
The molecular weight of the synthesized polymer was investigated by GPC measurements in THF solution. From the GPC, we obtained a weight average molecular weight (Mw) of 2498 g mol−1 and a number average molecular weight (Mn) of 2485 g mol−1 with n = 8 and 9. The thermal property of the polymer (p-CzTT) was investigated by thermogravimetric analysis (TGA) under nitrogen atmosphere at a heating rate of 10 °C min−1, as shown in Fig. 1. The TGA curve illustrates that the 5% decomposition temperature (Td) of the polymer was found to be 358 °C. The polymer showed high thermal stability, which makes it an attractive candidate for electrical and solar cell applications.34
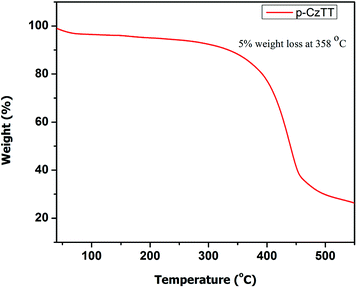 |
| Fig. 1 TGA curve of the p-CzTT polymer. | |
2.3. 1H-NMR spectroscopy
The 1H-NMR spectrum of the copolymer (p-CzTT) was recorded in DMSO-d6, and the obtained spectrum is given in Fig. 2. The p-CzTT copolymer containing an aldehyde proton (a) appeared at 10.13 ppm. The hexyl protons (c, d, e) appeared in the range of 0.5–5 ppm, whereas the carbazole protons (b, *) appeared as multiplets in the range of 6.5–9.5 ppm. These results confirm the chemical structure of the p-CzTT copolymer.
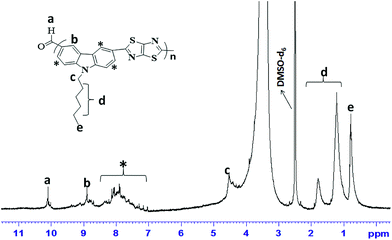 |
| Fig. 2 1H-NMR spectrum of p-CzTT. | |
2.4. FT-IR spectra of undoped and doped polymers
Synthesized and doped polymers were studied by FT-IR spectroscopy. FT-IR spectra recorded by making a solid mixture of the polymer with dopants and the obtained spectra are shown in Fig. 3. The obtained results are summarized in Table 1. From the FT-IR spectra, the effects of various dopants were compared and these confirmed the coordination of the dopants with the polymer. The FT-IR spectra of the polymer showed the C
N vibration peak at 1673 cm−1, which gets shifted to a low vibration frequency of 1593 cm−1 after HCl addition. This might be due to protonation of the nitrogen atoms. The peak at 1192 cm−1 indicates B–O vibration of the H3BO3 doped sample. The shift of the peak at 1321 cm−1 to 1379 cm−1 could be possibly due to bond formation between B–N after doping with H3BO3.25 The characteristic polymer peak at 1321 cm−1 is completely varied after adding dopant, which indicates the formation of a new bond between the dopant and polymer.35
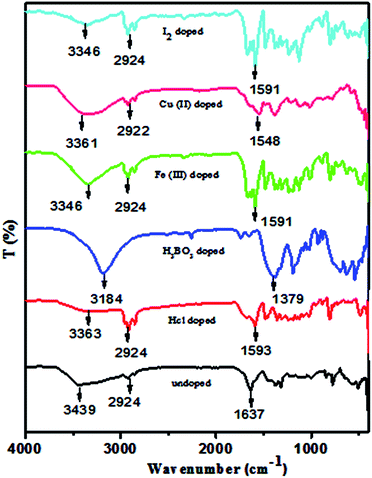 |
| Fig. 3 FT-IR spectra of the undoped and doped forms of the polymer samples. | |
Table 1 FT-IR spectra data of the undoped and doped polymers
Samples |
FT-IR absorption peak (in cm−1) |
p-CzTT |
773 |
— |
1321 |
1637 |
2924 |
3439 |
Cu(II) doped |
— |
— |
1381 |
1548 |
2922 |
3361 |
Fe(III) doped |
804 |
1236 |
1483 |
1591 |
2924 |
3346 |
I2 doped |
804 |
1236 |
1483 |
1591 |
2924 |
3346 |
HCl doped |
806 |
1259 |
1483 |
1593 |
2918 |
3363 |
H3BO3 doped |
791 |
1192 |
1379 |
1643 |
2970 |
3184 |
2.5. UV-visible absorption spectroscopy
The UV-vis absorption spectra of the synthesized monomer (CzTT) and polymer (p-CzTT) were examined in DMF solutions (1.5 × 10−5 M), and the absorption results are shown in Fig. 4. CzTT and p-CzTT showed three maximum absorption bands at (284, 282 nm), (326, 338 nm) and (403, 557 nm), respectively. The bands at 326 nm and 338 nm for the monomer and polymer indicate the electron transition of π–π* for the carbazole moiety. The 403 nm and 557 nm bands correspond to the thiazolo[5,4-d]thiazole (S and N) group and the n–σ* electron transition for CzTT and p-CzTT, respectively. Fig. 5 illustrates the UV spectra of the undoped and HCl-doped copolymers in