DOI:
10.1039/C6RA08404D
(Paper)
RSC Adv., 2016,
6, 53804-53812
Synthesis and characterization of PLGA nanoparticle/4-arm-PEG hybrid hydrogels with controlled porous structures
Received
1st April 2016
, Accepted 26th May 2016
First published on 27th May 2016
Abstract
Controlled porous structures, adjustable surface properties and good mechanical properties are essential for hydrogels in promoting cell adhesion and growth. In this work, we developed four armed poly(ethylene glycol) (4-arm-PEG) hydrogels crosslinked by poly(lactic-co-glycolic acid) nanoparticles (PLGA NPs). Branched polyethyleneimine (b-PEI) was employed for aminolysis of PLGA to engineer 300, 530 and 1000 nm NPs with a nitrogen contents of 11.7%, 9.4% and 9.7%. Hybrid hydrogels were formed by crosslinking amino-packed NPs with polymeric chains of 4-arm-PEG-NHS. By manipulating the NP and PEG contents as well as the NP sizes, the pore sizes could be tailored from 10–20 μm to 20–40 μm and 100–200 μm, and the maximal compressive strength could be optimized to 0.37 MPa. Cytotoxicity trials indicated the hydrogels were almost non-toxic and biocompatible. Cell adhesion evaluation testified higher amino contents and a smaller proportion of PEG led to more cell attachment. These results demonstrated that this kind of hybrid hydrogel may be a suitable candidate for further biomedical applications in tissue engineering.
Introduction
Hydrogels are a kind of biomimetic material in terms of high water content, insolubility, biocompatibility and highly tunable mechanical properties which can provide temporary supports for cells and vehicles for drug delivery and thus have been widely studied as promising candidates for tissue engineering.1–4 Numerous natural and synthetic hydrogels, or a combination of both have been investigated for tissue regeneration due to their distinct properties, and their three-dimensional (3D) structures have a significant effect on the biological activities.5 Generally, the interconnected porous structure allows cell rapid growth and migration as well as enhances nutrient diffusion or waste removal.6–9 Meanwhile, the surface properties like roughness, topography, and chemical groups also highly affect adhesion, growth and migration of the cells.10,11 Accordingly, there is a need for hydrogels with pores of several decades to hundred micrometres in diameters, which are large enough to allow cell ingrowth.12 If the pores are too small, cell growth and migration are limited. Conversely, the too large pores that cause the decrease in the surface area are not favourable for the cell adhesion.13 Moreover, cell adhesion also highly depends on the surface properties of hydrogels. For example, the surfaces covalently grafting amines, collagen, RGD, fibronectin and other biological activities can be conducive to the attachment of cells.14,15 Therefore, maintaining a balance between the optimal pore size for cell migration and special surface chemical characteristics for cell attachment is essential. So, the design of a hydrogel scaffold with controlled porous structures, adjustable surface properties and good mechanical properties is extremely necessary.
Many efforts have been taken to design hydrogels to meet the requirements for cell adhesion, growth and migration.16,17 Recently, a new method has been explored for producing NP-crosslinked hydrogel scaffolds with well-interconnected pores and excellent mechanical properties.18,19 Dan Moro et al. developed a temperature sensitive three-dimensional network by covalently crosslinking PNIPAM-co-AA nanoparticles for drug release.12 Kazunari Akiyoshi et al. reported the acryloyl group modified cholesterol-bearing pullulan nanogel tectonic porous gel for advanced scaffold.20 Biodegradable and biocompatible polyesters such as poly(lactic-co-glycolic acid) (PLGA) are popular materials for the manufacture of tissue engineering scaffolds. Recently, PLGA NPs are entrapped in the hydrogel scaffold physically to provide efficient drug delivery systems.21 However, chemical hydrogels crosslinked by PLGA NPs are seldom reported.
It is well-known that the functional groups are absent in the backbone of the PLGA, which makes it difficult to form chemical hydrogels crosslinked through the PLGA NPs. A lot of approaches have been taken to modify the surface of PLGA NPs including plasma treatment and surface entrapment.22–24 According to our previous reports, amino groups can be easily introduced into the surfaces of PLGA NPs by an ammonia plasma treatment technique, it can not only enhance the wettability of the surface but also equip a positively charge surface which are beneficial for the cells adhesion.23 But the ammonia plasma treated NPs possess less amino contents or shorter amino chains which are not enough for further reaction. Aminolysis is a technology which has been used in the textile industry for many years.25,26 So far, aminolysis technology has been used by Cooper-White groups to modify the biodegradable polyester membranes, and introduce amine groups onto the surface with minimal degradation to make the materials suitable for directed tissue growth.27
In the work, we prepare three uniform PLGA NPs with 300, 530 and 1000 nm by microfluidics and premix membrane emulsification (PME) technologies. After treatment of b-PEI with various minutes, primary and secondary amines were attached in the surface of these PLGA NPs in a controllable manner (Scheme 1). The b-PEI modified PLGA NPs have good water dispersity. When mixing the NP dispersion with active terminal 4-arm-PEG-NHS, fast crosslinking reaction between active ester and amino groups facilely form PLGA nanoparticle/4-arm-PEG hybrid chemical hydrogels. Then the structures, mechanical properties and swelling properties of the hydrogels were systematically examined, as well as their cell cytotoxicity and effectiveness in promoting cell adhesions.
