DOI:
10.1039/C6RA08157F
(Paper)
RSC Adv., 2016,
6, 65222-65231
Purification, properties and application of a collagenolytic protease produced by Pseudomonas sp. SUK
Received
30th March 2016
, Accepted 27th June 2016
First published on 4th July 2016
Abstract
The extracellular collagenolytic protease produced by Pseudomonas sp. SUK was purified to homogenecity by ammonium sulfate precipitation followed by DEAE-cellulose anion exchange chromatography. The enzyme was purified by 15.40 fold with 26.80% recovery and its molecular mass was found to be 58.6 kDa by SDS-PAGE. The optimum temperature and pH for the enzyme were 60 °C and 8.0, respectively. The purified enzyme was stable over a wide pH and temperature range and it was able to degrade various types of collagen. The Km and Vmax of the enzyme was 1.05 ± 0.09 mg ml−1 and 6.03 ± 0.52 × 10−4 mol l−1 min−1, respectively. EDTA, Fe3+, Hg2+, SDS, methanol and iso-propyl alcohol inhibited >25% enzyme activity whereas Zn2+, Ba2+, Ca2+, Tween 80, toluene and n-hexane were found to be good enhancers. Biophysical characterization revealed that the enzyme is 68.4% α-helix and 8.32% β-sheet, with hydrodynamic radius of approximately 3.1 nm. Furthermore the enzyme has a negative charge at pH 7.5 with a zeta potential value of −28.7 mV and Tm 62.3 °C ± 0.02 °C. This study assumes that the collagenolytic protease purified from Pseudomonas sp. SUK could be potentially exploited for meat tenderization at reduced temperatures as well as in animal tissue cultures as a tissue dissociating and cell dislodging agent.
1. Introduction
Protease represents one of the most important groups of enzyme with wide industrial applications, which accounts for approximately 60% of the total enzyme sales in the world.1 Proteases from complex eukaryotic systems like plants and animals are very costly to produce and they cannot meet the current demand. This attracted scientist and researcher interest toward microbial protease production which is relatively simple and cost effective.2 Microbial proteases account for approximately 59% of the total enzyme used worldwide.3
Collagen is the structural protein which is most abundant in the human body. Collagens are made up of three helically wound polypeptide fibrils and are approximately 30% of total proteins in mammalian body. Due to its structural complexity, collagen is very rigid in nature and can be cleaved by a limited number of proteases.4 Collagenolytic proteases are the enzymes having ability to hydrolyze the native and denatured triple helix of collagen to smaller fragments and hence they have wide application in pharmaceutical and food industries.5
In recent years collagenolytic protease has been exploited for various clinical as well as therapeutic applications like wound healing,6 preparation of intact mammalian cells,7 treatment of retained placenta,8 sciatica9 and various types of destructive fibrosis such as liver cirrhosis10 Dupuytren's contracture,11 Peyronie's disease.12 These proteases are also used as a hydrolyzing agent to obtain collagen or gelatin peptides with important biological activities like antihypertensive, anticancer and antioxidant.13 These peptides also have antimicrobial activity and are reported for their use in the suppression of collagen induced arthritis as well as osteoporosis.4 Food industry is another important sector where these proteases are extensively used. They have been used in production of protein hydrolysate as well as in meat tenderization.14,15
Many collagenolytic proteases have been reported from various microbial sources, such as Porphyromonas,16 Candida,17 Vibrio,18 Clostridium,19 Bacillus20 and Penicillium.21 Pseudomonas is a Gram-negative bacterium which is reported to have an array of proteolytic enzymes,22 but very little knowledge is known about collagenolytic protease from this species.
In our previous study, collagenolytic protease producing Pseudomonas sp. SUK was isolated and its production media was optimized with the help of RSM.23 Present work describes the purification, biochemical and biophysical characterization of collagenolytic protease from Pseudomonas sp. SUK. The meat tenderization ability of purified collagenolytic protease at lower temperature was evaluated. The application of enzyme in dissociation of animal tissues was also investigated in this study.
2. Materials and methods
2.1. Reagents
Collagen from calf skin, collagen from bovine achilles tendon, phenylmethanesulfonyl fluoride (PMSF), N-p-tosyl L-phenylalanine chloromethyl ketone (TPCK), pepstatin A, leupeptin, N-ethylmalemide (NEM), glutathione and 5,5-dithio-bis-(2-nitro benzoic acid) (DTNB) were purchased from Sigma (India); all other chemical reagents and organic solvents were of analytical grade and procured from Himedia (India) and local suppliers.
2.2. Microorganism and production of collagenolytic protease
Pseudomonas sp. SUK previously isolated in our lab was used for the production of enzyme. It was maintained on gelatin agar slants and stored at 4 °C. For the production of enzyme; previously statistically optimized medium was used containing gelatin 12.05 g l−1, peptone 12.26 g l−1, K2HPO4 1.29 g l−1, yeast extract 1 g l−1, MgSO4·7H2O 0.2 g l−1, CaCl2·2H2O 0.02 g l−1, MnCl2·4H2O 0.02 g l−1, ZnSO4·7H2O 0.02 g l−1, FeSO4·7H2O 0.02 g l−1 and pH 7.0.23
2.3. Determination of enzyme activity
Collagenolytic activity was measured as described Warinda et al.24 with little modification. The reaction mixture contained 0.3 ml of 0.2% (w/v) gelatin, 0.6 ml 100 mM Tris–HCl buffer, pH 7.5, containing 10 mM CaCl2 and 0.1 ml enzyme. The reaction mixture was incubated at 37 °C for 30 min and reaction was stopped by the addition of 0.5 ml of 10% trichloroacetic acid. The amount of released free amino acid was measured by the ninhydrin method using standard leucine curves. One unit activity of collagenolytic protease was expressed as 1 μmol of leucine equivalents released per minute per ml of enzyme.
2.4. Protein determination
The protein content was determined by Lowry method using bovine serum albumin (BSA) as a standard protein.25 During column chromatography, concentration of protein in the respective fraction is monitored by measuring their absorbance at 280 nm with a UV-vis spectrophotometer (Schimadzu UV1200).