various concentrations. The polymer showed the last band at two shoulder peak λmax wavelengths at 524 nm and 557 nm. After HCl doping, the shoulder peak completely shifted from 557 nm to 567 nm. This is due to the interaction of the HCl proton with the polymer nitrogen, leading to the protonation of N. The UV-vis absorbance spectra of Cu(II)-, Fe(III)- and I2-doped p-CzTT polymer recorded in DMF solvent (0.20 g L−1) are shown in Fig. 6. The concentration of dopants were 2 N (HCl), 1.81 × 10−4 M (Cu(II)), 1.6 × 10−4 M (Fe(III)), 6.18 × 10−4 M (H3BO3) and 1.2 × 10−4 M (I2) in DMF solutions. The electron-withdrawing nature of the dopants causes the shift in absorption peaks from a lower to higher wavelength. Moreover, it was observed that the I2-doped polymer showed more absorbance compared to the other dopants. The monomer and polymer optical band gaps were calculated from the UV absorption edge slope and found to be 451 and 635 nm, respectively. From these results, the optical band gap for the monomer and polymer was found to be 2.74 and 1.95 eV, respectively. The doped form of the polymer with HCl, Cu(II), Fe(III), H3BO3 and I2 showed optical band gaps (Eoptg) of 1.92, 3.17, 2.89, 1.92 and 2.63 eV, respectively. The above-mentioned values were calculated from the UV absorption edge of 645, 391, 429, 640 and 471 nm, respectively, corresponding to the doped form of the polymer with HCl, Cu(II), Fe(III) and I2.
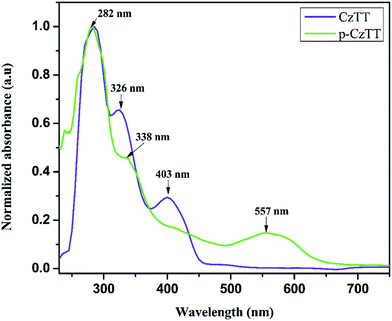 |
| Fig. 4 UV-visible spectra of the monomer and polymer in DMF solution. | |
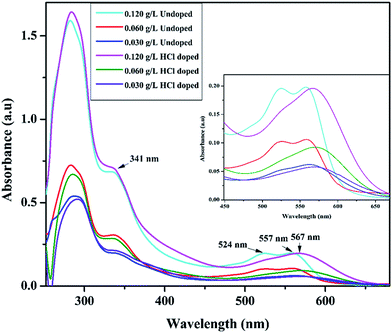 |
| Fig. 5 UV-visible spectra of the polymer and HCl-doped polymer at various concentrations in DMF solution. | |
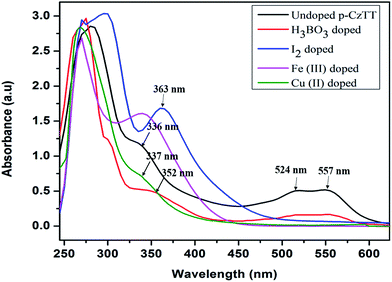 |
| Fig. 6 UV-visible spectra of undoped and Fe(III)-, Cu(II)- and I2-doped polymers in DMF solution. | |
2.6. Fluorescence spectroscopy
The synthesized monomer and polymer were characterized by fluorescence (FL) spectroscopy in DMF solvent. The polymer showed an emission peak at 448 nm and an excitation peak at 338 nm. The polymer doped with HCl, Cu(II), Fe(III), H3BO3 and I2 showed an excitation peak at 341, 341, 340, 342 nm and 363 nm and an emission peak at 443, 440, 443, 441 nm and 447 nm, respectively. Further, the FL quantum yields (ΦF) were measured by using quinine sulphate as the reference standard.32 The FL quantum yields were found to be 0.29 and 0.30 for CzTT and p-CzTT, respectively, in DMF solutions. The HCl-, Cu(II)-, Fe(III)-, H3BO3- and I2-doped polymer showed a ΦF of 0.25, 0.33, 0.36, 0.33 and 0.34, respectively. The Fe(III)- and I2-doped polymers showed higher ΦF values due to the greater electron-withdrawing nature of the dopant. The undoped polymer was quenched with the addition of 0.1 mL HCl increments and the fluorescence was recorded until it was completely quenched with an equal molar ratio of polymer and HCl. The fluorescence study was done by the 0.1 mL addition of each dopant into the polymer. The FL intensity was observed to continuously decrease with the addition of dopant up to an equimolar addition. All the emission peaks were almost similar to each other. These results conclude that the dopant–polymer interactions were well identified. The quenching FL intensity and red-shifted emission suggest that the polymer molecules have stronger π–π interaction with the dopants. The fluorescence results of the doped form of the polymers reveal the intermolecular interaction and enhance the charge carrier transport properties (Fig. 7).
