DOI:
10.1039/C6RA08606C
(Paper)
RSC Adv., 2016,
6, 52131-52136
Conformal and non-conformal surface modification of honeycomb-patterned porous films via tunable Cassie–Wenzel transition†
Received
4th April 2016
, Accepted 20th May 2016
First published on 20th May 2016
Abstract
We describe here a facile and robust approach to conformal and non-conformal surface modification by tuning the wetting transition between the Wenzel state and the Cassie state. Highly ordered honeycomb-patterned porous films were prepared from a diblock copolymer by the breath figure method, and pincushion-like surfaces were obtained by removing the top surface layer of honeycomb films. It is found that the surface modification is highly dependent on the pre-wetting process. After complete pre-wetting, polydopamine (PDA) and polyetherimide (PEI) deposit both inside and outside of the pores, and thus the whole honeycomb film surface becomes hydrophilic. Water droplets on the surfaces transform from a Cassie state to a Wenzel state after 6–12 h PDA/PEI deposition, during which the apparent water contact angles decrease from above 90° (e.g., 115°) to below 90° (e.g., 55°). If the honeycomb-patterned porous films are not pre-wetted, PDA/PEI deposit only on the external surfaces, forming a top layer covering the pores, and the pore walls are not modified. This approach provides a versatile route for controlling water spreading and impregnation behaviors on patterned porous films, which will be useful in fields such as microreactors, supercapacitors, and wearable electronics.
Introduction
Ordered porous structures prepared by the breath figure method have attracted much attention in the past decades due to the wide range of applications, such as templating materials,1–5 superhydrophobic surfaces,6–11 biomaterials,12–16 separation membranes,17 and optical and energy materials.18–21 The process of the breath figure method is very simple. When a polymer in a volatile solvent is cast onto a substrate under a humid airflow, evaporation of the solvent leads to temperature reduction and hence results in condensation and growth of water droplets. At the same time, the polymer aggregates at the interface between solution and water to stabilize the water droplets. Through thermocapillary effect and Marangoni convection, water droplets arrange into close packed hexagonal arrays, and finally a film with ordered porous structure is obtained after the solvent and water are completely evaporated.22,23 Compared with other top-down patterning techniques, the bottom-up breath figure method is a cost-saving and environmentally friendly strategy, and is versatile to many film-forming materials.
The wettability of ordered porous surfaces plays an important role in the practical applications, such as anti-fouling surfaces and oil-water separation. It is well known that the roughness of a surface can enhance the hydrophobicity or hydrophilicity.24 If the honeycomb films are formed from hydrophobic polymer, it tends to form hydrophobic surfaces where water droplets rest only on the tops of the porous films, forming the so-called Cassie state.25 On the other hand, water droplets may impregnate the pores in films prepared from hydrophilic polymers, which is demonstrated as the Wenzel state.26 The Cassie–Wenzel state transition is very critical in many aspects such as water adhesion27,28 and site-specific functionalization.29 For example, the transition from the Cassie to the Wenzel state can facilitate pore filling of microspheres, which is an easy approach to functional microarrays.30,31 Ke et al.32 prepared positively charged honeycomb films by the breath figure method. Before pre-wetting, the film surface is at a Cassie state where the negatively charged silica nanoparticles can selectively assemble on the external surface of films; however, silica nanoparticles assemble on both external and internal surfaces of pre-wetted films at a Wenzel state. Consequently, by controlling the wetting states, nanoparticles selectively assemble inside or outside the pores. Rodríguez-Hernández et al.29 fabricated a honeycomb film from a fluorinated copolymer, and then made thiolated molecules attach specifically to the domain that is rich of ene group via thiol-para fluorine “click” reaction to achieve site-specific modification.
In addition to surface roughness, surface chemistry also plays a significant role in the wettability. Inspired by the strong adhesive protein in mussels, dopamine has been proved to be able to deposit on almost any substrates.33 The polydopamine (PDA) coating is a post modification platform that can perform Michael addition or Shiff base reaction with thiol or amino group, which makes it promising for controlling surface wettability by immobilizing various functional molecules onto the surfaces.33,34 Recently, Yang et al.35 reported a facile method to modify the surface wetting behavior via co-depositing PDA and polyetherimide (PEI). They found that the surface coated with PDA/PEI is more hydrophilic than that coated only by PDA. Also, the PDA/PEI layer is stable in acidic, neutral and strong basic solutions.
