DOI:
10.1039/C6RA07940G
(Paper)
RSC Adv., 2016,
6, 52411-52420
Dimethyl ether steam reforming to produce H2 over Ga-doped ZnO/γ-Al2O3 catalysts†
Received
27th March 2016
, Accepted 21st May 2016
First published on 23rd May 2016
1. Introduction
The increasing consumption of fossil fuels inevitably leads to both energy shortage and various environmental pollutions. So, the exploration and application of new clean energies are becoming more and more important. In recent years the fuel cells using H2 as an energy source, such as the proton exchange membrane fuel cells (PEMFCs), have attracted a lot of attention due to their multiple advantages, e.g. high energy density, rapid start response, compact size and zero pollution.1 To more cleanly and efficiently use the limited fossil fuels, the traditional fuels including hydrocarbons, alcohols and ethers are often converted to hydrogen through reforming processes. Among the reported reforming techniques, steam reforming (SR) operating at relatively low temperatures can generate more H2 due to the efficient utilization of the hydrogen element in water. For instance, the dimethyl ether steam reforming (DME SR) can generate 6 times molar amounts of H2 per molar DME, as described in eqn (1): |
(CH3)2O + 3H2O (g) → 6H2 + 2CO2, ΔH298 = 135 kJ mol−1
| (1) |
As a H2 carrier material, DME possesses a lot of advantages, such as high H/C ratio, high energy density, no toxicity and no C–C bonds in DME molecule. The absence of C–C bonds in DME greatly decreases the carbon deposition on catalysts during DME SR. All of these advantages make DME SR technique very promising in areas of onboard hydrogen production. The DME SR reaction consists of two successive steps, namely DME hydrolysis to methanol (DME H) and methanol steam reforming (MSR) as expressed in eqn (2) and (3), respectively.
|
(CH3)2O + H2O (g) → 2 CH3OH, ΔH298 = 37 kJ mol−1
| (2) |
|
CH3OH + H2O → 3H2 + CO2, ΔH298 = 49 kJ mol−1
| (3) |
The DME hydrolysis is catalyzed by solid acid catalysts, such as bayerite-derived Al2O3, γ-Al2O3, ZSM-5, H-mordenite and TiO2. The alumina-based solid acid catalysts show higher catalytic stability than the zeolite-based ones, since the strong acidity of zeolites can readily lead to the formation of coke, and decrease the activity and durability of the catalysts.2–4
The MSR reaction requires redox catalysts, such as copper and zinc-based catalysts, especially the Cu-containing one.5–9 Our previous work has reported that the CuZnAl1−xMxO (M = Zr or Ce) catalysts were active for MSR during DME SR.5,6 The partial substitution of Al by Zr or Ce can increase the dispersion of copper species, improve the reducibility of the catalysts, and inhibit the sintering of Cu crystallites in the DME SR reaction.5,6 Shimoda et al. reported that the CuFe2O4 spinel mixed with γ-Al2O3 exhibited high activity and stability for DME SR because of formation of a new active phase of Cu–Fe–AlO4 for the MSR step.7 Although the reported Cu-based catalysts displayed high performance for DME SR, they also had some shortcomings, such as high CO selectivity, low thermal stability and short catalyst durability. Thus, it is necessary to develop copper-free catalyst systems for DME SR.