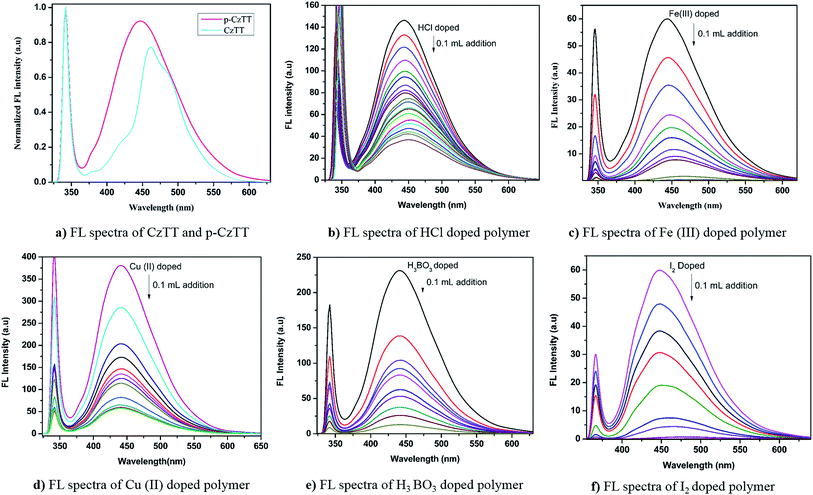 |
| Fig. 7 Fluorescence spectra of the undoped and doped forms of the polymers. | |
2.7. Electrochemical properties
The electrochemical behaviour of the undoped and doped polymer samples was studied by cyclic voltammetry in DMSO solutions with 0.1 M [nBu4N]+[PF6] electrolyte solution. The obtained results are shown in Fig. 8, and the data are summarized in Table 2. The onset oxidation potential and reduction onset potential of the undoped polymer were observed at 1.38 eV and −1.29 eV. The HOMO and LUMO energy levels were calculated by using eqn (1) and (2). The band gap was calculated from the energy difference between the HOMO and LUMO energy levels. |
HOMO = −eV (Eonsetox − E1/2ferrocene + 4.8)
| (1) |
|
LUMO = −eV (Eonsetred − E1/2ferrocene + 4.8)
| (2) |
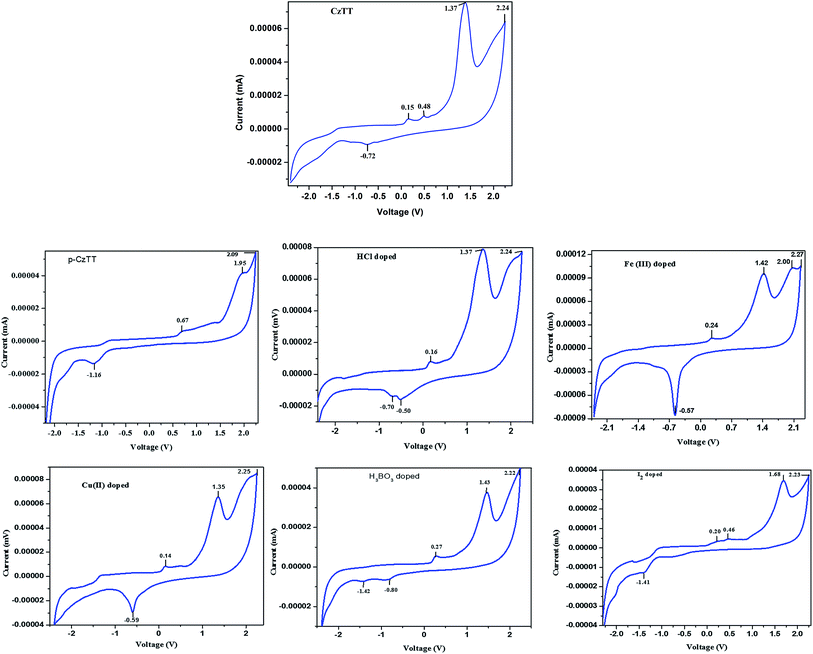 |
| Fig. 8 Cyclic voltammograms of the undoped and doped forms of the polymer samples. Scan rate = 100 mV s−1. | |
Table 2 CV data of the undoped and doped polymers
Samples |
Onset oxidation potential (V) |
Onset reduction potential (V) |
HOMO (eV) |
LUMO (eV) |
Band gap (Eg) (eV) |
CzTT |
0.65 |
−1.39 |
−5.11 |
−3.07 |
2.04 |
p-CzTT |
1.38 |
−1.29 |
−5.86 |
−3.19 |
2.67 |
HCl doped |
0.50 |
−2.02 |
−4.98 |
−2.46 |
2.52 |
H3BO3 doped |
0.95 |
−1.91 |
−5.43 |
−3.17 |
2.26 |
Cu(II) doped |
0.59 |
−1.40 |
−5.07 |
−3.08 |
1.99 |
Fe(III) doped |
0.54 |
−1.60 |
−5.02 |
−2.88 |
2.14 |
I2 doped |
0.82 |
−0.95 |
−5.30 |
−3.53 |
1.77 |
In these cases, the doped polymers have enormously changed oxidation and reduction potential due to their oxidation and reduction behaviours. The HOMO and LUMO and the electrochemical band gap of doped and undoped polymers are also given in the energy level diagram in Fig. 9. Metal-doped polymer shows a low band gap compared to acid-doped polymer. Fe(III)-doped polymer shows a strong reduction potential range at −1.60 eV. The H3BO3- and I2-doped polymer show deep-lying HOMO energy levels in the energy band diagram. CV studies concluded that the doped form of the polymer could be used in organic photovoltaic applications. The doped forms of the polymer showed the lowest HOMO and LUMO energy levels compared to the undoped polymer. The polymer also showed a deep-lying HOMO energy level at −5.86 eV, which could enhance the open-circuit voltage of solar cells, whereas the hole mobility and band gap are still low. The doped form of the polymer showed a low band gap and the lowest HOMO and LUMO energy levels. The H3BO3- and I2-doped polymer both showed a deep-lying HOMO energy level at −5.43 and −5.30 eV, respectively, while the Fe(III)- and HCl-doped polymers