2.5. Purification of enzyme
2.5.1. Crude enzyme preparation. The medium was inoculated with 1% fresh culture of Pseudomonas sp. SUK which was incubated at 37 °C for 36 hours and then centrifuged at 8000 rpm for 20 min. The enzyme from cell free supernatant was precipitated by adding solid ammonium sulphate to a final concentration of 60% saturation. The precipitate was harvested by centrifugation at 8000 rpm for 20 min and re-suspended in a minimal volume of 100 mM Tris–HCl buffer (pH 7.5) and dialyzed overnight against the same buffer (4 °C). The dialyzed sample was assayed for enzyme activity and protein content.
2.5.2. Ion exchange chromatography. Dialyzed enzyme was further purified by the DEAE-cellulose column chromatography. The column (1 × 15 cm) was equilibrated with 100 mM Tris–HCl buffer (pH 7.5) and washed with the same buffer after loading of crude enzyme. The enzyme was then eluted with 100–500 mM NaCl in 100 mM Tris–HCl buffer (pH 7.5) at a flow rate of 0.5 ml min−1 (5.0 ml per tube). All fractions were checked for enzyme activity and positive fractions were dialyzed overnight against 100 mM Tris–HCl (pH 7.5) buffer.
2.5.3. SDS-PAGE (sodium dodecyl sulfate-polyacrylamide gel electrophoresis). Purified enzyme in dye was boiled at 100 °C for 5 min and then subjected to SDS-PAGE analysis with 12% resolving and 5% stacking gel according to the method of Laemmli and gels were stained with Coomassie Brilliant Blue R-250.26 Prestained protein markers (medium range, Catalog no. 623112375001730, Genei™, Bangalore, India) having phosphorylase b (98 kDa), bovine serum albumin (68 kDa), ovalbumin (44 kDa), glutathione-S-transferase (29 kDa), and lysozyme (16 kDa) were used for molecular weight comparison.
2.6. Characterization of purified collagenolytic protease
2.6.1. Biochemical characterization.
2.6.1.1. Effect of pH. The optimum pH for the enzyme was determined by testing its activity at a range of pH using 100 mM citrate buffer, pH 4.0–6.0; 100 mM sodium phosphate buffer, pH 7.0; 100 mM Tris–HCl buffer, pH 7.5–9.0; glycine NaOH buffer, pH 10.0–11.0 at 37 °C. The pH stability was determined after incubating the enzyme at 25 °C for 1 h at the pH range of 4.0–11.0 and residual activity of the enzyme was determined at pH 8.0 using standard assay conditions.
2.6.1.2. Effect of temperature. Optimum temperature for enzyme was examined at various temperatures (4–80 °C) in 100 mM Tris–HCl buffer (pH 8.0) under standard assay conditions. To determine temperature stability, enzyme was initially pre-incubated at various temperatures (4–80 °C) for 1 h without the substrate and residual enzyme activity was measured at pH 8.0 and 60 °C using standard assay conditions.
2.6.1.3. Substrate specificity and kinetic parameters. The substrate specificity study of the enzyme was carried out using three substrates, such as acid soluble collagen (ASC), bovine achilles tendon collagen (BATC) and gelatin. The standard reaction mixture, containing 0.3 ml of 1 mg ml−1 substrate in 0.6 ml 100 mM Tris–HCl buffer (pH 8.0) including 10 mM CaCl2 was incubated with 0.1 ml enzyme at 60 °C for 30 min. The reaction was stopped by the addition of 0.5 ml of 10% trichloroacetic acid; the amount of free amino groups and subsequently enzyme activity was measured as per standard assay conditions.The Michaelis–Menten constant (Km) of purified enzyme was calculated from Lineweaver–Burk plot with 100 mM Tris–HCl buffer (pH 8.0) including 10 mM CaCl2 at 60 °C with acid soluble collagen as the substrate. The range of the substrate concentration used to calculate Km and Vmax was 0.1–1 mg ml−1. All data obtained from the enzyme kinetic assays and plotting were processed using Microsoft Office Excel.
2.6.1.4. Effect of different inhibitors, metal ions and surfactants on the enzyme. The effects of inhibitors like phenylmethanesulfonyl fluoride (PMSF), N-p-tosyl L-phenylalanine chloromethyl ketone (TPCK), pepstatin A, leupeptin, N-ethylmalemide (NEM), ethylene-diaminetetraacetic acid (EDTA), glutathione, 5,5-dithio-bis-(2-nitro benzoic acid) (DTNB); various metal ions like NaCl, KCl, MnCl2, BaCl2, MgCl2, CaCl2, FeCl3, ZnCl2, HgCl2, PbCl2 and surfactants like Tween 80 and sodium dodecyl sulfate on enzyme stability were investigated by pre-incubating the enzyme at 25 °C for 30 min. Enzyme assays were carried out at pH 8.0 and 60 °C using standard assay conditions. The activity of enzyme without any inhibitor, metal ion and surfactant was taken as 100%.
2.6.1.5. Effect of organic solvents on the enzyme. The organic solvent stability of the enzyme was studied by incubating the enzyme with organic solvents 25% (v/v) at 25 °C for 1 day and 30 days with shaking (150 rpm). Various solvents like methanol, iso propyl alcohol, n-propanol, toluene and n-hexane with different log
P values were used in this study and residual activity was calculated at pH 8.0 and 60 °C using standard assay conditions. The activity of enzyme without any organic solvent was taken as 100%.
2.6.2. Biophysical characterization.
2.6.2.1. Circular dichroism (CD) spectropolarimetry. The enzyme was subjected to circular dichroism (CD) spectropolarimeter analysis. All far-UV CD spectra were obtained at room temperature and recorded on a JASCO J-715 (Jasco Inc., Easton, MD, USA) spectropolarimeter using solutions of a protein concentration about 0.15–0.2 mg ml−1. All spectra resulted from averaging three scans and were corrected for the respective blanks. Results are expressed as molar ellipticity, [Θ] (deg cm2 dmol−1), based on a mean amino acid residue weight (MRW). The molar ellipticity was determined as [Θ]λ = (Θ × 100 × MRW)/(cl), where l, is the light path length in centimeters, ‘c’ is the protein concentration in mg ml−1, and Θ is the measured ellipticity in degrees at the relevant wavelength. A collected CD spectrum was processed using K2D3 program and secondary structural features were elucidated.