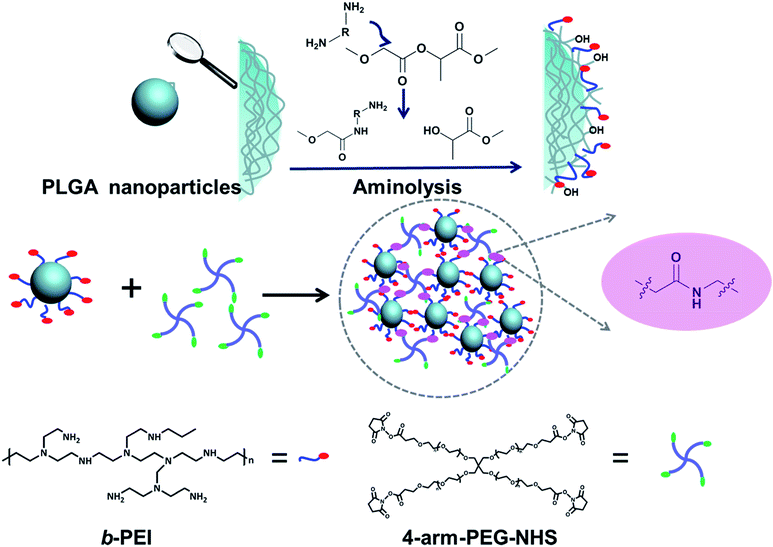 |
| Scheme 1 Schematic for formation of b-PEI modified PLGA nanoparticles and their hybrid hydrogels. | |
Results and discussion
Preparation of PLGA NPs
The size of NPs had an effect on the morphology and mechanical properties of the hybrid hydrogels. High special surface energy of the small nanoparticles diminished the dispersion of the NPs suspension.28 Compared with the PME method, microfluidics performed a high efficiency on preparing small NPs. Therefore, microfluidics was adopted to produce 300 nm PLGA NPs, but PME was used to prepare 530 and 1000 nm PLGA NPs. The morphology and size distribution of the PLGA NPs were measured by scanning electron microscopy (SEM) and dynamic light scatting (DLS). Fig. 1a–c showed that all the PLGA NPs obtained were uniform, and their surfaces were dense and smooth. Fig. 1d–f further demonstrated the PLGA NPs possessed a narrow polydispersity to be 0.182, 0.048 and 0.107, respectively.
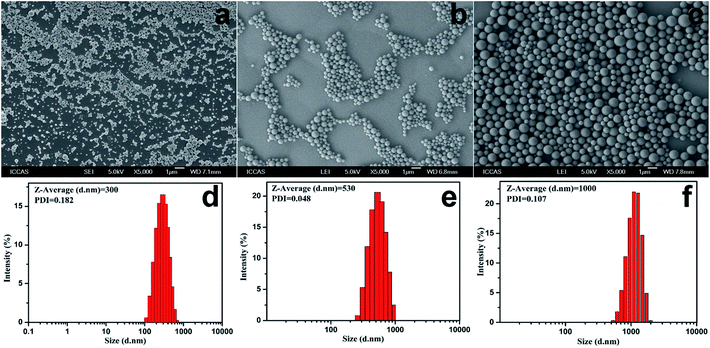 |
| Fig. 1 (a–c) SEM images and (d–f) DLS results of PLGA NPs produced by (a and d) microfluidics and (b, e and c, f) PME methods. | |
Aminolysis
Jiacong Shen et al. employed PEI to engineer PDL-LA substrate to obtain a stable positively charged surface,22 and high molecule weight of PEI was always used as gene transfection agent or functionalized the surface of PLGA NPs for gene silencing.29,30 In this study, b-PEI was successfully grafted to the surface of NPs via aminolysis to obtain an amino packed surface. Change of surface charges was measured by zeta potential analysis, while the number of nitrogen content was quantified using EDX. Fig. 2a showed unmodified nanoparticles exhibited a negative zeta potential arising from the surface hydroxyl groups, but b-PEI modified nanoparticles had a positively charged surface, indicating successful deposition of b-PEI onto the surfaces of PLGA NPs. With the elongation of treatment time, the surface zeta potential firstly increased and then decreased, which may ascribe to that the partial surfaces were eroded into small dissoluble molecules. EDX analysis can directly provide detailed element information of NP surfaces. Table 1 manifested no nitrogen was detected on the untreated NP surface, but after b-PEI modification, the nitrogen increased to be 11.7%, 9.4% and 9.7% for 300, 530 and 1000 nm NPs, which was consistent with the results of zeta potential. For the PLGA NPs, the theoretical O/C ratio is 0.74, but after aminolysis the O/C ratio decreased with the introduction of PEI. SEM images in Fig. 2b and c revealed no obvious change of surface morphology before and after PEI treatment. These results demonstrated that the NPs could be armed with more contents of amino groups in a highly controlled manner without destroying the surface morphology by the b-PEI modified method.