showed low-lying LUMO energy levels of −2.88 and 2.46 eV, respectively. The Fe(III)- and Cu(II)-doped polymers show very close HOMO and LUMO energy levels. Wei et al. reported that the ideal polymer showed HOMO and LUMO energy levels and a band gap at −5.4 eV, −3.9 eV and 1.5 eV, respectively.36 According to the ideal values, our doped form of the polymer sample also showed HOMO and LUMO energy levels close to the reported results, and, due to the above reason, all these doped forms of the polymer could be used as a donor in bulk heterojunction organic solar cell (BHJ-OSC) applications.
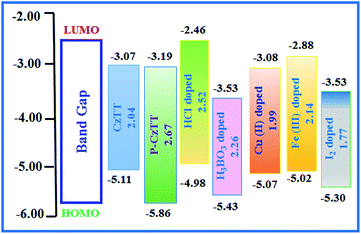 |
| Fig. 9 HOMO and LUMO energy level diagram of the undoped and doped polymers. | |
2.8. SEM microscopy analysis
The surface morphology of undoped and Fe(III)-doped polymer were investigated by scanning electron microscopy (SEM) analysis and the images are shown in Fig. 10. The surface of the undoped p-CzTT sample appears to have a gel-like morphology. A porous gel network surface covered with tumours was observed for the Fe(III)-doped polymer. The appearance of tumours might be due to the incorporation of Fe(III) in the polymer. Hence, these results indicate that the formation of pores might facilitate the electron movement. The incorporation of dopants in the polymer promotes an easy transportation of electrons, leading to the enhanced electric conductivity of the doped polymer.
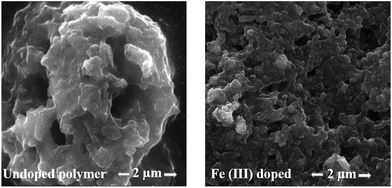 |
| Fig. 10 SEM images of the undoped and Fe(III)-doped polymer. | |
2.9. Powder XRD of the undoped and doped polymer
The undoped and HCl- and Fe(III)-doped polymer samples were studied by powder XRD. The XRD patterns (Fig. 11a) show the amorphous nature of the undoped polymer used as a precursor. Fig. 11b reveals the presence of multiple elements, such as S, HCl and H. The peaks corresponding to these elements were confirmed by matching with the standard JCPDS data cards (S: 96-901-2335, 2783; HCl: 96-101-0389 and H: 96-901-3086, 3088), respectively. Thus, this reveals that HCl was successfully doped with the polymer. Similarly, dual peaks were noticed in Fig. 11c. These peaks were found to match with the standard JCPDS patterns of Fe (96-900-0667) and S (96-901-2363). These observations prove the presence of Fe(III) in the polymer matrix. The XRD patterns also reveal the amorphous nature of the polymer samples, which could be useful for electrical conductivity applications. The HCl-doped polymer samples show a more crystalline pattern as compared to Fe(III)-doped polymer samples. Due to this crystalline nature, the HCl-doped polymer shows a low electrical conductivity.