2.6.2.2. Dynamic light scattering (DLS). Dynamic light scattering study was performed using Zeta Sizer Nano ZS (Malvern Instruments Ltd, Worcestershire, UK). The experimental set up was as follows: laser used was 633 nm, 4 mW linearly polarized; the light scattered by the sample Single Photon Counting Module (SPCM). The sample was prepared at concentration of 1 mg ml−1 in 100 mM Tris HCl buffer (pH 7.5) and the experiment was carried out at 25 °C.
2.6.2.3. Zeta potential. The zeta potential was measured using the laser Doppler velocimetry mode of a Zeta Sizer Nano ZS (Malvern Instruments Ltd, Worcestershire, UK). In the solution, fixed charge associated with any particle is always surrounded by counter ions. Therefore as positive counter ionic potential increases bound negative charge also increases. To a certain level of attraction the counter ions move with the particle during Brownian motion. These (known as Stern charges) constitute zeta potential. For moving particles in the measurement zone, shift in the frequency of scattered light is proportional to their velocity. Since the zeta potential is pH dependent, the measurements were performed in 100 mM Tris HCl buffer (pH 7.5) with enzyme at concentration of 1 mg ml−1. Each result was obtained from the average of repetition of 30 runs.
2.6.2.4. Differential scanning calorimetry (DSC). DSC was used to analyze thermal denaturation of enzyme. The sample with concentration of 0.5 mg ml−1 in 100 mM Tris HCl buffer (pH 7.5) were heated at a constant rate of 90 °C h−1 from 20° up to 100 °C on a VP-DSC differential scanning calorimeter (MicroCal VP-Capillary DSC System, GE Healthcare Bio-Sciences Corp., NJ, USA). Calorimetric curves were corrected for instrumental background as described earlier27 and transition temperature (Tm) was determined from the maximum of the thermal transition.
2.7. Meat tenderization
Defatted goat meat was cut into 2 cm × 2 cm × 2 cm cubes and incubated at 4 °C for 20 hours with 0.2 mg of enzyme as test. Meat cubes incubated at 4 °C for 20 hours in sterile distilled water without enzyme were used as control. The samples are analyzed with the help of TA-XT2 Texture Analyzer, Stable Micro System Ltd., London. Texture profile analysis was carried out using probe P/75 (75 mm compression platen), in compression mode at pretest speed 1.00 mm s−1, test speed 5.00 mm s−1, post-test speed 5.00 mm s−1. Target mode was kept at 40.00% strain and trigger force 5.0 g. Data acquisition rate was kept at 200.00 pps.28
2.8. MRC-5 and Vero cell dissociation study
MRC-5 and Vero cell lines were used in the present investigation. Three to seven days old cells were maintained in Eagle's Minimum Essential Medium (Eagle's MEM) containing 2 mM glutamine, 1.5 g l−1 sodium bicarbonate and 10% fetal bovine serum (FBS) in 25 cm2 tissue culture flask (TCF). Culture medium was removed and both the cell monolayers were rinsed with 0.2 mg of enzyme solution to remove all traces of serum. Cell monolayers were then incubated with 0.2 mg of enzyme solution at 37 °C for 2–5 minutes and were observed for rounding off of the cells. Then the cells were observed under inverted microscope for possible detachment of cells.
2.9. Statistical analysis
Results obtained were mean of three or more determinants. One way ANOVA was done using Microsoft Office data analysis tool pack. Difference of P ≤ 0.05 was considered as significant.
3. Results
3.1. Purification of enzyme
Pseudomonas sp. SUK was found to secrete extracellular collagenolytic protease which was purified from crude extract by ammonium sulphate precipitation and anion exchange chromatography using DEAE resin. Fraction number 12 and 13 eluted with 200 mM NaCl showed the presence of purified enzyme of 58.6 kDa, which was confirmed by SDS-PAGE analysis (Fig. 1). Both the purified fractions were pooled together which gave 26.80% yield with 15.40 fold purification (Table 1).
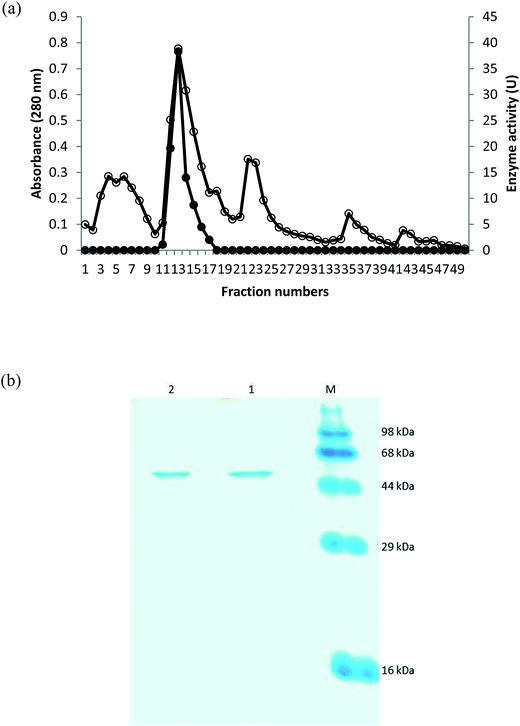 |
| Fig. 1 (a) Chromatography of the collagenolytic protease from Pseudomonas sp. SUK on DEAE-cellulose. Protein content at 280 nm (○) and collagenolytic protease activity (•). (b) SDS-PAGE of the purified collagenolytic protease. (M) Prestained protein markers. (1) Purified collagenolytic protease (13th elution). (2) Purified collagenolytic protease (12th elution). | |
Table 1 Flow sheet for collagenolytic protease purification
Purification step |
Total activity (unit) |
Total protein (mg) |
Specific activity (U mg−1) |
Yield |
Purification fold |
Crude extract |
1081.74 |
86.96 |
12.44 |
100 |
1 |
Ammonium sulfate |
605 |
11.77 |
51.40 |
55.93 |
4.13 |
DEAE cellulose |
289.8 |
1.51 |
191.48 |
26.80 |
15.40 |
3.1.1. Biochemical characterization. Effect of different pH ranging from 4 to 11 on relative and residual activity of purified enzyme was determined. Enzyme was found to be active over a broad pH range of 6 to 9 with 77.41 and 88.54% relative activity. Optimum activity was found to be at pH 8 with highest relative activity (Fig. 2a). Enzyme was found to be moderately stable at extreme pH values of 5 and 11 while high stability was found in the range of pH 6 to 10 with 94.21 and 82.93% residual activity (Fig. 2b).