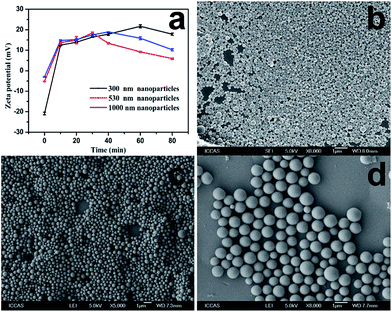 |
| Fig. 2 (a) Zeta potentials of 300, 530 and 1000 nm PLGA NPs after b-PEI aminolysis. SEM images of (b) 300, (c) 530 and (d) 1000 nm PLGA NPs at maximal zeta potential. | |
Table 1 Atomic compositions of untreated and b-PEI modified PLGA NPs as determined via EDX
Sample |
C (at%) |
N (at%) |
O (at%) |
O/C |
O/N |
PLGA |
57.5 |
0 |
42.6 |
0.74 |
— |
300 nm–60 min |
69.7 |
11.7 |
24.6 |
0.35 |
2.10 |
530 nm–30 min |
66.9 |
9.4 |
23.8 |
0.36 |
2.54 |
1000 nm–40 min |
77.6 |
9.7 |
12.7 |
0.16 |
1.30 |
Formation of hybrid hydrogels
To produce hybrid hydrogels, we mixed the aqueous solution of 4-arm-PEG-NHS with the amino functionalized NP suspension, followed by vortex at room temperature. Since reaction between active ester and amino group is very fast, the hydrogels could be obtained rapidly via in situ formation of amide crosslinks. The process of producing hybrid hydrogels was simply summarized in Scheme 1. Here, PLGA NPs as the multiple crosslinking points linked 4-arm-PEG together to form the network structure. On-site gelation of hybrid hydrogels was examined by the test tube inversion method (Fig. 3, 530 nm). No gelation was observed for 1000 nm PLGA NP even its content was increased to 10 wt%, which may ascribe to that short polymer strands were difficult to be bridged by the large sizes of NPs.31 In comparison, for 530 nm NPs with the solid contents to be 7.5 wt% and 10 wt%, the hydrogels were formed immediately (less than 10 s) when mixing the 4-arm-PEG-NHS solution (2.5 wt% or 5 wt%). But if the NP content was less than 5 wt%, these NPs could not effectively bridge the 4-arm-PEG to form the complete crosslinking network. For 300 nm NPs, we could still produce the hybrid hydrogels quickly through mixture of 5 wt% of NPs and 2.5 wt% of 4-arm-PEG-NHS solutions, which was denoted as NP3005-PEG2.5. It must note that all hybrid hydrogels were prepared with the condition of excessive amino contents.
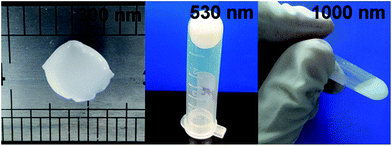 |
| Fig. 3 Photographs of hybrid hydrogels formed by various PLGA NPs. | |
Morphologies of freeze-dried hybrid hydrogels
The SEM micrographs of the cross-sectional morphologies of the hybrid hydrogels as a function of NPs content, diameter and the concentration of the polymer were summarized in Fig. 4. The results demonstrated that the hydrogels possessed porous network structures which could provide numerous channels for water to enter into or squeeze out. Fig. 4 also depicted the pores inside the hydrogel matrix turned smaller and denser significantly with the increasing of the NP and PEG contents. For example, the pore sizes of the NP3005-PEG2.5 and NP3007.5-PEG2.5 hydrogels were 20–40 μm, but decreased to 10–20 μm with the PEG content increasing into 5 wt%. The results indicated that with the increase of the concentration of 4-arm-PEG-NHS, the higher crosslinking degree was achieved. In addition, increased NP sizes significantly enlarged the pore sizes of the formed hydrogels, e.g., the size increased from 20–40 μm to 100–200 μm using the 530 nm NPs to replace 300 nm NPs. So, the pore sizes of hybrid hydrogels could be tuned by adjusting the NP and PEG contents as well as the NP sizes. Since the pore size of hydrogels was relative to the cells growth, the hydrogel scaffolds may be customized for applications in tissue engineering.32
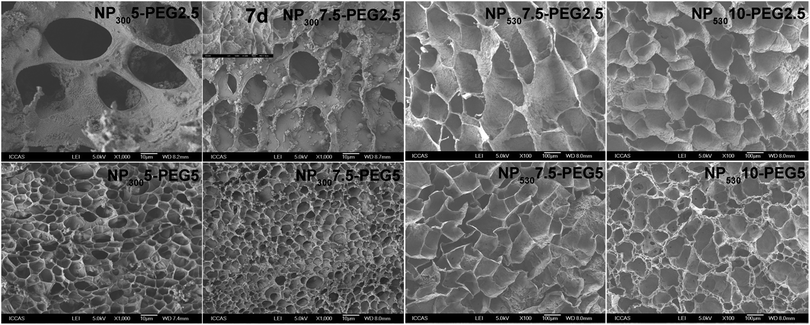 |
| Fig. 4 SEM images of cross-sectional morphologies of hybrid hydrogels formed by PLGA NPs with various feed ratios. NP300m-PEGn meant the hybrid hydrogel was formed by mixing m% 300 nm PLGA NPs and n% 4-arm-PEG-NHS. Inset: SEM image of the NP3007.5-PEG2.5 hydrogel after being immersed in water for 7 days. | |
Equilibrium swelling ratio (ESR) of hybrid hydrogels
ESR was one of the most important properties of hydrogels, and in particular, high swelling ratios were preferred in many applications.33 The ESR was measured by soaking the hydrogels in water to reach the balance. Fig. 5 clearly showed that the ESR decreased with the increasing content of NPs or PEG. The result was reasonable because the increasing content of NPs or PEG facilitated to form a denser network as depicted in the results of the SEM, causing the decrement of its ESR. With the same contents of NPs and PEG, larger NPs yielded higher ESR like 10.9 and 11.7 for NP3007.5-PEG2.5 and NP5307.5-PEG2.5, which may be attributed to larger NPs with less amino contents led to formation of loose networks with lower crosslinking degrees. The weight of the hydrogels exhibited some extent of reduction after being immersed into the water for a long time. As shown in Fig. 4, the porous network structures of the NP3007.5-PEG2.5 hydrogel were collapsed compared with the original SEM morphology after being immersed in water for 7 days. It could be explained that the hydrogel had a certain degree degradation.