In methanol conversion reactions the CO2 selectivity was highly related to the oxidation of formaldehyde. The literature reported that zinc oxide without copper was a good CO2-selective catalyst in MSR, which gave the CO2 selectivity as high as 95–99.6%.10 ZnO was beneficial to oxidization of the formaldehyde and the formaldehyde-derived intermediates toward CO2, resulting in the high CO2 selectivity.10 However, another study reported that considerable amounts of CH4 and CO could be identified during DME SR over ZnO–Al2O3 catalysts by a co-precipitation method. The presence of vast Al3+ ions probably enhanced the direct decomposition of methanol and DME, producing more CO and CH4.11 Our previous work reported that the partial substitution of Al3+ (20%) by Ce4+ in ZnAlO mixed oxide catalyst can improve the performance of ZnAlO catalyst for DME SR, and the modified ZnAlCeO catalyst exhibited higher CO2 selectivity and better start-off durability than ZnO and ZnAlO catalysts.12 The introduction of Ce not only enhanced the dispersion of Zn species but also inhibited the transformation of ZnO to spinel ZnAl2O4. In addition, the interaction between ceria and zinc oxide may produce new active sites, such as the Ce4+–O–Zn2+ linkages.12
Intrinsic ZnO is an n-type semiconductor.13 According to the electron theory of catalysis on semiconductors, the catalytic activity of semiconductor is determined by the position of the Fermi level on the surface of the crystal, which can be varied to some extent by doping the surface or the bulk of the crystal with the properly chosen impurities in proper concentrations.14 Ga atoms can substitutionally enter the lattice of ZnO acted as donors,15 and it can raise the Fermi level on the surface of ZnO. Zinc oxide catalysts modified by gallium have been proved to be active for many reactions including water splitting,16,17 DME SR18 and MSR reactions.19
Based upon the above analysis, we expected the doping of gallium could improve the catalytic performance of ZnO-based catalysts for DME SR. In this work a series of gallium-doped ZnO (GDZ) catalysts physically mixed with γ-Al2O3 (GDZ/γ-Al2O3) with different zinc/gallium molar ratios were designed and prepared. Our results show that the GDZ/γ-Al2O3 catalysts do display the remarkably improved catalytic performance, such as high DME conversion, high CO2 selectivity and long high-temperature durability, as compared with the traditional CuZnAlO catalyst physically mixed with γ-Al2O3. By using multiple techniques including XRD, TPD-MS-CH3OH, in situ FTIR of CH3OH, X-ray photoelectron spectroscopy (XPS) and four-probe direct current (DC) method, the catalysts were reasonably characterized, and the structure–performance relationship and the reaction pathways of CH3OH reforming were discussed, as well.
2. Experimental
2.1 Catalyst preparation
The GDZ catalysts with different Zn/Ga atomic ratios were prepared by co-precipitation method, using sodium carbonate as precipitant. Zn(NO3)2·6H2O and Ga(NO3)3 solution (purchased from Alfa Aesar) were used as source materials. A Na2CO3 solution with [CO32−] = 0.5 M and a mixed solution of Zn and Ga nitrates with the total cations concentration of 1.0 M were prepared, respectively. Then these two solutions were simultaneously dropped into 100 mL deionized water by keeping the pH around 7.5 under vigorous mechanical stirring at the temperature of 60 °C. After aged at the same temperature for 2 h, the obtained precipitate was filtered and washed by deionized water for three times, then dried overnight at 120 °C and finally calcined at 500 °C for 4 h. According to the Zn/Ga molar ratio, the prepared catalysts were denoted as ZnO, Zn9.5Ga0.5O, Zn9Ga1O, Zn8Ga2O and Zn7Ga3O, respectively. For comparison, a conventional CuZnAlO catalyst was also prepared, the details of which can be found elsewhere.6
Before used for the measurements of activity and durability, the GDZ and CuZnAlO catalysts were mechanically mixed with γ-Al2O3 (supplied by Tianjin Chemical Research & Design Institute, calcined at 600 °C for 2 h, SSA = 189 m2 g−1) with the mass ratio of 2
:
1 (GDZ or CuZnAlO to γ-Al2O3), and the catalysts were made into small pellets with the size of 40–60 mesh. The total acidity of γ-Al2O3 calculated by the temperature-programmed desorption of ammonia (NH3-TPD) is 50 μmol g−1. The catalysts were denoted as GDZ/γ-Al2O3 or CuZnAlO/γ-Al2O3.
2.2 Catalyst characterization
The specific surface area (SSA) of the catalysts was measured by nitrogen adsorption at −196 °C on a Quantachrome QuadraSorb SI instrument. Before the measurements, the catalysts were degassed under vacuum at 300 °C for 3 h. The specific surface area was calculated using standard BET method in 0–0.3 partial pressure range.
The crystal structure of the catalysts was characterized by X-ray diffraction (XRD). The tests were performed on Rigaku D/Max-2500 X-ray diffractometer operating at 200 mA and 40 kV, using Cu Kα (λ = 0.15418 nm) as radiation source. The diffraction data in the range of 2θ from 10° to 90° were collected at a speed of 8° min−1, and the slow scan were collected at a speed of 1° min−1 from 30° to 40°.