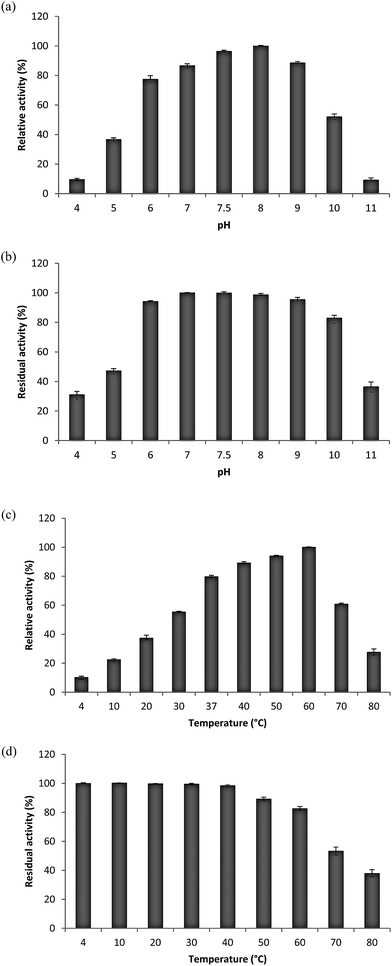 |
| Fig. 2 Effect of pH on the activity (a) and stability (b) of collagenolytic protease. The activity of the enzyme at pH 8.0 for (a) and at 7.0 for (b) was taken as 100%. Effect of temperature on the activity (c) and stability (d) of collagenolytic protease. The activity of the enzyme (c) at 60 °C and (d) 4 °C was taken as 100%. Each point represents the mean (n = 3) ± SD. | |
Effect of different temperatures ranging from 4 °C to 80 °C on relative and residual activity of purified enzyme was determined. The optimum temperature of enzyme was found to be 60 °C but its activity was drastically reduced above it. Enzyme was found to be active even at 4 °C with 10.23% relative activity (Fig. 2c). Stability studies inferred that the enzyme was stable over wide range of temperatures but its activity was reduced linearly from 50 °C and above (Fig. 2d).
The substrate specificity of enzyme was determined using ASC, BATC and gelatin which showed very high activity towards gelatin followed by ASC and BATC (Table 2). Kinetic study of enzyme was performed using ASC as a substrate. The Km and Vmax values were calculated using Lineweaver–Burk plot which were 1.05 ± 0.09 mg ml−1 and 6.03 ± 0.52 × 10−4 mol l−1 min−1 respectively.
Table 2 Substrate specificitya
Values represents mean of three replicates, and ±standard errors are reported. |
Substrate |
Gelatin |
BATC |
ASC |
% activity |
100 ± 1.1 |
51.65 ± 2.9 |
67.10 ± 2.4 |
In the inhibition study, enzyme was not affected by the most inhibitors tested. However, 80.98% of enzyme activity was inhibited by EDTA which is well-known inhibitor of metalloproteases. Of the several metal ions assayed for their effects on collagenolytic protease, only Fe3+ and Hg2+ were found to have significant inhibitory effect with 63.78 and 59.65% enzyme activity inhibition, respectively. While it was enhanced up to 106.53, 114.96 and 125.92% with addition of Zn2+, Ba2+ and Ca2+ respectively. The nonionic surfactants Tween 80 exhibited slight positive effect enhancing the enzyme activity up to 103.55% whereas anionic surfactant SDS inhibited enzyme activity by 28.39% (Table 3).
Table 3 Effects of various inhibitors and metal ions on collagenolytic protease stability. Collagenolytic protease activity measured in the absence of any inhibitor, metal ion or surfactant was taken as control (100%). Residual activity was measured at pH 8.0 at 60 °Ca
Inhibitor/metal ions |
Concentration |
Residual activity (%) |
Values represents mean of three replicates, and ±standard errors are reported. |
Control |
— |
100 ± 1.3 |
PMSF |
5 mM |
99.77 ± 1.2 |
TPCK |
1 mM |
98.50 ± 1.8 |
Pepstatin A |
1 mM |
86.92 ± 2.4 |
Leupeptin |
50 μg ml−1 |
94.95 ± 2.4 |
N-Ethylmalemide |
2 mM |
97.18 ± 1.4 |
EDTA |
10 mM |
19.02 ± 2.3 |
Glutathione |
5 mM |
88.70 ± 2.3 |
DTNB |
5 mM |
94.95 ± 1.2 |
Na |
5 mM |
99.54 ± 1.7 |
K |
5 mM |
98.68 ± 2.7 |
Mn |
5 mM |
90.93 ± 1.6 |
Ba |
5 mM |
114.96 ± 2.0 |
Mg |
5 mM |
91.97 ± 2.3 |
Ca |
5 mM |
125.92 ± 0.9 |
Fe |
5 mM |
36.22 ± 1.8 |
Zn |
5 mM |
106.53 ± 2.5 |
Hg |
5 mM |
40.35 ± 3.5 |
Pb |
5 mM |
68.74 ± 2.1 |
Tween 80 |
0.1% |
103.55 ± 1.03 |
SDS |
0.1% |
71.61 ± 1.77 |
Various organic solvents were examined for their effects on the stability of enzyme. Methanol, iso-propyl alcohol and n-propanol were moderately lowered the enzyme activity by 46.41, 31.95 and 16.48%, respectively. Whereas, in the presence of hydrophobic organic solvents like toluene and n-hexane (with log
P values ≥ 2.0), enzyme showed remarkable stability over the period of month (Table 4).