In this work, we prepared honeycomb-patterned porous films from a block copolymer polystyrene-block-poly(N,N-dimethylaminoethyl methacrylate) (PS-b-PDMAEMA) by the breath figure method, and then present a facile approach to the formation of conformal and non-conformal surface layers on the honeycomb films as shown in Scheme 1. These unique conformal and non-conformal functional layers are the results of controlling the surface wettability.
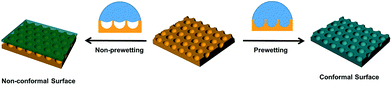 |
| Scheme 1 Honeycomb-patterned porous films without pre-wetting present a Cassie state which induce the formation of PDA/PEI layer above the honeycomb films and thus attain a non-conformal surface structure (left). Pre-wetted honeycomb films induce a Wenzel state, PDA/PEI will cover all over the honeycomb film surfaces, which makes the contribution to the conformal surface structure (right). | |
Experimental
Materials
The synthesis of polystyrene-block-poly(N,N-dimethylaminoethyl methacrylate) (PS247-b-PDMAEMA14, Mn = 27
900 g mol−1, Mw/Mn = 1.24) by atom transfer radical polymerization (ATRP) was described elsewhere.3 Dopamine hydrochloride (Sigma-Aldrich, AP), Tris(hydroxymethyl)aminomethane (Sigma-Aldrich), and polyetherimide (PEI, Mw = 600 g mol−1, Sigma-Aldrich) were used as received. Poly(ethylene terephthalate) (PET) films were provided by Hangzhou Tape Factory (China) and cleaned with acetone for 2 hours before use. Water used in the experiments was deionized and ultra-filtrated to 18.2 MΩ with an ELGA LabWater system. All other reagents were acquired from Sinopharm Chemical Reagent Co. Ltd. (China) and used without further purification.
Formation of honeycomb-patterned and pincushion-like films via the breath figure method
The breath figure method was operated according to our previously reported procedure.3 Briefly, PS247-b-PDMAEMA14 was dissolved in carbon disulfide (CS2) at a concentration of 2 mg mL−1 at room temperature. Then a 50 μL droplet of the polymer solution was drop-cast onto a PET substrate, which was placed under a 2 L min−1 humid airflow (∼25 °C and ∼80% RH). Owing to the evaporation of CS2, water in the humid airflow condensed onto the solution surface and arranged closely. After solidification, the film was dried at room temperature. The area of honeycomb-patterned films is about 1.5 cm2 and the thickness is about 2 μm. Pincushion-like films were prepared by peeling off the top layer of the honeycomb films using an adhesive Scotch tape. The top layer will be separated from the bottom layer at the thinnest and weakest position in the pore walls. As a result, the residual films on both the substrate and the adhesive tape have a pincushion-like structure. The films remaining on the substrate were used in this work.
Mussel-inspired surface modification by the co-deposition of dopamine/PEI
The co-deposition of dopamine/PEI was conducted at different concentration ratios. In a typical experiment, dopamine hydrochloride (2 mg mL−1) and PEI (1 mg mL−1) were dissolved in a Tris buffer solution (pH = 8.5, 50 mM). The honeycomb films were pre-wetted by immersing in ethanol for 1 min, and then immediately transferred into 10 mL of the freshly prepared dopamine/PEI solution. The samples were gently shaken for a designated time at room temperature. The samples were taken out from the solution, washed by deionized water for three times, and then dried in a vacuum oven at room temperature overnight.