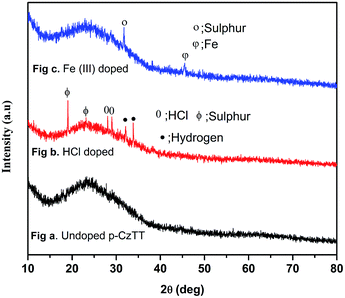 |
| Fig. 11 XRD patterns of the undoped and HCl and Fe(III)-doped polymers. | |
3. Electrical conductivity
The electrical conductivities of the undoped and doped forms of the polymers were measured using the impedance spectroscopy method, and the obtained results are shown in Fig. 12. The conductivity was calculated by using the equation σ = (L/RbA), where Rb is the bulk resistance, L is the thickness and A is the area of the sample. The conductivity was done at three different temperatures, such as 303, 323, 373 K, and the obtained data are summarized in Table 3. The conductivity of the polymer was found to increase with temperature because of the enhancement in the free volume theory and segmental motion of the polymer chain, which leads to improving the electrical conductivity of the polymer.33 It is shown in Table 3 that the conductivity values of the doped polymer are higher than the undoped polymer with an increase in temperature.26 This may be due to increasing the overall mobility of the electrons and the free volume of the polymer chains. Since I2 and Fe(III) are more electron-withdrawing, these dopants may enhance the electrical conductivity. The incorporation of Fe(III) and I2 dopants can improve the electrical properties in different ways; for example, the porous surface will dissociate the moving of more electrons.34 The HCl-doped polymer showed a more crystalline nature compared to the Fe(III)-doped polymer, as confirmed by the XRD results. The amorphous nature of the polymer showed its high conductivity as it initiates the breakdown of transient coordination bonds between charge carriers.35 Due to this, a higher electrical conductivity was observed in the doped form of the polymers. At 303 K, we observed that the electrical conductivity increases from undoped to Fe(III)-doped polymers from one-fold to nine-fold. Similarly, at the highest temperature of 373 K, the conductivity increases from one-fold to seven-fold. The percentage of the conductivity also increased with respect to the dopant, which is shown Table 3. In particular, Fe(III)-doped polymer showed the maximum electrical conductivity of 6.8 × 10−6 S cm−1 at 373 K.
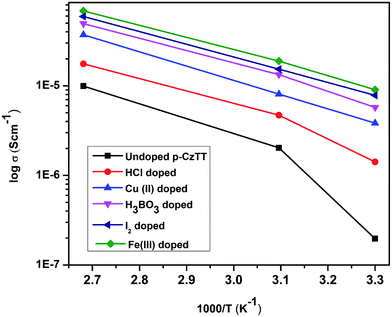 |
| Fig. 12 Electrical conductivity of the undoped and doped polymers. | |
Table 3 Conductivity values of the undoped and doped polymers
Samples |
Conductivity (S cm−1) |
303 K |
% |
323 K |
% |
373 K |
% |
p-CzTT |
1.30 × 10−7 |
— |
1.93 × 10−6 |
— |
9.89 × 10−6 |
— |
HCl doped |
1.65 × 10−6 |
1169 |
4.68 × 10−6 |
143 |
1.76 × 10−5 |
77 |
Cu(II) doped |
3.82 × 10−6 |
2838 |
8.00 × 10−6 |
314 |
3.69 × 10−5 |
273 |
H3BO3 doped |
5.82 × 10−6 |
4376 |
1.34 × 10−5 |
594 |
4.92 × 10−5 |
397 |
I2 doped |
7.81 × 10−6 |
5907 |
1.54 × 10−5 |
697 |
5.93 × 10−5 |
499 |
Fe(III) doped |
9.04 × 10−6 |
6853 |
1.90 × 10−5 |
884 |
6.85 × 10−5 |
592 |
4. Conclusions
In conclusion, we observed that the addition of a dopant to the copolymer can lead to an observable decrease in the band gap (Ecvg) and an increase in the electrical conductivity of p-CzTT. The use of HCl, Cu(II), Fe(III), H3BO3 and I2 dopants changed their polymer HOMO and LUMO energy levels, optical and electrochemical properties. Also, the polymer exhibited a strong FL emission peak at 448 nm and excitation peak at 338 nm. The Cu(II)- and I2-doped polymers showed very low bang gaps of 1.99 and 1.77 eV, respectively. The maximum electrical conductivity was shown by the Fe(III) polymer and was found to be 6.8 × 10−5 S cm−1. The undoped and doped polymers showed more thermal stability, a deep-lying HOMO energy level, and broad absorption range. This result concluded that doped p-CzTT is a good donor material for BHJ-OSC applications.
5. Experimental
5.1. Materials
Carbazole, 1-bromohexane and dithiooxamide were purchased from Sigma Aldrich Company. Phosphoryl chloride (POCl3), dry dimethylformamide (DMF), dichloromethane (DCM) and chloroform (CHCl3) were obtained from SD Fine-Chem Limited. Dimethyl sulfoxide (DMSO), methanol, HCl solution (37%), Cu(CH3COO)2·H2O, FeCl3·6H2O, H3BO3, I2, potassium tert-butoxide (t-BuOK), potassium hydroxide (KOH) and sodium hydroxide (NaOH) were purchased from AVRA Company. Other solvents were purchased commercially as AR-grade quality and used without further purification. Column chromatography was performed using silica gel Merck (60–120 mesh).