Table 4 Effects of organic solvents on the stability of the purified collagenolytic protease. The non-treated enzyme was considered as 100%a
Organic solvent (50%, v/v) |
log P |
Residual activity (%) |
After 1 day |
After 30 days |
Values represents mean of three replicates, and ±standard errors are reported. |
Control |
— |
100 ± 1.4 |
100 ± 1.4 |
Methanol |
−0.76 |
70.29 ± 2.9 |
53.59 ± 1.8 |
Iso-propyl alcohol |
0.05 |
77.13 ± 1.0 |
68.05 ± 0.6 |
n-Propanol |
0.28 |
91.59 ± 1.6 |
83.52 ± 1.4 |
Toluene |
2.5 |
125.99 ± 2.9 |
103.01 ± 1.8 |
n-Hexane |
3.5 |
158.04 ± 6.3 |
133.76 ± 2.4 |
3.1.2. Biophysical characterization. Circular dichroism spectropolarimetry was used to elucidate the secondary structural features of the purified enzyme. The far UV region of enzyme shows two minimum values for molar ellipticity at about 210 and 220 nm while maximum value molar ellipticity can be seen at 195 nm (Fig. 3). The K2D3 analysis suggested that, the enzyme has 68.4% α-helix and 8.32% β-sheet.
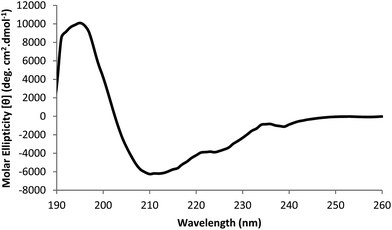 |
| Fig. 3 Far-UV CD spectra of native collagenolytic protease. The CD data were expressed as molar ellipticity (deg cm2 dmol−1). | |
In dynamic light scattering studies, the cumulant fit curve for autocorrelation function indicates smooth decay up to 100 μs (Fig. 4). The translational diffusion coefficient determined from this curve indicated that the purified enzyme has hydrodynamic radius approximately 3.1 nm. Based on Henry's zeta potential model it was −28.7 mV. The results indicated that at pH 7.5, enzyme was negatively charged.
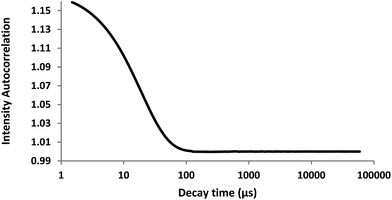 |
| Fig. 4 DLS analysis of purified collagenolytic protease. | |
Thermal stability of the enzyme was assessed by differential scanning calorimetry. The stability was determined as a midpoint temperature (Tm) of the thermal melting curve at which the molar heat capacity peaked. A single thermal transition was observed at Tm 62.3 °C ± 0.02 °C (Fig. 5).
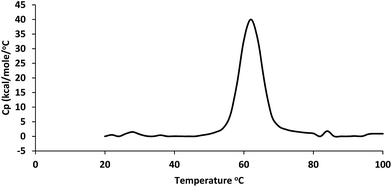 |
| Fig. 5 Study of thermal stability of collagenolytic protease by Differential Scanning Calorimetry (DSC). | |
3.1.3. Application of purified collagenolytic protease. In meat tenderization studies goat meat treated with enzyme and without enzyme were analyzed by texture profile analyzer and results are shown in Table 5. As compared to control hardness, gumminess and chewiness of the test samples were significantly decreased; whereas springiness, cohesiveness and adhesiveness were moderately decreased (Fig. 6a).
Table 5 Texture profile analysis of meata
Sample |
Hardness (gf) |
Springiness |
Cohesiveness |
Gumminess |
Chewiness |
Adhesiveness (gf) |
Values represents mean of three replicates, and ±standard errors are reported. |
Control |
3529 ± 428 |
0.94 ± 0.05 |
0.61 ± 0.04 |
2162 ± 390 |
2055 ± 483 |
−23 ± −3.3 |
Purified enzyme |
1255 ± 112 |
0.91 ± 0.001 |
0.52 ± 0.04 |
652 ± 108 |
595 ± 99 |
−25.9 ± −2.9 |
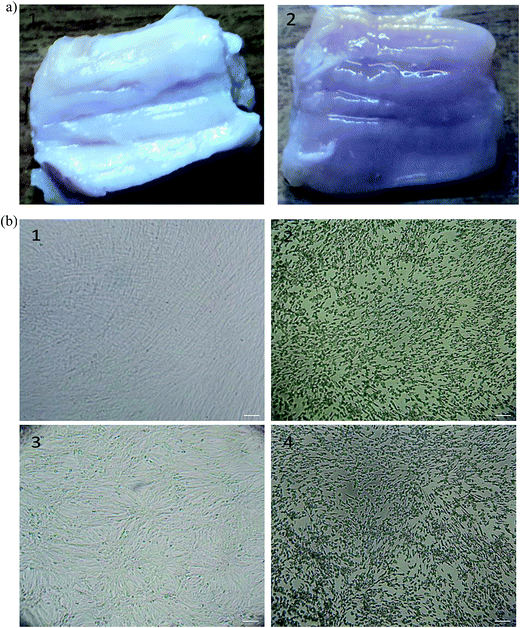 |
| Fig. 6 The appearance of meat treated with enzyme (a). Meat dipped in distilled water was used as control (1) purified collagenolytic protease (2). Animal tissue dissociation assay (b). MRC-5 cells before collagenolytic protease treatment (1), after treatment (2), Vero cells before collagenolytic protease treatment (3), after treatment (4). Scale bar indicates 100 μm. | |
Whereas in animal tissue culture studies, MRC-5 and Vero cell lines were treated with enzyme and then observed under inverted microscope. In both the cell lines, cells were found to be separated from the substratum (Fig. 6b).