Characterization
Water contact angles, drop contact diameter (CD), and volume of water droplets were measured using deionized water on a DropMeter A-200 contact angle system with digital image analysis software (MAIST Vision Inspection & Measurement Ltd Co.) at room temperature. To evaluate the time dependence of water droplets, the samples were placed in a home-made chamber, and typically 8 droplets were deposited around the measuring droplet beforehand to saturate the environment, which can minimize the effects of evaporation on the water contact angles. Field emission scanning electron microscope (FESEM, S-4800, Hitachi) was used to observe the surface morphology of films at an accelerating voltage of 3 kV. Prior to observation, the samples were sputtered with gold using an ion sputter JFC-1100. X-ray photoelectron spectroscopy (XPS) experiments were carried out on a PHI-5000C ESCA system (Perkin Elmer, USA) with Al Kα radiation (1486.6 eV).
Results and discussion
Mussel-inspired surface modification of honeycomb-patterned porous films
Honeycomb-patterned porous films were prepared by the breath figure method under an optimal condition. The surface and cross-sectional morphology of the films were characterized using FESEM (Fig. 1, S1 and S2 in ESI†). Some particles are found on the film surface when dopamine is solely deposited for 24 hours (Fig. 1b). By contrast, no obvious aggregates can be seen on the film surfaces co-deposited with PDA and PEI (Fig. 1c–e). This interesting phenomenon is induced by the destruction of PDA aggregates from covalent interaction between PDA and PEI.35 Before being precipitated on the surface, dopamine will react with amino groups of PEI first and thus decrease the number of PDA particles in the co-deposition process.36 Although the PDA aggregates are too small to fully block the pores of honeycomb films, it is detrimental to a honeycomb film where a smooth outer surface is preferential. By removing the top layer of honeycomb films, highly ordered pincushion-like structures can be obtained. The pincushion-like structure also remains after the co-deposition of PDA/PEI (Fig. 1f). XPS results support the surface modification (Fig. S3†).
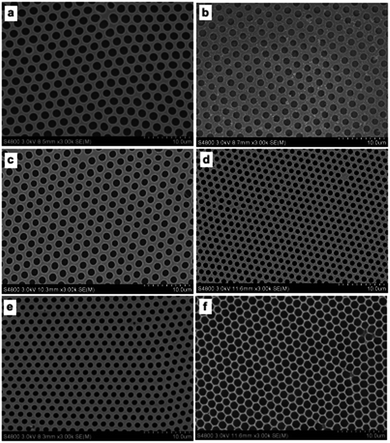 |
| Fig. 1 Top-down SEM images of nascent (a) and modified honeycomb-patterned porous films with different concentrations of dopamine/PEI solutions: (b) 2/0, (c) 2/0.5, (d) 2/1, (e) 2/2. (f) Pincushion-like surface. Deposition time is 24 h. | |
The effects of co-deposition concentration and time on the wettability of the honeycomb-patterned films were investigated. As shown in Fig. 2a, the apparent water contact angles decrease slowly after the addition of PEI, and then increase dramatically to about 90–98°. This change in apparent water contact angles is due to the composition variation of the honeycomb film surfaces after modification. The surfaces become more and more hydrophilic when the content of PEI, which has high surface energy, increases from 0 to 1 mg mL−1. However, as excess PEI was added in the co-deposition system, too high concentration of amino group will hinder the self-polymerization and assembly of dopamine, which is unfavorable to the formation of PDA coating on honeycomb film surfaces. A similar phenomenon has also been found in the co-deposition of dopamine with thiol.19 Furthermore, we investigated the effect of co-deposition time on the apparent water contact angles with fixed 2 mg mL−1 dopamine and 1 mg mL−1 PEI. As shown in Fig. 2b, the apparent water contact angles decrease gradually with deposition time. The hydrophobic surfaces change into hydrophilic surfaces with an apparent water contact angle of 52° after 24 h co-deposition.