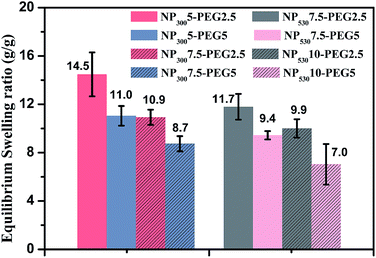 |
| Fig. 5 ESR profiles of various hybrid hydrogels. | |
Mechanical properties of hybrid hydrogels
Fig. 6a and b showed the stress–strain curves of the hybrid hydrogels at room temperature. The compressive strengths of NP3005-PEG2.5, NP3005-PEG5, NP5307.5-PEG2.5 and NP5307.5-PEG5 hydrogels were 50.8 ± 4.6, 79.5 ± 2.9, 152.2 ± 10.2 and 256.7 ± 13.6 kPa. When increasing the NP content, the compressive strengths increased to 101.3 ± 6.6, 101.4 ± 3.4, 215.0 ± 7.6 and 367.4 ± 10.7 kPa for NP3007.5-PEG2.5, NP3007.5-PEG5, NP53010-PEG2.5 and NP53010-PEG5 hydrogels. The results obviously demonstrated that relatively dense and rigid networks of the hydrogels along with the increasing of NP content and polymer concentration could enhance their compressive strength. It was reported that well-connected small pores could effectively prevent the crack propagation because the stress distributed more evenly throughout the surfaces with smaller pore structures.34 When the hybrid hydrogels had the same NP content, the larger NPs leaded to the higher strain like 84.9% and 89.9% for NP3007.5-PEG2.5 and NP5307.5-PEG2.5. In general, the maximal compressive strength could reach 0.37 MPa at a strain of 74.5% (Fig. 6b). These results showed that the NPs played a key role in tuning the pore size thereby affecting the mechanical properties of the hybrid hydrogels.
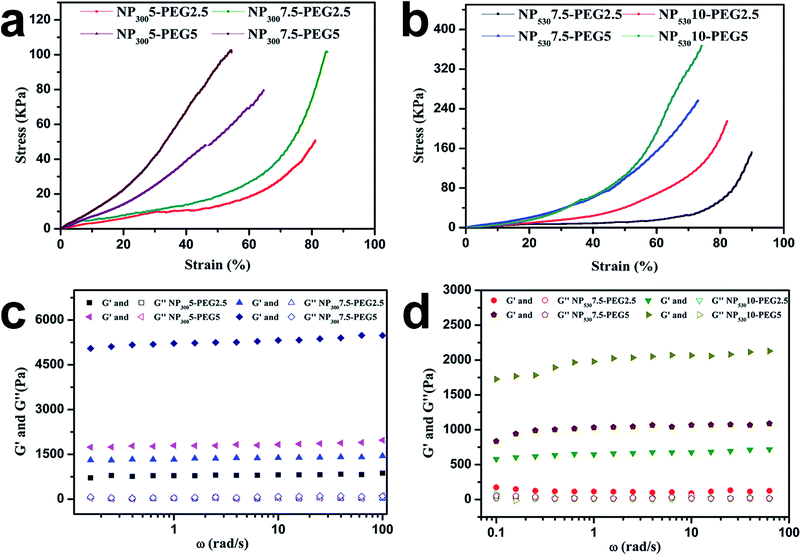 |
| Fig. 6 (a and b) Stress–strain curves under uniaxial compression and (c and d) dynamic frequency-sweep measurement of hybrid hydrogels with different feed ratios. | |
Rheological characterization
The frequency-sweep viscoelasticity measurements at γ = 0.5% were performed at room temperature with angular frequencies ranging from 10−1 to 102 rad s−1. Storage modulus (G′) and loss modulus (G′′) were documented as depicted in Fig. 6c and d. For all the samples, the G′ values were independent on frequency over the test range and higher than G′′ values, indicating an elastic solid-like behaviour of crosslinked hybrid hydrogels.35 It was also observed that the storage modulus increased with the increase of NP content and polymer concentration. This was due to the fact that with the increase of NPs content and polymer concentration, the networks of the hybrid hydrogels became denser, resulting in a rise of the strength of hybrid hydrogels. Besides, with the increase of NP size, the storage modulus decreased obviously, whereas the loss modulus almost kept unchangeable, indicating a decrease of elasticity. These results demonstrated that rheological properties of the hybrid hydrogels relied on the polymer concentration, NP size and content.