5.2. Methods
The IR spectra were recorded using a SHIMADZU Infrared spectrophotometer (400–4000 cm−1) with IV resolution. The FT-IR spectra were measured by using a KBr pellet. 1H and 13C-NMR spectra were obtained with a Bruker 400 MHz spectrometer in CDCl3 and DMSO-d6 solvent using tetramethylsilane as an internal standard. The UV-visible absorption spectra were recorded with a JASCO V-670 spectrophotometer at room temperature. The fluorescence spectra were recorded using a Hitachi F-7000FL spectrometer. Thermal analyses were carried out using a SDT Q600 V8.3 Build 101 thermogravimetric analyzer (TGA). Cyclic voltammograms were recorded with AutoLab 302 N at a scan rate of 100 mV s−1 in a 0.1 M solution of tetrabutylammonium tetrafluoroborate in DMSO solution. Two platinum electrodes were used as counter and reference electrode and a glassy carbon electrode as the working electrode. The powder XRD analyses were recorded using the PANalytical device (PW3040/60) at room temperature. The morphology study was carried out using a Hitachi (S-3000H) scanning electron microscope (SEM). The electrical conductivity of the polymer was studied by impedance analysis using a 3250 LCR HITESTER meter in the frequency range of 50 Hz to 5 MHz at different temperatures (303–373 K).
5.2.1. Synthesis of 9-hexyl-9-H-carbazole (I). Carbazole (3.30 g, 20 mmol) and potassium hydroxide (2.96 g, 52.8 mmol) were dissolved in DMF (40 mL) and then 1-bromohexane (3.30 g, 20 mmol) was added drop wise. The reaction mixture was stirred at room temperature for 48 h. The reaction mixture was poured into water and extracted with chloroform and then dried over anhydrous magnesium sulphate. The solvent was removed by rotary evaporator, and the residue was recrystallized in n-hexane to afford compound 1a (4.8 g, 92%) as a white solid. 1H NMR (400 MHz, CDCl3, ppm): 8.11–8.09 (d, 2H, J = 2.4 Hz), 7.46–7.42 (m, 2H), 7.42–7.39 (d, 2H, J = 8.4 Hz), 7.26–7.20 (m, 2H), 4.31–4.28 (t, 2H, J = 7.2 Hz), 1.88–1.85 (m, 2H), 1.41–1.37 (m, 6H), 0.86 (t, 3H).
5.2.2. Synthesis of 9-hexyl-9H-carbazole-3-carbaldehyde (II). POCl3 (25 mL, 0.27 mol) was added drop wise to DMF (35 mL, 0.45 mol) for 30 min at 0 °C. Compound 1a (4.00 g, 16 mmol) in dichloromethane (20 mL) was added to the above solution at room temperature, after which the temperature was increased to 90 °C and the mixture was stirred for 4 h. The reaction mixture was then poured into ice cubes and neutralized with NaOH solution. The solution was extracted three times with ethyl acetate and dried over anhydrous magnesium sulphate. The excess of dichloromethane was removed by vacuum distillation. The crude product was purified by column chromatography (eluent: hexane/ethyl acetate – 9
:
1) giving the product as a white solid compound (9-hexyl-9H-carbazole-3-carbaldehyde (II), yield: 68%).1H NMR (CDCl3, ppm): 10.07 (s, 1H), 8.57 (s, 1H), 8.14–8.12 (d, 1H, J = 8 Hz), 8.00–7.98 (dd, 1H, J = 6.8 Hz), 7.55–7.51 (t, 1H, J = 8 Hz), 7.44–7.42 (d, 2H, J = 8.4 Hz), 7.33–7.30 (t, 1H, J = 7.2 Hz), 4.30–4.26 (t, 2H, J = 7.6 Hz), 1.90–1.82 (m, 2H), 1.31–1.28 (m, 6H), 0.87 (m, 3H).
5.2.3. Synthesis of 9-hexyl-9H-carbazole-3,6-dicarbaldehyde (III). POCl3 (25 mL, 0.27 mol) was added drop wise to DMF (35 mL, 0.45 mol) for 1 h at 0 °C. Compound 1a (4.00 g, 16 mmol) in dichloromethane (20 mL) was added to the above solution at room temperature, after which, the temperature was increased to 90 °C and the mixture was stirred for 24 h. The same work-up procedure was followed according to the synthesis of compound II. The crude product was purified by column chromatography (eluent: hexane/ethyl acetate – 8
:
2) using silica gel (2.9 g, yield: 59%).1H NMR (CDCl3, ppm): 10.13 (s, 2H), 8.67 (s, 2H), 8.09–8.07 (d, 2H, J = 8.4 Hz), 7.56–7.54 (d, 2H, J = 8.8 Hz), 4.40–4.36 (t, 2H, J = 7.2 Hz), 1.93–1.89 (m, 2H), 1.38–1.34 (m, 6H), 0.86 (m, 3H).