The temperature-programmed desorption of CH3OH (TPD-MS-CH3OH) was performed on a TPDRO instrument (Xian Quan TP-5079) connected with a mass spectrometer (Hiden HPR20). 50 mg catalyst powder (40–60 mesh) was put into the quartz tube reactor and pretreated at 400 °C for 1 h under 8% H2/N2 stream (30 mL min−1), then the feed gas was switched to pure helium flow (99.999%, 30 mL min−1) for 0.5 h at the same temperature to purge the gaseous and adsorbed H2. After the temperature cooled down to 60 °C in the same helium flow, pulses of CH3OH (1.0 μL) were injected into the catalyst bed until the MS signal got stable. Subsequently, the temperature-programmed desorption was conducted from ambient temperature to 700 °C at a heating rate of 10 °C min−1 in the same helium flow (99.999%, 30 mL min−1).
To evaluate the quantity of methanol adsorbed on the surface of the catalysts, the CH3OH pulse adsorption was also performed on the TP-5079 with a thermal conductivity detector. Similar to the test of TPD-MS-CH3OH, the catalysts were first pretreated with 8% H2/N2 and purified with helium before measurement. Then, the pulses of CH3OH (1.0 μL) were rapidly injected into the tube every 0.5 h until the detector signal got stable at 60 °C.
The in situ Fourier-transform infrared (FTIR) spectroscopies of CH3OH were performed on a Nicolet Nexus spectrometer equipped with a MCT detector cooled by liquid nitrogen. 20 mg catalyst was ground into fine powder and pressed into a thin wafer, which was then placed in a quartz IR cell connected to a high vacuum system. The catalyst was in situ pretreated for 1 h at 400 °C in 5% H2/N2 mixture gas flow with a rate of 50 mL min−1. The background spectra were collected in vacuum. Subsequently, the catalyst was exposed to CH3OH at 50 °C in N2 flow to achieve adsorption saturation. The FTIR spectra were recorded at the desired temperatures from 50 to 400 °C.
The conductivities of the catalysts were measured with a four-probe direct current (DC) method with an electrochemical workstation (VersaSTAT 3, Ametek). The powders were pressed at 18 MPa and made into rectangular bars with a typical size of 25 × 7 × 1.5 mm and then calcined at 500 °C for 3 h to form dense bars. Four silver wires with silver paste were used as electrodes. Before the test, the bars were reduced in H2 (50 mL min−1) at 400 °C for 0.5 h, and then the conductivities of the catalysts were measured in H2 atmosphere.
The X-ray photoelectron spectra were analyzed using an X-ray photoelectron spectroscopy (XPS) (K-alpha X, Thermo Fisher co., USA). The binding energies were referenced to the C1s line at 284.6 eV from adventitious carbon.
The procedures of NH3-TPD and thermal gravimetric analysis (TG) can be obtained in ESI.†
2.3 Catalytic activity and durability tests
The evaluation of catalytic activity and durability of the catalysts was performed in a continuous flow fixed-bed reactor under atmospheric pressure. Before activity evaluation, 500 mg catalyst was loaded in the quartz reactor and in situ reduced at 400 °C for 0.5 h in 10% H2/N2 (50 mL min−1). The feedstock for activity and durability tests is composed of N2 (40%), H2O vapor (50%) and DME (10%) with the steam/carbon molar ratio (S/C) fixed at 2.5, showing a space velocity of 12
000 mL h−1 gcat−1. The external and internal mass transfer limitations have already been excluded by variation of the linear flow rate over the catalyst and the catalyst particle size, respectively. A mixture gas of DME and N2 was directly fed to the reactor, and the water was vaporized at 150 °C before entering the reactor. For activity measurements the reaction temperature varied in the range of 370–450 °C, while for durability tests the reaction temperature was fixed at 440 °C. The compositions of the influent and effluent gases containing DME, CH4, CH3OH, N2, CO, CO2 and H2 were analyzed by an Agilent 7890A chromatograph (GC). DME, CH4 and CH3OH were separated by a PoraPak Q capillary column and analyzed by a flame ionization detector (FID), while N2, CO and CO2 were separated by a PoraPak N column and a MoleSieve 5A column, and analyzed by a thermal conductivity detector (TCD). H2 was analyzed by TCD with N2 as carrier gas. The DME conversion (XDME), H2 yield (yH2) and the selectivity (SCi) to Ci (including CO2, CO and CH4) are defined as follows: |
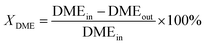 | (4) |
|
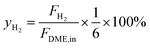 | (5) |
|
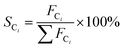 | (6) |
here, DMEin, and DMEout stand for the molar flow rate of DME in feed gas and product gas. FH2 represents the effluent molar flow rate of H2, and FCi is the molar flow rate of the Ci products.