Cytotoxicity and cell adhesion
The results of CCK-8 assay for cytotoxicity test of various hybrid hydrogels were shown in Fig. 7. The relative cell vitalities of MC3T3-E1 cells incubated with all the hybrid hydrogels after 48 h was more than 90%, indicating that the hybrid hydrogels were non-toxic to the MC3T3-E1 and suitable as extracellular matrix for cell growth. It was well known that the proportion of PEG segment, amino content and pore size were three significant contributors to the MC3T3-E1 model cell adhesion.24,36–38 It was well documented that implanted materials were immediately coated with proteins from blood and interstitial fluids, so it was the adsorbed proteins, instead of the surface itself, to which cells initially responded.39 The amino groups could effectively interact with peptide chains to absorb the proteins, therefore, more amines on the surfaces were favourable to enhance cell attachment.24,40 Fig. 8 showed more MC3T3-E1 cells were attached on the hydrogel substrates with 2.5 wt% PEG content than those with 5 wt% PEG content, testifying decreased proportion of PEG segments facilitated cell adhesion. Fig. 8 also depicted that very large pore size (100–200 μm) resulted from the 530 nm NP hybrid hydrogels with less amino contents was not favourable to attach cell in the hydrogel matrix. Among all the crosslinking of NPs and PEG, amino content was always superfluous. Therefore, the hybrid hydrogels of the 300 nm NPs had more amino residues, which would be helpful to enhance the cell adhesion. The results clearly demonstrated PEG and amino contents could significantly influence the cell adhesion on the hybrid hydrogels.
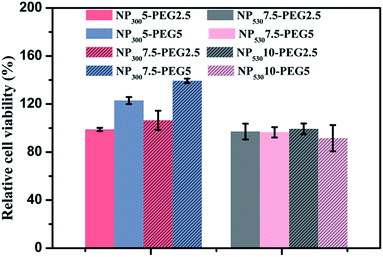 |
| Fig. 7 CCK-8 results of MC3T3-E1 cells seeded on the various hybrid hydrogels with culture time of 48 h. | |
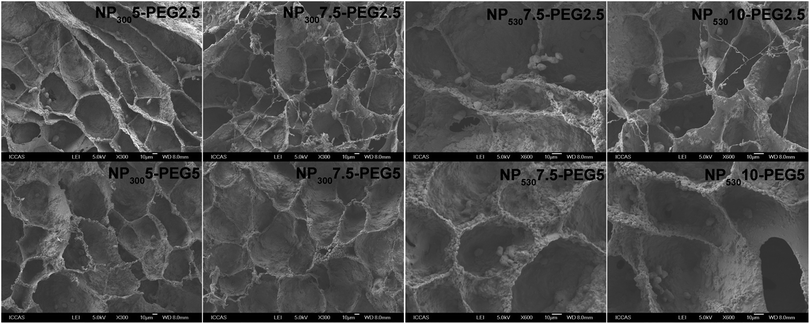 |
| Fig. 8 SEM images of various hybrid hydrogels after cell adhesion. | |
Experimental
Chemicals
Glycolide and lactide were purchased from Purac (Netherlands) and further purified by recrystallization from ethyl acetate twice. Stannous octoate (A.R.) was purchased from Sigma (Germany) and directly used without further purification. Poly(vinyl alcohol) (PVA), 87–90 mol% hydrolysed with an average Mw of 30
000–70
000, was purchased from Sigma-Aldrich. 4-Arm-poly(ethylene glycol) succinimidyl ester-10K (4-arm-PEG-NHS) was purchased from XIAMEN SINOPEG BIOTECH CO., LTD. Branched polyethyleneimine (b-PEI, MW 1200, 99%) was purchased from Alfa Aesar. Cell counting kit-8 was purchased from Beyotime Biotechnology. DMEM (Corning) with 4.5 g L−1 glucose, L-glutamine, without sodium pyruvate was purchased from Beijing BioDee Biotechnology Co. Ltd. Other compounds and solvents were purchased from Beijing Chemical Reagents Company, China.
Characterization
The morphologies of NPs and hydrogels were characterized using scanning electron microscopy (SEM, JSM-6700F, JEOL). NPs were mounted to aluminium stubs using double side sticky tape and coated with gold–palladium in argon gas using a sputter coater (E-1010; Hitachi Ltd; Tokyo, Japan). The samples were observed using SEM with an accelerating voltage of 5 kV and a working distance of 8 mm. The dried NPs were dispersed in distilled water and measured by dynamic light scattering (DLS, Nano ZS90, Malvern). The elements of NPs were confirmed by energy dispersive X-ray spectrum (EDX, S-4300) at 15 kV. The mechanical and rheological properties were measured by advanced rheometric expansion system (TA ARES-G2 rheometer) with a 8 mm parallel-plate geometry.