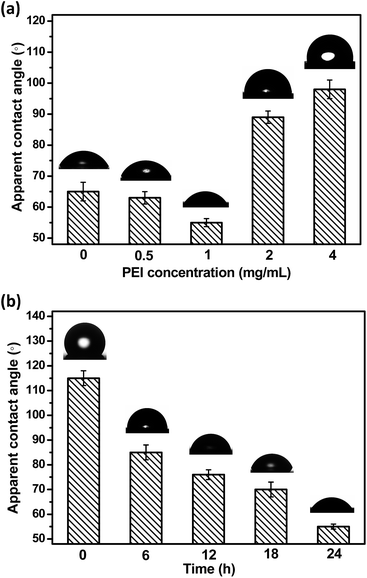 |
| Fig. 2 (a) Effects of PEI concentration on apparent water contact angles of honeycomb-patterned porous films. All the films are fully pre-wetted with ethanol before deposition. Dopamine concentration is 2 mg mL−1. (b) Effects of co-deposition time on apparent water contact angles of honeycomb-patterned porous films. Dopamine/PEI = 2/1 mg mL−1. | |
Surface wettability from the Cassie state to the Wenzel state
It is known that a flat polystyrene film has a water contact angle of about 89°. As a result of large area fraction of pores, the honeycomb film surface has an apparent water contact angle of 115° (Fig. S4†). It is well known that a highly porous film can entrap air and stay at the Cassie state, or the metastable Cassie state (a transition state from Cassie state to Wenzel state). The apparent contact angles (θM) can be theoretically calculated using the Cassie–Baxter's law:16
cos θM = (1 − fpore) × cos θpolymer + fpore × cos θpore |
where fpore is the area fraction of pores at the surface, θpolymer is the water contact angle on a flat and smooth polymer film (∼89° for a PS film), and θpore is the water contact angle on the pore, which is 180° when the water droplet is in the Cassie state. The fpore value can be estimated from the SEM images shown in Fig. 1, which is 0.43–0.50. The calculated water contact angles (114.8–119.4°) are close to the experimental result (117°), indicating that the film is in the Cassie state. If the honeycomb film was converted into a pincushion-like surface, which has a pore area fraction more than 80%, the water contact angle on the surfaces increases to 135° (Fig. S4†). This value is slightly smaller than the theoretically calculated value (145°), indicating a metastable Cassie state.
The wetting state is easy to be transformed to the Wenzel state when modified by PDA/PEI co-deposition. We systematically investigated the effects of co-deposition time on apparent water contact angles, volume ratio of water droplets, and drop contact diameter (CD). In Fig. 3a, the apparent water contact angle shows no change with time on the nascent film surface within 100 seconds, which indicates that on the film surface water droplets are very stable in the Cassie state. Volume ratio, the ratio of the real time water volume on a film surface to the initial water volume of droplet, is used to evaluate the change in the size of water droplets. There are two main factors that attributed to water loss to decrease the volume ratio, one is the evaporation and the other is the impregnation of water into the pores of the honeycomb films. The volume ratio slowly decreases to 0.97 almost linearly in 100 seconds on the nascent honeycomb film surface. The 3% decrease is mainly due to evaporation of water although a nearly saturated environment was created. At the same time, the drop CD changes little (Fig. S5†). However, apparent water contact angles decrease dramatically to a low value on films modified with PDA/PEI for 6, 12, 18, and 24 hours (Fig. 3b–e), especially on the last two modified films which show highly hydrophilic property (apparent water contact angle < 30°). Besides the apparent water contact angles, the volume ratios also decrease with water spreading on the modified surfaces. The volume ratio of a nascent film shows a linear decline of about 3% with time. The volume of water droplets on the PDA/PEI-modified film surfaces presents a non-linear decline. Moreover, about 8% decrease of water volume can be observed, which is obviously larger than the 3% on the nascent film surface.
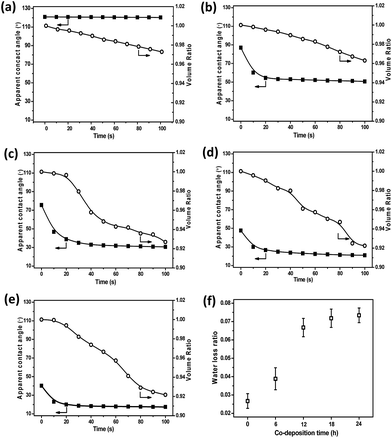 |
| Fig. 3 Effects of co-deposition time on apparent water contact angles and volume ratio. Dopamine/PEI = 2/1 mg mL−1 (a–e) Films modified with PDA/PEI for 0, 6, 12, 18, and 24 hours, respectively. (f) Water loss ratio evolution on PDA/PEI coated films after water drop on the films for 100 second. | |
The water loss ratio (Rl) was calculated according to the following equation,
where
Vd is the volume of the initial water droplet at time zero and
Vm is the volume of water droplet at 100 seconds; both
Vd and
Vm are measured by the contact angle instrument.