3. Results and discussion
3.1 Catalysts characterization
3.1.1 Texture and bulk structure of the catalysts. The XRD patterns of the catalysts are displayed in Fig. 1(a). The pattern of the ZnO catalyst shows peaks matching with the zincite structure [JCPDS 36-1451]. The same peaks were also observed on the GDZ catalysts, and no other phase was detected. Additionally, compared with the pure ZnO catalyst, the intensity of the XRD peaks are noticeably reduced with the increase of the Ga content in the catalysts. It suggests the gradually decreased particle size and crystallinity. By applying the Scherrer equation, the average crystallite size has been estimated to be 45.5 nm for the pure ZnO, 11.7, 9.0, 4.4 and 3.9 nm for the catalysts Zn9.5Ga0.5O, Zn9Ga1O, Zn8Ga2O and Zn7Ga3O, respectively.
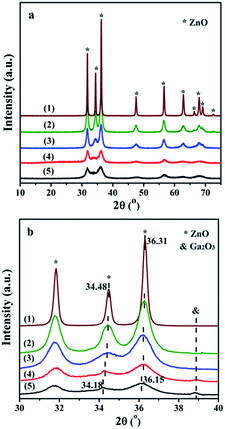 |
| Fig. 1 XRD patterns in the region of (a) 10° to 75° with a speed of 8° min−1 and (b) 30° to 40° with a speed of 1° min−1: (1) ZnO, (2) Zn9.5Ga0.5O, (3) Zn9Ga1O, (4) Zn8Ga2O and (5) Zn7Ga3O. | |
Furthermore, Fig. 1(b) shows the slow scan XRD patterns. Doping of ZnO with Ga causes the shift of the XRD peaks towards small angles. The peak corresponding to (002) plane shifted from 34.48 to 34.18°, and the peak corresponding to (101) plane shifted from 36.31 to 36.15°. The shift of the diffraction peaks indicates the GDZ catalysts still keep the zincite structure and Ga is successfully incorporated into the crystal lattice causing the lattice distortion. Wei et al. reported that the interplanar spacing of the (101) plane in Ga-doped ZnO catalysts shifted from 0.2468 to 0.2473 nm.20 According to the semiconductor theory, the Ga cations in the zincite structure probably exist in the form of Ga3+·e, which can increase the conductivity and the electron-donating ability of the catalyst. A weak diffraction peak of gallium oxide (Ga2O3 [JCPDS 06-0509]) appears in the Zn8Ga2O pattern, and it becomes stronger in the Zn7Ga3O pattern. It indicates that partial of Ga exists in the form of Ga2O3 on the GDZ catalysts.
The results of the specific surface areas of the catalysts are summarized in Table 1. The surface areas are enlarged with the Ga contents increased.