4. Discussion
Till date very few collagenolytic proteases have been reported. This study describes the purification and characterization of collagenolytic protease from Pseudomonas sp. SUK. The strain SUK was grown in an optimized media and enzyme was purified from its culture supernatant. Enzyme was purified by ammonium sulphate precipitation followed by DEAE cellulose chromatography. Two fractions (12th and 13th) showed purified enzyme with 15.40 fold and 26.80% yield. In contrast, protease from Pseudomonas thermaerum with similar protocol only had 9.3% recovery with 6.08 purification fold.29 SDS-PAGE analysis revealed that the strain SUK enzyme has molecular weight of 58.6 kDa which was nearly equal to collagenolytic protease from Bacillus pumilus Col-J with molecular weight 58.64 kDa.20 On the other hand it was very close to protease produced by Pseudomonas aeruginosa MCM B-327 having molecular weight of 56 kDa but contradictorily this protease was reported inactive against collagen.30
Most collagenolytic protease exhibited optimum pH values in the range of 7.0 to 8.0 (ref. 20) in contrast to this strain SUK enzyme was active in the range of 6.0 to 9.0 pH. Its pH optima was found to be 8.0, which is near about similar to the majority of the collagenolytic protease from different bacterial sources. Bacillus cereus MBL 13 showed optimum pH as 8.0 while Streptomyces exfoliatus CFS 1068 showed optimum at pH 7.0.31,32 Strain SUK enzyme was found to be stable in the wide pH range of 6.0 to 10.0 whereas collagenolytic protease from Bacillus pumilus Col-J and Bacillus cereus MBL 13 were having stability in the range of 6.5 to 8.5 pH.20,31 This characteristic feature of strain SUK enzyme opens an avenue for its use in various industries.
The optimum temperature of strain SUK enzyme was found to be at 60 °C which was greater than collagenolytic protease from Bacillus pumilus Col-J having optima at 45 °C and lower than collagenase from Streptomyces exfoliatus CFS 1068 having optima at 70 °C.20,32 The versatility of strain SUK enzyme was its activity even at 4 °C which can be exploited potently for tenderization of meat at refrigeration temperature. Collagenolytic protease from Bacillus cereus MBL 13 and protease from Pseudomonas putida SKG-1 showed about 60% and 38% relative activity at 10 °C, respectively.31,33 Protease from Pseudoalteromonas sp. SM9913 was also having nearly 12% relative activity at 4 °C.34 In case of thermal stability, strain SUK enzyme was stable up to 40 °C and lost only 17% of its activity at 60 °C in one hour of incubation. Comparatively protease from Pseudomonas aeruginosa A2 was also stable up to 40 °C but lost around 40% of its activity at 60 °C in one hour of incubation.22
The strain SUK enzyme was found to have high activity towards gelatin followed by ASC and least activity was found in the case of BATC. Km of strain SUK enzyme was found to be 1.05 ± 0.09 mg ml−1 which was higher than the collagenolytic proteases from Bacillus licheniformis F 11.4 (0.26 mg ml−1) and Bacillus pumilus Col-J (0.79 mg ml−1).20,35 But it was lower than the collagenolytic protease from Bacillus cereus MBL13 (1.31 mg ml−1) and protease from haloalkaliphilic Bacillus sp. (2 mg ml−1) and Pseudomonas aeruginosa PseA (2.69 mg ml−1).31,36,37 Lower Km value of the strain SUK enzyme suggests its higher affinity and efficiency towards collagen substrate.
Classification of protease can also be done on the basis of their sensitivity to various inhibitors. Pepstatin which is a potent inhibitor of nearly all acid proteases38 and glutathione which is a potent sulfhydryl reagent39 partly inhibited strain SUK enzyme. A metal cofactor appears to be required for activity, since EDTA inhibited the enzyme activity.40 Elastase from Pseudomonas aeruginosa CTM50182 coincides with these results where it was highly inhibited by EDTA and partially by glutathione but not by pepstatin A.39 Strain SUK enzyme was highly inhibited by Fe3+ and Hg2+ whereas activity was enhanced after addition of the Zn2+, Ba2+ and Ca2+. These results are corroborated by Pseudomonas aeruginosa strain K protease where Zn2+, Ba2+ and Ca2+ found to enhance the activity whereas Fe3+ completely inhibited the activity.41 Strain SUK enzyme was found to have activity even in the presence of heavy metals as well as surfactants like Hg2+, Pb2+, Tween 80 and SDS; similar reports have been reported by protease from Pseudomonas putida SKG-1.33
Amongst the various organic solvents tested, strain SUK enzyme stability was found to be reduced in methanol, iso-propyl alcohol and n-propanol whereas it was increased in the presence of toluene and n-hexane. Proteases which are naturally stable in the presence of organic solvents are very useful for synthetic reactions.41 Stability of biocatalyst is highly dependent on polarity of the solvent system which is often measured in “log
Po/w” value; Po/w is the measure of partition coefficient of the solvent in the octanol–water two phase system. Solvents with high log
Po/w values causes less inactivation of biocatalysts than solvents with low Po/w values.42 Similarly increasing log
Po/w values of the solvents were found to stabilize the activity of strain SUK enzyme. Parallel reports were reported by Pseudomonas aeruginosa CTM50182 elastase and Pseudomonas aeruginosa strain K protease.39,41
Circular dichroism spectropolarimetric analysis revealed that all-α proteins of strain SUK enzyme shows an intense negative band with two peaks (at 210 and 220 nm) and a strong positive band (at 191–195 nm). α-Helix which is often the major stabilizing conformation in proteins found to be dominating in case of strain SUK enzyme. Similar results were reported in case of collagenase from Achromobactor iophagus and Clostridium histolyticum.43 Dynamic light scattering studies revealed that the suspension contained colloidal particles of single size. Hence, suspension was monomodal monodisperse having purified collagenolytic protease with hydrodynamic radius approximately 3.1 nm. Zeta potential studies indicated that strain SUK enzyme with zeta potential of −28.7 mV was negatively charged indicating good colloidal stability at pH 7.5.44 Thermal stability of the purified collagenolytic protease was determined as a midpoint temperature (Tm) 62.3 °C ± 0.02 °C. It can be corroborated by the residual activity of the strain SUK enzyme which was lowered up to 50% at 70 °C.
The texture profile studies revealed that test meat samples were found to be tenderized effectively by the strain SUK enzyme. So this could be a pragmatic solution where meat can be tenderized at refrigeration temperature; lowering the chances of microbial contamination. Collagenolytic protease from Pseudoalteromonas sp. SM9913 was also studied for its meat tenderization ability at reduced temperature and was found to tenderize meat effectively.15 In another application, strain SUK enzyme was found to dissociate the animal tissues very efficiently. Trypsin which is widely used for dissociation of animal tissues actually acts in a narrow pH range (7.2–7.4) while strain SUK enzyme is active in broad pH range which overcomes the limitations of trypsin.45 Hence it can be effectively used in animal tissue culture as a cell dislodging agent.