Fig. 3f shows that the water loss ratios increase with co-deposition time, and then remain almost stable on the films modified with PDA/PEI for 18 and 24 hours. This phenomenon indicates the transition from the Cassie state to the Wenzel state changes dramatically on film surfaces modified PDA/PEI for more than 6 hours. The reason is that the deposition solution is easily to entry into the pores after pre-wetting the nascent honeycomb films with ethanol, making the PDA/PEI deposit inside the pores. Consequently, the water droplets tend to impregnate into the pores of hydrophilized films.
Conformal and non-conformal surface modification
The above-mentioned results indicate that the PDA/PEI-deposited honeycomb surfaces show two wetting states, i.e., Cassie and Wenzel states, which depends on the degree of hydrophilization. The original honeycomb-patterned porous films are prepared from hydrophobic PS, on which a water droplet will stay at the Cassie state. With the pre-wetting step, the pores of honeycomb films can be wetted by the deposition solution. As a result, both the top surface and the pore surface of the films can be modified. Where will the PDA/PEI deposit without the pre-wetting step?
If we carefully control the wetting state of the honeycomb-patterned porous film surface, we can realize site-specific functionalization and special structure of honeycomb films.37 We investigated the effects of pre-wetting conditions as shown in Table 1. The film surfaces without pre-wetting by ethanol present a Cassie state; the entrapped air prevents water from entering into the pores and thus induces the formation of a flat PDA/PEI layer above the honeycomb films (Fig. 4a–c). Results of the cross-sectional images (inset in Fig. 4c) also confirm the non-conformal deposition; the pore walls are highly smooth without any particles of PDA. The water contact angle (∼66°) on the non-conformal surfaces is close to the value of a PDA/PEI free-standing film.38 And, water droplets cannot impregnate into pores through the PDA/PEI film. Such unique non-conformal layer on the pores will be useful in some interesting fields such as microreactors.
Table 1 Experimental conditions for conformal and non-conformal surface modification
Sample |
Pre-wetting time/(min) |
PDA : PEI |
Deposition time/(h) |
a |
0 |
2 : 0.5 |
6 |
b |
0 |
2 : 1 |
12 |
c |
0 |
2 : 1.5 |
18 |
d |
5 |
2 : 0.5 |
12 |
e |
5 |
2 : 1 |
18 |
f |
5 |
2 : 1.5 |
6 |
g |
60 |
2 : 0.5 |
18 |
h |
60 |
2 : 1 |
6 |
i |
60 |
2 : 1.5 |
12 |
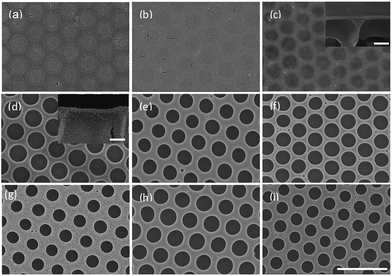 |
| Fig. 4 Top-down SEM images of honeycomb-patterned porous prepared in accordance with Table 1 (a–i). The inset images are the cross section of films (c and d). The top-down SEM images scale bar is 10 μm, and the cross section scale bar is 500 nm. | |
On the other hand, the pre-wetted honeycomb films by ethanol induce a Cassie–Wenzel transition and hence allow infiltration of aqueous suspension into the pores. This operation can realize the co-deposition of PDA/PEI inside the pores as well as on the outer surfaces. The conformal structure of PDA/PEI layer can be confirmed by the cross-sectional image (inset in Fig. 4d) where PDA particles can be observed obviously at high magnification. Pre-wetting with ethanol for 5 minutes is similar with that for 60 minutes. Such conformally structured films could be in blockage of electronic leakage in supercapacitors and wearable electronics.39,40
Conclusions
In summary, we have developed a facile and robust approach to conformal and non-conformal surface modification by tuning Cassie–Wenzel transition on honeycomb-patterned porous films. The films surfaces without pre-wetting present a Cassie state and induce the formation of PDA/PEI layer on the honeycomb films. Thus, non-conformal surface structure is obtained. On the other hand, the pre-wetted honeycomb films by ethanol induce the Wenzel state, PDA/PEI covers all over the honeycomb film surfaces, leading to a conformal surface structure. This approach provides a versatile route for controlling the morphology of porous films, which may be useful in fields such as microreactors, supercapacitors, and wearable electronics.