Table 1 Specific surface (SSA), average crystallite size (ACS), CH3OH adsorption amounts (MAA), apparent activation energy (Ea) and TOF of different catalysts
Catalyst |
SSA (m2 g−1) |
ACS (nm) |
MAAa (mmol g−1) |
Eab (kJ mol−1) |
TOFc (s−1) |
Methanol adsorption amounts (MAA) was calculated from the area of the peaks in the CH3OH pulse adsorption profiles. Apparent activation energy (Ea) was estimated from Arrhenius equation based on the activity over all the catalysts (50 mg) at 1 atm total pressure (space velocity of 12 000 mL h−1 gcat−1. 10% DME, 50% H2O and 40% N2). Turnover frequency (TOF) was estimated based on the MAA results and the activity tests over all the catalysts (50 mg) at 430 °C and 1 atm total pressure (space velocity of 12 000 mL h−1 gcat−1. 10% DME, 50% H2O and 40% N2). |
ZnO |
9.2 |
45.5 |
0.05 |
— |
— |
Zn9.5Ga0.5O |
57.0 |
11.7 |
0.31 |
213.6 ± 4.4 |
0.013 |
Zn9Ga1O |
68.8 |
9.0 |
0.34 |
197.4 ± 2.7 |
0.019 |
Zn8Ga2O |
80.2 |
4.4 |
0.33 |
203.6 ± 11.0 |
0.016 |
Zn7Ga3O |
93.2 |
3.9 |
0.35 |
210.2 ± 5.9 |
0.015 |
3.1.2 Electrical conductivity of the catalysts. Doping of semiconductor can change the position of the Fermi level on the surface and in the bulk. The position of Fermi level will affect both the electrical conductivity and the catalytic activity, simultaneously.14 Therefore, there is a correlation, which is related to the type of reaction (n-type or p-type reaction) and the type of semiconductor (n-type or p-type), between the catalytic activity and the electrical conductivity.14 ZnO is an n-type semiconductor. Generally, Ga can be doped into the ZnO crystal lattice as a donor impurity and be considered in the form of Ga3+·e, which will increase the conductivity of GDZ catalysts. However, excessive Ga will also lead to the formation of Ga2O3, reducing the conductivity of GDZ catalysts. The conductivity data of the catalysts are displayed in Fig. 2. The conductivity of all of the GDZ catalysts increase with the rising temperature, and the Zn9Ga1O catalyst shows the highest conductivity. The data of the ZnO catalyst are not given, because the undoped ZnO has unstable electrical properties.15 These results indicate that Ga atoms have been doped into ZnO crystal lattice and lead to the increase of conductivity, which is in accordance with the XRD results.
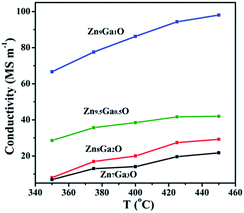 |
| Fig. 2 The conductivity of the GDZ catalysts. | |
3.1.3 TPD-MS-CH3OH. Methanol is an intermediate product in DME SR, and its adsorption and activation on catalyst surface is rather important. The methanol adsorption behaviour on the catalyst surface was investigated by TPD-MS-CH3OH. Table 1 lists the methanol adsorption amounts (MAA) of the catalysts measured by pulse adsorption. In the TPD-MS-CH3OH tests, the MS signals of the desorbed CH3OH, H2, CO2 and H2O were simultaneously monitored, as shown in Fig. 3(a–d), respectively. Fig. 3(a) shows that the desorption of CH3OH from the pure ZnO takes place at around 100 °C with a small peak area, suggesting that only small amount of CH3OH can be adsorbed on ZnO, similarly to the previous report.12 The desorption signals of CH3OH from the GDZ catalysts exhibits two desorption peaks with much larger peak areas than that from ZnO. The peak in the range of ∼60–200 °C is attributed to the desorption of molecularly adsorbed methanol, and the top of the peak gradually increase from 98 to 115 °C with the increase of Ga in the catalyst, indicating doping of Ga raises the ability for methanol adsorption on the catalyst. The signal of desorption of CH3OH above 200 °C mainly comes from the rehydrogenation of methoxy species by hydroxyl groups.21 No methanol was desorbed on ZnO above 200 °C in Fig. 3(a). Probably, there is no chemically adsorbed methanol on ZnO under the present experimental conditions. It can also be verified by the CO2 desorption (Fig. 3(b)) and H2 desorption (Fig. 3(c)) that there is also no CO2 and H2 desorption peak for ZnO. For comparison, we carried out a TPD-MS-CH3OH experiment with adsorption of CH3OH on ZnO at 100 °C. The corresponding results are plotted in Fig. S1.