Bacterial collagenolytic proteases include some metalloproteases from M9 family and serine proteases distributed in the S1, S8, and S53 families. In addition, some members of the U32 family, mainly from pathogenic bacteria, are also reported to be collagenolytic proteases. M9 family members Clostridium and Vibrio collagenases contains a conserved zinc-binding motif either (HEY/FTH) or (HEYT/VH) which functions as a catalytic domain.46 The S1 family has His–Asp–Ser as their catalytic residues whereas S8 family is characterized by an Asp–His–Ser catalytic triad.47 Members of the S53 family have a unique catalytic triad as Glu–Asp–Ser.48 The U32 family contains several collagenolytic proteases of unknown catalytic type.49
Protein database search for collagenase from Pseudomonas sp. applying filter of molecular weight cutoff between 58
400 and 58
800 Da, yielded two entries with accession no. WP_026000506 and WP_017699480. Both sequences showed none of the active site motif belonging to M9, S1, S8 and S53 families. According to Conserved Domain Database (CDD) these sequences (WP_026000506 and WP_017699480) showed relation with U32 protease superfamily. Additionally, activity of our collagenolytic protease investigated in the study was enhanced by Ca2+ and inhibited by EDTA similar to previously reported PrtC from U32 protease superfamily.50 From these clues we believe that purified collagenolytic protease under investigation belongs to U32 protease family. Unfortunately, because of unavailability of structural information, the protein fold and active site residues of the catalytic domain for any member of this family are unclear.51
5. Conclusion
Considering the characteristics of extracellular collagenolytic protease produced by Pseudomonas sp. SUK, such as ability to hydrolyze various collagen sources, broad pH, temperature activity and stability as well as solvent stability; opens an avenue for its use in various industries. Pseudomonas sp. SUK could be a potential candidate for industrial production of collagenolytic protease as its simple purification steps lowers the cost of purification. This is a valuable enzyme source which can act on meat at reduced temperatures as well as dissociate animal tissues very effectively and hence it can be potentially exploited by food as well as pharma industries.
Acknowledgements
Prashant K. Bhagwat is thankful to UGC for awarding BSR meritorious Fellowship for doctoral research. Sowmya B. Jhample, one of the authors is thankful to UGC-RGNF for awarding Junior Research Fellowship. Corresponding author wish to thank UGC-MRP with sanction grant No. F. No. 41-1282/2012 (SR).
References
- M. B. Rao, A. M. Tanksale, M. S. Ghatge and V. V. Deshpande, Microbiol. Mol. Biol. Rev., 1998, 62(3), 597–635 CAS.
- S. B. Jhample, P. K. Bhagwat and P. B. Dandge, Biocatal. Agric. Biotechnol., 2015, 4(3), 370–379 Search PubMed.
- W. H. Chu, J. Ind. Microbiol. Biotechnol., 2007, 34(3), 241–245 CrossRef CAS PubMed.
- K. Watanabe, Appl. Microbiol. Biotechnol., 2004, 63(5), 520–526 CrossRef CAS PubMed.
- H. S. Hamdy, Indian J. Biotechnol., 2008, 7(3), 333–340 CAS.
- O. Erdeve, B. Atasay and S. Arsan, Pediatr. Dermatol., 2007, 24, 195–196 CrossRef PubMed.
- M. Kim, S. E. Hamilton, L. W. Guddat and C. M. Overall, Biochim. Biophys. Acta, 2007, 1770, 1627–1635 CrossRef CAS PubMed.
- H. Eiler and F. M. Hopkins, J. Am. Vet. Med. Assoc., 1993, 203, 436–443 CAS.
- K. H. Chu, Clin. Orthop., 1987, 215, 99–104 Search PubMed.
- B. Jin, H. J. Alter, Z. C. Zhang, J. W. Shih, J. M. Esteban, T. Sun, Y. S. Yang, Q. Qiu, X. L. Liu, L. Yao, H. D. Wang and L. F. Cheng, Lab. Invest., 2005, 85, 992–1002 CrossRef CAS PubMed.
- C. C. Neal, C. S. Ramesh, J. S. Melissa and C. C. Kevin, HAND, 2011, 6, 250–255 CrossRef PubMed.
- H. J. Gerald, J. Sex. Med., 2008, 5, 180–187 Search PubMed.
- A. Aleman, E. Perez-Santin, S. Bordenave-Juchereau, I. Arnaudin, M. C. Gomez-Guillen and P. Montero, Food Res. Int., 2011, 44(4), 1044–1051 CrossRef CAS.
- R. Gupta, Q. Beg and P. Lorenz, Appl. Microbiol. Biotechnol., 2002, 59(1), 15–32 CrossRef CAS PubMed.
- G. Y. Zhao, M. Y. Zhou, H. L. Zhao, X. L. Chen, B. B. Xie, X. Y. Zhang and Y. Z. Zhang, Food Chem., 2012, 134(4), 1738–1744 CrossRef CAS PubMed.
- M. A. Houle, D. Grenier, P. Plamondon and K. Nakayama, FEMS Microbiol. Lett., 2003, 221(2), 181–185 CrossRef CAS PubMed.
- C. A. Lima, P. M. Rodrigues, T. S. Porto, D. A. Viana, J. L. Lima Filho, A. L. Porto and M. G. Carneiro-da-Cunha, Biochem. Eng. J., 2009, 43(3), 315–320 CrossRef CAS.
- N. Teramura, K. Tanaka, K. Iijima, O. Hayashida, K. Suzuki, S. Hattori and S. Irie, J. Bacteriol., 2011, 193(12), 3049–3056 CrossRef CAS PubMed.
- M. K. Gelbard, L. Chagan and J. P. Tursi, J. sex. Med., 2015, 12(6), 1481–1489 CrossRef CAS PubMed.
- Q. Wu, C. Li, C. Li, H. Chen and L. Shuliang, Appl. Biochem. Biotechnol., 2010, 160(1), 129–139 CrossRef CAS PubMed.