5.2.4. Synthesis of carbazole-thiazolo[5,4-d]thiazole (CzTT). Compound II (0.620 g, 2.2 mmol) and dithiooxamide (0.120 g, 1 mmol) in DMF solution were heated and refluxed for 5 h under a N2 atmosphere. After the reaction mixture was cooled to room temperature, it was poured into water and extracted with dichloromethane. The organic layer was washed with water and dried over anhydrous magnesium sulphate. The crude mixture was purified by column chromatography (eluent: hexane/ethyl acetate – 8
:
2) giving the product as a yellow solid (yield: 32%). 1H-NMR (CDCl3, ppm): 8.75 (s, 2H), 8.21–8.19 (d, 2H, J = 7.6 Hz), 8.13–8.10 (dd, 2H, J = 6.8 Hz), 7.53–7.50 (t, 2H, J = 7.2 Hz), 7.48–7.54 (dd-4H, J = 4 Hz), 7.24–7.22 (t, 2H, J = 2.4 Hz) 4.35–4.32 (t, 4H, J = 7.2 Hz), 1.92–1.89 (m, 4H), 1.32–1.25 (m, 12H), 0.89 (m, 6H). 13C-NMR (CDCl3, ppm): 142.06, 141.34, 126.63, 125.59, 124.60, 123.61, 121.07, 119.90, 118.97, 109.37, 43.60, 31.80, 29.94, 27.21, 22.78, and 14.24.
5.2.5. Synthesis of poly(carbazole-thiazolo[5,4-d]thiazole) (p-CzTT). The compound III (0.61 g, 2 mmol) and dithiooxamide (0.20 g, 2 mmol) were mixed with ethanol and stirred at room temperature under a N2 atmosphere. After that, 1 M sodium hydroxide solution was added to the reaction mixture. Then, the temperature was increased to 90 °C and the mixture was stirred overnight, after which the reaction mixture was cooled to room temperature and poured into methanol. The obtained precipitate was filtered, washed with cold methanol and dried in an oven overnight. The compound was further purified by column chromatography (eluent: chloroform) using silica gel and precipitated with ethanol to give the product as a brown solid (yield: 68%). The compound p-CzTT was confirmed by 1H-NMR spectroscopy, as shown in Fig. 2.
5.2.6. p-CzTT polymer doped with different dopants. The p-CzTT polymer was doped with HCl, H3BO3, Cu(II), Fe(III) and I2 using Cu(CH3COO)2·H2O, FeCl3·6H2O, H3BO3 and I2 chemical agents. The polymer and doped polymers were recorded by using FT-IR, UV-vis absorption, cyclic voltammetry (CV) and electrical conductivity. The UV-visible spectrometry was performed with the polymer in DMF solution. For the cyclic voltammetry measurements, FT-IR and electrical conductivity measurements, the doping processes were carried out by mixing and grinding in the solid phase. 15 mg of solid dopants of Cu(CH3COO)2·H2O, FeCl3·6H2O, H3BO3, and I2 were added into the each 50 mg of the p-CzTT copolymer.
Acknowledgements
This work was supported by VIT University by providing laboratory facilities and fellowship to Mr G. S. The authors thank the DST for the financial support to this work through SERB research grant (SERB-SB/FT/CS-185/2011) and Solar Energy Research Initiative (SERI) Programme (DST/TM/SERI/FR/172(G)).