† In Fig. S1,† the desorption signal of CH3OH, CO2 and H2 can be clearly observed. It indicates that methanol is hardly chemically adsorbed over ZnO at 60 °C until the adsorption temperature increases to 100 °C. Nevertheless, doping Ga into ZnO is an efficient way to enhance the chemical adsorption of methanol, which occurs even at 60 °C, as shown in Fig. 3. The desorption profiles of CO2 (Fig. 3(b)) show that the initial desorption temperature of CO2 of the GDZ catalysts is at about 260–280 °C. With the increase of Ga in the catalyst, the temperature of the desorption peak shifted from 336 (Zn9.5Ga0.5O) to 326 °C (Zn9Ga1O) and then raises to 338 °C (Zn7Ga3O). In Fig. 3(c), the temperature for H2 desorption from the catalysts has a similar trend with the CO2 desorption. The H2 desorption of the Zn9Ga1O catalyst shows the lowest temperature. The initial temperature of H2 desorption is at about 230 °C for the GDZ catalysts, which is lower than that of CO2 (about 260–280 °C). Moreover, the temperature of the H2 desorption peak is also lower than CO2. Thus, it can be deduced that the formation or desorption of CO2 might be the rate-controlling step of DME SR. Two H2O desorption peaks are observed in Fig. 3(d). The peak below 400 °C is attributed to the desorption of molecular H2O formed during CH3OH adsorption, and the peak above 400 °C is ascribed to the water produced from the interaction of surface hydroxyls.12 The surface hydroxyls play a vital role in the reaction from CH3OH to CO2 and H2. Chadwick and Zheng found that over the dehydroxylated ZnO catalyst the dominant reaction was the decomposition of methanol to form CO and H2 rather than the MSR to form CO2 and H2.22 In addition, the formation of hydrogen often takes place via the interaction of surface hydroxyl groups.23 Herein, above 400 °C the GDZ catalysts show the larger peak area of water desorption signals than that of ZnO, indicating that the former may have higher catalytic activities for DME SR.
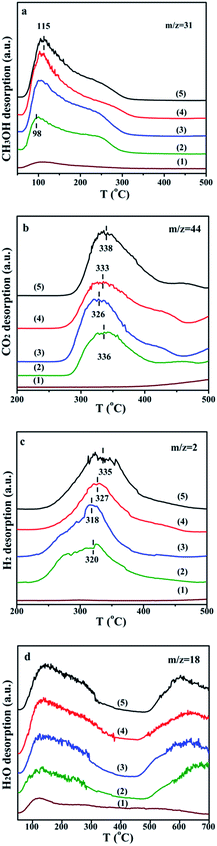 |
| Fig. 3 The signals of desorption of (a) CH3OH, (b) CO2, (c) H2 and (d) H2O in TPD-MS-CH3OH over (1) ZnO, (2) Zn9.5Ga0.5O, (3) Zn9Ga1O, (4) Zn8Ga2O and (5) Zn7Ga3O. | |
3.2 Activity and stability
3.2.1 Activity on DME SR. Fig. 4 shows the conversion of DME, the yield of hydrogen and the selectivity of CO and CO2 of the catalysts as a function of the reaction temperature. Zn9Ga1O/γ-Al2O3 exhibits the best catalytic performance as compared with others. At 400 °C, the GDZ/γ-Al2O3 catalysts exhibit higher DME conversion (13–20%) than that of pure ZnO/γ-Al2O3 (∼10%). With the temperature increasing to 450 °C, Zn9Ga1O/γ-Al2O3 displays a DME conversion as high as 95%, which is much higher than that for pure ZnO (65%). Several assumptions to estimate the apparent activation energy of DME SR over different catalysts were put up by Eguchi et al.24 namely: (1) it is a pseudo-first-order reaction, (2) the reactor is an isobaric plug flow reactor, and (3) side reactions are neglected. Based on these assumptions, corresponding Arrhenius plots for DME SR over this series of catalysts are obtained (Fig. S2†). The good linearity of Arrhenius plots indicates that the assumptions are acceptable for the GDZ/γ-Al2O3 catalysts. The obtained apparent activation energy (Ea) and turnover frequency (TOF) of different catalysts are listed in Table 1. Zn9Ga1O/γ-Al2O3 shows the lowest Ea (197.4 kJ mol−1) and highest TOF (0.019 s−1) values, which is in accordance with the highest DME conversion. The hydrogen yield (yH2) exhibits the same trend with the DME conversion as seen in Fig. 4(b), the values of the H2 yield and the DME conversion are similar, indicating that DME almost transform to H2 and CO2. During DME SR, some side reactions might occur,4–6 and the main by-products are CO and CH4. As shown in Fig. 4(c), all catalysts exhibit the CO selectivity less than 5% in the whole reaction temperature range, except Zn7Ga3O. The selectivity of CO is much lower than that of our previously reported CuZnAlO based catalysts.5,6 The small amount of CO may come from some potential side reactions such as reverse water gas shift reaction (r-WGS) and methanol decomposition. Moreover, CH4 was not found in the product. Thus, except Zn7Ga3O, all catalysts exhibit higher CO2 selectivity than 95% in the whole reaction temperature range, as shown in Fig. 4(d).