- C. A. Lima, A. C. F. Junior, J. L. Lima Filho, A. Converti, D. A. V. Marques, M. G. Carneiro-da-Cunha and A. L. F. Porto, Biochem. Eng. J., 2013, 75, 64–71 CrossRef CAS.
- O. Ghorbel-Bellaaj, B. K. Hayet, A. Bayoudh, I. Younes, N. Hmidet, K. Jellouli and M. Nasri, Int. J. Biol. Macromol., 2012, 50(3), 679–686 CrossRef CAS PubMed.
- P. K. Bhagwat, S. B. Jhample and P. B. Dandge, Microbiology, 2015, 84(4), 520–530 CAS.
- S. Warinda, B. Cheirsilp and A. Jongjareonrak, New Biotechnol., 2011, 28, 649–655 CrossRef PubMed.
- O. H. Lowry, N. J. Rosebrough, A. L. Farr and R. J. Randall, J. Biol. Chem., 1951, 193(1), 265–275 CAS.
- U. K. Laemmli, Nature, 1970, 227(5259), 680–685 CrossRef CAS PubMed.
- E. Kremneva, O. Nikolaeva, R. Maytum, A. M. Arutyunyan, S. Y. Kleimenov, M. A. Geeves and D. I. Levitsky, FEBS J., 2006, 273(3), 588–600 CrossRef CAS PubMed.
- A. A. Gatade and A. K. Sahoo, J. Food Sci. Technol., 2015, 52(12), 8395–8402 CrossRef CAS PubMed.
- S. Gaur, S. Agrahari and N. Wadhwa, Open Microbiol. J., 2010, 4, 67 CrossRef CAS PubMed.
- V. Zambare, S. Nilegaonkar and P. Kanekar, New Biotechnol., 2011, 28(2), 173–181 CrossRef CAS PubMed.
- L. Liu, M. Ma, Z. Cai, X. Yang and W. Wang, Food Technol. Biotechnol., 2010, 48(2), 151–160 CAS.
- R. Jain and P. C. Jain, Indian J. Exp. Biol., 2010, 48(2), 174 CAS.
- S. K. Singh, S. K. Singh, V. R. Tripathi, S. K. Garg and S. K. Khare, Biotechnol. Prog., 2013, 29(1), 99–108 CrossRef CAS PubMed.
- X. L. Chen, Y. Z. Zhang, P. J. Gao and X. W. Luan, Mar. Biol., 2003, 143(5), 989–993 CrossRef CAS.
- A. Baehaki, Afr. J. Microbiol. Res., 2012, 6(10), 2373–2379 CAS.
- R. K. Patel, M. S. Dodia, R. H. Joshi and S. P. Singh, World J. Microbiol. Biotechnol., 2006, 22, 375–382 CrossRef CAS.
- A. Gupta, I. Roy, S. K. Khare and M. N. Gupta, J. Chromatogr. A, 2005, 1069, 155–161 CrossRef CAS PubMed.
- H. Umezawa, T. Aoyagi, H. Morishima, M. Matsuzaki, M. Hamada and T. Takeuchi, J. Antibiot., 1970, 23(5), 259–262 CrossRef CAS PubMed.
- B. Jaouadi, N. Z. Jaouadi, H. Rekik, B. Naili, A. Beji, A. Dhouib and S. Bejar, Int. J. Biol. Macromol., 2013, 60, 165–177 CrossRef CAS PubMed.
- K. D. Zaqueo, A. M. Kayano, T. F. Domingos, L. A. Moura, A. L. Fuly, S. L. da Silva, G. Acosta, E. Oliveira, F. Albericio, F. B. Zanchi, J. P. Zuliani, L. A. Calderon, R. G. Stabeli and A. M. Soares, Comp. Biochem. Physiol., Part A: Mol. Integr. Physiol., 2016, 195, 15–25 CrossRef CAS PubMed.
- R. N. Z. R. A. Rahman, L. P. Geok, M. Basri and A. B. Salleh, Enzyme Microb. Technol., 2006, 39(7), 1484–1491 CrossRef.
- C. Laane, S. Boeren, K. Vos and C. Veeger, Biotechnol. Bioeng., 1987, 30(1), 81–87 CrossRef CAS PubMed.
- M. C. Heindl, S. Fermandjian and B. Keil, Biochim. Biophys. Acta, Protein Struct., 1980, 624(1), 51–59 CrossRef CAS.
- B. Heurtault, P. Saulnier, B. Pech, J. E. Proust and J. P. Benoit, Biomaterials, 2003, 24(23), 4283–4300 CrossRef CAS PubMed.
- K. Manjusha, P. Jayesh, D. Jose, B. Sreelakshmi, P. Priyaja, P. Gopinath and I. B. Singh, Cytotechnology, 2013, 65(2), 199–212 CrossRef CAS PubMed.
- U. Eckhard, E. Schonauer and H. Brandstetter, J. Biol. Chem., 2013, 288, 20184–20194 CrossRef CAS PubMed.
- N. D. Rawlings and A. J. Barrett, Introduction: serine peptides and their clans, in Handbook of proteolytic enzymes, ed. A. J. Barrett, N. D. Rawlings and J. F. Woessner, Elsevier, London, United Kingdom, 2nd edn, 2004, pp. 1425–1427 Search PubMed.
- N. Tsuruoka, T. Nakayama, M. Ashida, H. Hemmi, M. Nakao, H. Minakata, H. Oyama, K. Oda and T. Nishino, Appl. Environ. Microbiol., 2003, 69, 162–169 CrossRef CAS PubMed.
- N. D. Rawlings, A. J. Barrett and A. Bateman, Nucleic Acids Res., 2010, 38, 227–233 CrossRef PubMed.
- T. Kato, N. Takahashi and H. K. Kuramitsu, J. Bacteriol., 1992, 174, 3889–3895 CAS.
- Y. Z. Zhang, L. Y. Ran, C. Y. Li and X. L. Chen, Appl. Environ. Microbiol., 2015, 81, 6098–6107 CrossRef CAS PubMed.
|
This journal is © The Royal Society of Chemistry 2016 |