Synthesis and characterization of PLGA
PLGA was synthesized with a predetermined molar ratio of L-lactide and glycolide (nLA
:
nGA = 70
:
30) via ring-opening polymerization.41 Gel permeation chromatography (GPC) measurement was carried out on GPCmax VE-2001 (Viscotek). The molecule weight of the synthesized PLGA7030 was 85
000 with a polydispersity to be 1.22.
Preparation of PLGA NPs
In order to achieve a high yield and uniform size distribution nanoparticles, we used microfluidics and premix membrane emulsification method to prepare different sizes of NPs respectively. The 300 nm PLGA NPs were prepared in microfluidics (M110P-UL-CE, Newton, MA, USA) by O/W method. 0.1 g of PLGA was dissolved in 10 mL of dichloromethane with magnetic stirring overnight, and then the oil phase was mixed with an aqueous phase containing 0.25% (w/w) PVA by mechanically stirred for 30 min to form a primary emulsion. The emulsion was refluxed five times through the chamber under a high pressure of 20
000 psi. The obtained uniform emulsion was stirring overnight to solidify the NPs. The NPs were collected by centrifugation (Sigma 3K30, German, rotor 1976H) at 11
000 min−1 for 10 min and washed with deionized water three times then lyophilized to obtain dry powder. Using similar procedure of our previous work,42 530 nm and 1000 nm PLGA NPs were produced from a PME equipment with the pore sizes of glass (SPG) membranes to be 1 and 2.5 μm (FMEM-500M, National Engineering Research Centre for Biotechnology, Beijing).
Aminolysis of the PLGA NPs
To arm the NPs with more reactive groups and obtain a long enough chain to bridge the adjacent nanoparticles, we grafted b-PEI to the surface of NPs via aminolysis reaction. We dissolved 1 g of 300 nm NPs in 20 mL of alcohol/water (1
:
1, v/v) solution containing b-PEI (20 mM) and sonicated at the power of 20 W for 60 min, then rinsed with deionized water three times to remove free b-PEI. The acquired NPs were freeze-dried with a lyophilizer. The 530 and 1000 nm NPs were treated by b-PEI for 30 and 40 min as the same procedure.
Synthesis of hybrid hydrogels
The preparation of PLGA NP/PEG hybrid hydrogels was carried out in centrifuge tube by mixing aqueous solutions of 4-arm-PEG-NHS with a certain amount of PLGA NP suspensions. The reaction mixture was quickly mixed by a vortex mixer before being kept for hydrogel formation.
Equilibrium swelling ratio (ESR)
Swelling ratio of the hybrid hydrogels was measured gravimetrically. The experiments were performed by immersing hydrogels in a large excess of water at room temperature for certain hours until the weight reached the maximum. The ESR was calculated using the following equation: ESR = (Wwet − Wdry)/Wdry, where Wwet and Wdry were the weights of the swollen state and the dry state of hydrogels, respectively.
Rheological analysis
Linear viscoelastic properties of hybrid hydrogels were measured by using a TA ARES-G2 rheometer with a 8 mm parallel-plate geometry at room temperature. Oscillatory frequency sweep was performed to measure the storage and loss modulus (G′ and G′′), in a range of angular frequency between 10−1 and 102 rad s−1. The strain amplitude was kept at 0.5% to ensure the linearity. 200 μL of NP suspension and 4-arm-PEG-NHS in deionized water were mixed by a vortex for the rheological experiments.
Mechanical analysis
Compression stress–strain tests were performed on an ARES-G2 rheometer. The prepared cylindrical hydrogels with a diameter of 8 mm and a height of 3 mm were compressed at a rate of 0.3 mm min−1 until rupture. The measurement was performed for three times.
Cytotoxicity
CCK-8 assay was used for cytotoxicity study in this work. Briefly, the hybrid hydrogels were cut into 8 mm circular discs (diameter) and then sterilized by immersing them in 75% ethanol and followed by washing with 1× PBS three times. MC3T3-E1 cells were plated in 96-well cell culture plate at a density of 104 cells per well, and then incubated with the hybrid hydrogels in Dulbecco's modified Eagle medium (DMEM) containing 10% FBS under 5% CO2 at 37 °C for 48 h. Then the medium was replaced with 100 μL of fresh medium. Subsequently, 10 μL of CCK-8 was added to each well and homogeneously mixed, followed by incubation at 37 °C for 4 h in a CO2 incubator, finally, 100 μL of the solutions were put into a new 96-well plate. The optical density of each well at 450 nm was read by a microplate reader. The relative cell viability were concluded as means and standard deviations of the measured absorbance normalized to the absorbance of non-treated control cells in plain medium, all of the measured and non-treated absorbance needed to delete the absorbance of CCK-8 and the medium.