Acknowledgements
Financial support from the National Natural Science Foundation of China (51522305 and 21374100), Zhejiang Provincial Sci & Tech Plan of China (2015C31028) and the Fundamental Research Funds for the Central Universities (2015XZZX004-26) is gratefully acknowledged.
Notes and references
- L. A. Connal and G. G. Qiao, Adv. Mater., 2006, 18, 3024–3028 CrossRef.
- H. Yabu, Y. Matsuo, K. Ijiro, F. Nishino, T. Takaki, M. Kuwahara and M. Shimomura, ACS Appl. Mater. Interfaces, 2010, 2, 23–27 Search PubMed.
- Y. Ou, L.-W. Zhu, W.-D. Xiao, H.-C. Yang, Q.-J. Jiang, X. Li, J.-G. Lu, L.-S. Wan and Z.-K. Xu, J. Phys. Chem. C, 2014, 118, 4403–4409 Search PubMed.
- L. Li, Y. Zhong, C. Ma, J. Li, C. Chen, A. Zhang, D. Tang, S. Xie and Z. Ma, Chem. Mater., 2009, 21, 4977–4983 CrossRef.
- J.-L. Gong, B.-G. Xu and X.-M. Tao, RSC Adv., 2015, 5, 14341–14344 RSC.
- H. Yabu, M. Takebayashi, M. Tanaka and M. Shimomura, Langmuir, 2005, 21, 3235–3237 CrossRef PubMed.
- P. S. Brown, E. L. Talbot, T. J. Wood, C. D. Bain and J. P. S. Badyal, Langmuir, 2012, 28, 13712–13719 CrossRef PubMed.
- H. Yabu and M. Shimomura, Chem. Mater., 2005, 17, 5231–5234 CrossRef.
- T. Guo, K. Han, L. Heng, M. Cao and L. Jiang, RSC Adv., 2015, 5, 88471–88476 RSC.
- Z. Li, X. Ma, D. Zang, Q. Hong and X. Guan, RSC Adv., 2015, 5, 21084–21089 RSC.
- V. V. Tsukruk, Rubber Chem. Technol., 1997, 70, 430–467 CrossRef.
- A. Sanz de Leon, J. Rodriguez-Hernandez and A. L. Cortajarena, Biomaterials, 2013, 34, 1453–1460 CrossRef PubMed.
- T. Nishikawa, M. Nonomura, K. Arai, J. Hayashi, T. Sawadaishi, Y. Nishiura, M. Hara and M. Shimomura, Langmuir, 2003, 19, 6193–6201 CrossRef.
- S. Yamamoto, M. Tanaka, H. Sunami, E. Ito, S. Yamashita, Y. Morita and M. Shimomura, Langmuir, 2007, 23, 8114–8120 CrossRef PubMed.
- X. H. Wu, Z. Y. Wu, J. Qian, Y. G. Yan, J. Wei, H. Li and J. C. Su, RSC Adv., 2015, 5, 36007–36014 RSC.
- X. H. Wu, Z. Y. Wu, J. C. Su, Y. G. Yan, B. Q. Yu, J. Wei and L. M. Zhao, RSC Adv., 2015, 5, 6607–6616 RSC.
- L. S. Wan, J. W. Li, B. B. Ke and Z. K. Xu, J. Am. Chem. Soc., 2012, 134, 95–98 CrossRef PubMed.
- L. Heng, W. Qin, S. Chen, R. Hu, J. Li, N. Zhao, S. Wang, B. Z. Tang and L. Jiang, J. Mater. Chem., 2012, 22, 15869 RSC.
- L. Song, R. K. Bly, J. N. Wilson, S. Bakbak, J. O. Park, M. Srinivasarao and U. H. Bunz, Adv. Mater., 2004, 16, 115–118 CrossRef.