References
- V. Malytskyi, J. Simon, L. Patrone and J. Raimundo, RSC Adv., 2015, 5, 354 RSC
. - R. S. Kularatne, H. D. Magurudeniya, P. Sista, M. C. Biewer and M. C. Stefan, J. Polym. Sci., Part A: Polym. Chem., 2013, 51, 743 CrossRef CAS
. - J. Delgado, P. Bouit, S. Filippone, M. A. Herranz and N. Martín, Chem. Commun., 2010, 46, 4853 RSC
. - G. Zhang, Y. Fu, Q. Zhang and Z. Xie, Chem. Commun., 2010, 46, 4997 RSC
. - Y. Lin, Y. Li and X. Zhan, Chem. Soc. Rev., 2012, 41, 4245 RSC
. - J. Heinze, B. F. Uribe and S. Ludwigs, Chem. Rev., 2010, 110, 4724 CrossRef CAS PubMed
. - A. F. Diaz, K. K. Kanazawa and G. P. Gardini, J. Chem. Soc., Chem. Commun., 1979, 635 RSC
. - G. Tourillon and F. Garnier, J. Electroanal. Chem. Interfacial Electrochem., 1982, 35, 173 CrossRef
. - G. Sathiyan, E. K. T. Sivakumar, R. Ganesamoorthy, R. Thangamuthu and P. Sakthivel, Tetrahedron Lett., 2016, 57, 243 CrossRef CAS
. - P. Sakthivel, H. S. Song, N. Chakravarthi, J. W. Lee, Y. S. Gal, S. Hwang and S. H. Jin, Polymer, 2013, 54, 4883 CrossRef CAS
. - S. Ramkumar, S. Manoharan and S. Anandan, Dyes Pigm., 2012, 94, 503 CrossRef CAS
. - M. Irfan, K. D. Belfield and A. Saeed, RSC Adv., 2015, 5, 48760 RSC
. - W. Liu, S. Ying, Q. Sun, X. Qiu, H. Zhang, S. Xue and W. Yang, Dyes Pigm., 2016, 125, 8 CrossRef CAS
. - N. Nagarajan, G. Velmurugan, G. Prabhu, P. Venuvanalingam and R. Renganathan, J. Lumin., 2014, 147, 111 CrossRef CAS
. - D. Patra, D. Sahu, H. Padhy, D. Kekuda, C. W. Chu and H. C. Lin, J. Polym. Sci., Part A: Polym. Chem., 2010, 48, 5479 CrossRef CAS
. - Y. Li, Acc. Chem. Res., 2012, 45, 723 CrossRef CAS PubMed
. - P. Dutta, W. Yang, S. H. Eom and S. H. Lee, Org. Electron., 2012, 13, 273 CrossRef CAS
. - H. Z. Akpinar, Y. A. Udum and L. Toppare, J. Polym. Sci., Part A: Polym. Chem., 2013, 51, 3901 CrossRef CAS
. - W. Zhang, Q. Feng, Z. S. Wang and G. Zhou, Chem.–Asian J., 2013, 8, 939 CrossRef CAS PubMed
. - S. Subramaniyan, H. Xin, F. S. Kim, N. M. Murari, B. A. E. Courtright and S. A. Jenekhe, Macromolecules, 2014, 47, 4199 CrossRef CAS
. - Q. Shi, H. Fan, Y. Liu, J. Chen, Z. Shuai, W. Hu, Y. Li and X. Zhan, J. Polym. Sci., Part A: Polym. Chem., 2011, 49, 4875 CrossRef CAS
. - S. K. Lee, J. M. Cho, Y. Goo, W. S. Shin, J. C. Lee, W. H. Lee, N. Kang, H. K. Shim and S. J. Moon, Chem. Commun., 2011, 47, 1791 RSC
. - P. Sakthivel, K. Kranthiraja, C. Saravanan, K. Gunasekar, H. Kim, W. Shin, J. Jeong, H. Woo and S. H. Jin, J. Mater. Chem., 2014, 2, 6916 RSC
. - U. Olgun and M. Gulfen, React. Funct. Polym., 2014, 77, 23 CrossRef CAS
. - U. Olgun and D. M. Kalyon, Polymer, 2005, 46, 9423 CrossRef CAS
. - U. Olgun and M. Gulfen, RSC Adv., 2014, 4, 25165 RSC
. - U. Olgun and M. Gulfen, Dyes Pigm., 2014, 102, 189 CrossRef CAS
. - U. Olgun and M. Gulfen, Dyes Pigm., 2013, 99, 1004 CrossRef CAS
. - I. Osaka, R. Zhang, G. Sauve, D. M. Smilgies, T. Kowalewski and R. D. Mc Cullough, J. Am. Chem. Soc., 2009, 131, 2521 CrossRef CAS PubMed
. - V. Mierloo, S. Hadipour, A. Spijkman, M. J. Vanden brande, N. Ruttens and B. Kesters, Chem. Mater., 2012, 24, 587 CrossRef
. - S. P. Mishra, A. K. Palai, A. Kumar, R. Srivastava, M. N. Kamalasanan and M. Patri, Macromol. Chem. Phys., 2010, 211, 1890 CrossRef CAS
. - B. Umamahesh, M. Saravanakumar, T. Manojkumar and K. I. Sathiyanarayanan, RSC Adv., 2016, 6, 58549 RSC
. - M. D. Zipper, G. P. Simon, P. Cherry and A. J. Hill, J. Polym. Sci., Part B: Polym. Phys., 1994, 32, 1237 CrossRef CAS
. - V. Tamilavan, M. Song, S. H. Jin, H. J. Park, U. C. Yoon and M. H. Hyun, Synth. Met., 2012, 162, 1184 CrossRef CAS
. - S. Rajendran, R. S. Babu and P. Sivakumar, J. Power Sources, 2007, 170, 460 CrossRef CAS
. - H. Zhou, L. Yang and W. You, Macromolecules, 2012, 45, 607 CrossRef CAS
.
|
This journal is © The Royal Society of Chemistry 2016 |