Cell adhesion study
The hybrid hydrogels were cut into a diameter of 8 mm and then placed in a 48-well culture plate. After UV irradiation for 4 h, the hybrid hydrogels were immersed in the culture medium for 24 h, and then 20 μL of suspension of MC3T3-E1 with a density of 1.5 × 106 cells per mL was seeded on the hydrogels. Finally, the cells were cultured for 2 h prior to addition of culture medium into the culture plate. After 48 h, the medium was extracted from the culture plate and the hybrid hydrogels were washed with 1× PBS three times. The cells were fixed with 2.5% glutaraldehyde at 4 °C overnight and then freeze-dried for SEM performance.
Conclusions
In the present study, we prepared PLGA NP crosslinked 4-arm-PEG hydrogels with adjustable porous structures, surface properties and mechanical properties. Various uniform PLGA NPs with sizes of 300, 530 and 1000 nm were produced by microfluidics and PME. The amino groups were covalently introduced onto the surfaces of PLGA NPs via aminolysis of ester groups in PLGA using b-PEI. The nitrogen contents could reach 11.7%, 9.4% and 9.7% for 300, 530 and 1000 nm NPs. Mixing amino-packed PLGA NPs and 4-arm-PEG-NHS facilely formed the hybrid hydrogels in situ through quickly crosslinking reaction between active esters in 4-arm-PEG-NHS and amino groups in PLGA NPs. The pore size of hydrogels could be adjusted from 10–20 μm to 20–40 μm by altering NP content and PEG concentration for 300 nm NPs, but increased to 100–200 μm if we changed the NP size to 530 nm. The maximal compressive strength of the NP53010-PEG5 could reach 0.37 MPa. The hybrid hydrogels almost had no cytotoxicity for MC3T3-E1 model cells, indicating their good biocompatibility. The results of cell adhesion demonstrated more cells were attached onto the surface of NP3005-PEG2.5 and NP3007.5-PEG2.5. The method may be further explored to fabricate various kinds of NP/polymer hybrid hydrogels for applications in tissue engineering.
Acknowledgements
We gratefully acknowledge MOST (2014CB932200 and 2014BAI11B04), “Young Thousand Talents Program”, and NSFC (21504096, 51573195, 21174147 and 21474115) for financial support.
Notes and references
- C. M. Murphy, M. G. Haugh and F. J. O'Brien, Biomaterials, 2010, 31, 461–466 CrossRef CAS PubMed.
- A. Fakhari and J. A. Subramony, J. Controlled Release, 2015, 220, 465–475 CrossRef CAS PubMed.
- R. Hou, L. Nie, G. Du, X. Xiong and J. Fu, Colloids Surf., B, 2015, 132, 146–154 CrossRef CAS PubMed.
- E. P. da Silva, M. R. Guilherme, F. P. Garcia, C. V. Nakamura, L. Cardozo-Filho, C. G. Alonso, A. F. Rubira and M. H. Kunita, RSC Adv., 2016, 6, 19060–19068 RSC.
- X. Dong, C. Wei, T. J. Liu and F. Lv, RSC Adv., 2015, 5, 96336–96344 RSC.
- N. Stachowiak, A. Bershteyn, E. Tzatzalos and D. J. Irvine, Adv. Mater., 2005, 17, 383–498 CrossRef.
- A. Sannino, P. A. Netti, M. Madaghiele, V. Coccoli, A. Luciani, A. Maffezzoli and L. Nicolais, J. Biomed. Mater. Res., Part A, 2006, 79, 229–236 CrossRef CAS PubMed.
- A. Galperin, T. J. Long and B. D. Ratner, Biomacromolecules, 2010, 11, 2583–2592 CrossRef CAS PubMed.
- W. Teng, T. J. Long, Q. Zhang, K. Yao, T. T. Shen and B. D. Ratner, Biomaterials, 2014, 35, 8916–8926 CrossRef PubMed.
- B. M. Baker, B. Trappmann, W. Y. Wang, M. S. Sakar, I. L. Kim, V. B. Shenoy, J. A. Burdick and C. S. Chen, Nat. Mater., 2015, 14, 1262–1268 CrossRef CAS PubMed.
- S. Li, X. Wang, B. Cao, K. Ye, Z. Li and J. Ding, Nano Lett., 2015, 15, 7755–7765 CrossRef CAS PubMed.
- G. Huang, J. Gao, Z. Hu, J. V. St. John, B. C. Ponder and D. Moro, J. Controlled Release, 2004, 94, 303–311 CrossRef CAS PubMed.
- F. J. O'Brien, B. A. Harley, I. V. Yannas and L. J. Gibson, Biomaterials, 2005, 26, 433–441 CrossRef PubMed.
- V. Patrulea, N. Hirt-Burri, A. Jeannerat, L. A. Applegate, V. Ostafe, O. Jordan and G. Borchard, Carbohydr. Polym., 2016, 142, 114–123 CrossRef CAS PubMed.
- R. Suntornnond, J. An and C. K. Chua, Mater. Lett., 2016, 171, 293–296 CrossRef CAS.
- X. Zhu, S. Guo, T. He, S. Jiang, J. Dominik and G. Julius Vancso, Langmuir, 2016, 32, 1338–1346 CrossRef CAS PubMed.
- J. Kim, Y. Kong, S. M. Niedzielski, R. K. Singh, A. J. Putnam and A. Shikanov, Soft Matter, 2016, 12, 2076–2085 RSC.