- A. K. Singh, R. P. Pandey and V. K. Shahi, RSC Adv., 2014, 4, 22186–22193 RSC.
- H. Yabu, K. Nagamine, J. Kamei, Y. Saito, T. Okabe, T. Shimazaki and M. Nishizawa, RSC Adv., 2015, 5, 88414–88418 RSC.
- G. Widawski, M. Rawiso and B. Francois, Nature, 1994, 369, 387–389 CrossRef CAS.
- L. S. Wan, L. W. Zhu, Y. Ou and Z. K. Xu, Chem. Commun., 2014, 50, 4024–4039 RSC.
- X. Zhang, F. Shi, J. Niu, Y. Jiang and Z. Wang, J. Mater. Chem., 2008, 18, 621–633 RSC.
- A. Cassie and S. Baxter, Trans. Faraday Soc., 1944, 40, 546–551 RSC.
- R. N. Wenzel, Ind. Eng. Chem., 1936, 28, 988–994 CrossRef CAS.
- L. Heng, X. Meng, B. Wang and L. Jiang, Langmuir, 2013, 29, 9491–9498 CrossRef CAS PubMed.
- X. Yu, Q.-Z. Zhong, H.-C. Yang, L.-S. Wan and Z.-K. Xu, J. Phys. Chem. C, 2015, 119, 3667–3673 CAS.
- A. S. de León, A. d. Campo, C. Labrugère, M. Fernández-García, A. Muñoz-Bonilla and J. Rodríguez-Hernández, Polym. Chem., 2013, 4, 4024 RSC.
- L. S. Wan, J. Lv, B. B. Ke and Z. K. Xu, ACS Appl. Mater. Interfaces, 2010, 2, 3759–3765 CAS.
- X. Li, L. Zhang, Y. Wang, X. Yang, N. Zhao, X. Zhang and J. Xu, J. Am. Chem. Soc., 2011, 133, 3736–3739 CrossRef CAS PubMed.
- B. B. Ke, L. S. Wan, P. C. Chen, L. Y. Zhang and Z. K. Xu, Langmuir, 2010, 26, 15982–15988 CrossRef CAS PubMed.
- H. Lee, S. M. Dellatore, W. M. Miller and P. B. Messersmith, Science, 2007, 318, 426–430 CrossRef CAS PubMed.
- S. Hong, C. F. Schaber, K. Dening, E. Appel, S. N. Gorb and H. Lee, Adv. Mater., 2014, 26, 7581–7587 CrossRef CAS PubMed.
- H.-C. Yang, K.-J. Liao, H. Huang, Q.-Y. Wu, L.-S. Wan and Z.-K. Xu, J. Mater. Chem. A, 2014, 2, 10225–10230 CAS.
- H. Wang, J. Wu, C. Cai, J. Guo, H. Fan, C. Zhu, H. Dong, N. Zhao and J. Xu, ACS Appl. Mater. Interfaces, 2014, 6, 5602–5608 CAS.
- U. H. F. Bunz, Adv. Mater., 2006, 18, 973–989 CrossRef CAS.
- H.-C. Yang, W. Xu, Y. Du, J. Wu and Z.-K. Xu, RSC Adv., 2014, 4, 45415–45418 RSC.
- S. Choi, J. Park, W. Hyun, J. Kim, J. Kim, Y. B. Lee, C. Song, H. J. Hwang, J. H. Kim, T. Hyeon and D. H. Kim, ACS Nano, 2015, 9, 6626–6633 CrossRef CAS PubMed.
- Y. Ma, C. Fang, B. Ding, G. Ji and J. Y. Lee, Adv. Mater., 2013, 25, 4646–4652 CrossRef CAS PubMed.
Footnote |
† Electronic supplementary information (ESI) available: Top-down and cross-sectional SEM images; XPS spectras; apparent water contact angles; effects of co-deposition time on drop CD. See DOI: 10.1039/c6ra08606c |
|
This journal is © The Royal Society of Chemistry 2016 |
Click here to see how this site uses Cookies. View our privacy policy here.