- M. K. Jaiswal, J. R. Xavier, J. K. Carrow, P. Desai, D. Alge and A. K. Gaharwar, ACS Nano, 2016, 10, 246–256 CrossRef CAS PubMed.
- Q. Li, D. G. Barrett, P. B. Messersmith and N. Holten-Andersen, ACS Nano, 2016, 10, 1317–1324 CrossRef CAS PubMed.
- Y. Hashimoto, S. A. Mukai, S. Sawada, Y. Sasaki and K. Akiyoshi, Biomaterials, 2015, 37, 107–115 CrossRef CAS PubMed.
- J. C. Stanwick, M. D. Baumann and M. S. Shoichet, J. Controlled Release, 2012, 160, 666–675 CrossRef CAS PubMed.
- C. G. Y. B. Zhu, X. Y. Liu and J. C. Shen, Biomacromolecules, 2002, 3, 1312–1319 CrossRef PubMed.
- Y. Wan, J. Yang, J. Yang, J. Bei and S. Wang, Biomaterials, 2003, 24, 3757–3764 CrossRef CAS PubMed.
- Y. Wan, X. Qu, J. Lu, C. Zhu, L. Wan, J. Yang, J. Bei and S. Wang, Biomaterials, 2004, 25, 4777–4783 CrossRef CAS PubMed.
- Y. W. Awodi, A. Johnson, R. H. Peters and A. V. Popoola, J. Appl. Polym. Sci., 1987, 33, 2503–2512 CrossRef CAS.
- Z. H. Wei and Z. Y. Gu, J. Appl. Polym. Sci., 2001, 81, 1467–1473 CrossRef CAS.
- T. I. Croll, G. W. Stevens and J. J. Cooper-White, Biomacromolecules, 2004, 5, 463–473 CrossRef CAS PubMed.
- L. K. Limbach, Y. Li, R. N. Grass, T. J. Brunner, M. A. Hintermann, M. Muller, D. Gunther and W. J. Stark, Environ. Sci. Technol., 2005, 39, 9370–9376 CrossRef CAS PubMed.
- M. O. Andersen, A. Lichawska, A. Arpanaei, S. M. Rask Jensen, H. Kaur, D. Oupicky, F. Besenbacher, P. Kingshott, J. Kjems and K. A. Howard, Biomaterials, 2010, 31, 5671–5677 CrossRef CAS PubMed.
- K. Yu, S. Wu and H. Li, J. Exp. Nanosci., 2016, 11, 81–96 CrossRef CAS.
- E. A. Appel, M. W. Tibbitt, M. J. Webber, B. A. Mattix, O. Veiseh and R. Langer, Nat. Commun., 2015, 6, 6295 CrossRef CAS PubMed.
- L. Li, N. Wang, X. Jin, R. Deng, S. Nie, L. Sun, Q. Wu, Y. Wei and C. Gong, Biomaterials, 2014, 35, 3903–3917 CrossRef CAS PubMed.
- C. Garcia-Astrain, I. Ahmed, D. Kendziora, O. Guaresti, A. Eceiza, L. Fruk, M. A. Corcuera and A. N. Gabilondo, RSC Adv., 2015, 5, 50268–50277 RSC.
- W. Wei, X. Hu, X. Qi, H. Yu, Y. Liu, J. Li, J. Zhang and W. Dong, Colloids Surf., B, 2015, 125, 1–11 CrossRef CAS PubMed.
- B. Balakrishnan, N. Joshi, A. Jayakrishnan and R. Banerjee, Acta Biomater., 2014, 10, 3650–3663 CrossRef CAS PubMed.
- B. D. Boyan, T. W. Hummert, D. D. Dean and Z. Schwartz, Biomaterials, 1996, 17, 137–146 CrossRef CAS PubMed.
- B. A. C. Harley, H.-D. Kim, M. H. Zaman, I. V. Yannas, D. A. Lauffenburger and L. J. Gibson, Biophys. J., 2008, 95, 4013–4024 CrossRef CAS PubMed.
- Y. Hou, C. A. Schoener, K. R. Regan, D. Munoz-Pinto, M. S. Hahn and M. A. Grunlan, Biomacromolecules, 2010, 11, 648–656 CrossRef CAS PubMed.
- C. J. Wilson, R. E. Clegg, D. I. Leavesley and M. J. Pearcy, Tissue Eng., 2005, 11, 1–18 CrossRef CAS PubMed.
- R. Michael, V. Dorinel and S. Stefan, Adv. Colloid Interface Sci., 2011, 162, 87–106 CrossRef PubMed.
- S. Xu, F. Yang, X. Zhou, Y. Zhuang, B. Liu, Y. Mu, X. Wang, H. Shen, G. Zhi and D. Wu, ACS Appl. Mater. Interfaces, 2015, 7, 20460–20468 CAS.
- B. Liu, X. Zhou, F. Yang, H. Shen, S. Wang, B. Zhang, G. Zhi and D. Wu, Polym. Chem., 2014, 5, 1693–1701 RSC.
|
This journal is © The Royal Society of Chemistry 2016 |
Click here to see how this site uses Cookies. View our privacy policy here.