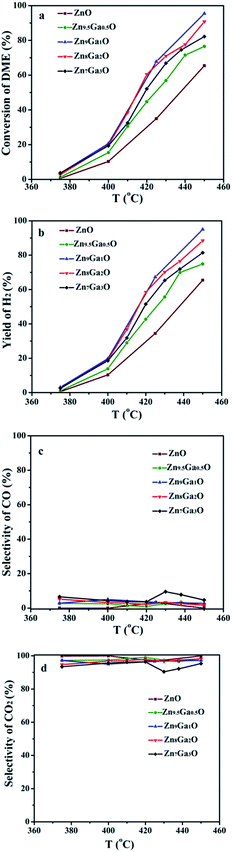 |
| Fig. 4 (a) Conversion of DME, (b) yield of H2, (c) selectivity of CO and (d) selectivity of CO2 for the DME SR reaction. | |
With the increase of Ga content, the conversion of DME and the yield of hydrogen increased and then decreased. The XRD results showed that Ga was doped into the crystal lattice of ZnO. As shown in Fig. 2, doping of Ga had caused the change of electrical conductivity. The relationship between electrical conductivity and catalytic performance is displayed in Fig. 5. Compared with the change of the conductivity of GDZ catalysts, the change of the conversion of DME and the yield of hydrogen displayed a similar trend. The electron theory of catalysis on semiconductor indicated in the case of an n-type reaction on an n-type semiconductor, the electrical conductivity and the activity in the same direction.14 Our results indicate that the rate-controlling step of DME SR over GDZ/γ-Al2O3 catalysts is an n-type reaction. It can be accelerated by doping donor in the lattice of n-type semiconductor of ZnO, which can enhance the donor ability. The rate-controlling step may change with the increase of donor. It indicates that a proper electrical conductivity is helpful to improve the activity and selectivity of the catalyst. In this work, the highest electrical conductivity corresponds to the highest catalytic activity. It indicates that increasing the conductivity of GDZ catalysts probably further enhance the activity of the catalyst. Moreover, as listed in Table 1, with the increase of the amount of gallium, the specific surface areas of the GDZ catalysts significantly enlarged. Thus, although the conductivity of Zn7Ga3O and Zn8Ga2O was lower than that of Zn9.5Ga0.5O, the formers still presented the higher catalytic activity than the later, since the specific surface areas of Zn8Ga2O (80.2 m2 g−1) and Zn7Ga3O (93.2 m2 g−1) are much larger than that of Zn9.5Ga0.5O (57 m2 g−1).
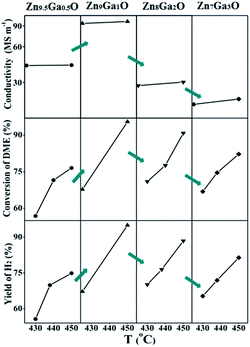 |
| Fig. 5 The trend of conversion of DME, yield of H2 and conductivity. | |
Understanding the formation and desorption of surface species is helpful to the improvement of catalyst. According to the results of TPD-MS-CH3OH, the initial desorption temperature of hydrogen on the GDZ catalysts is about 230 °C, which is lower than that of CO2 (about 260–280 °C). The top temperature of the H2 desorption peak is also lower than that of CO2. We found that the trend of the conversion of DME, as well as the yield of hydrogen, is similar to the trend of the CO2 desorption temperature. The lower desorption temperature of CO2 matches the higher activity and selectivity. Thus, we deduced that the formation of CO2 might be the rate-controlling step of DME SR using GDZ/γ-Al2O3 as catalysts. The signals of CO2 and H2 suggest that Zn9Ga1O has the highest activity to convert CH3OH to H2 and CO2, as shown in Fig. 4(b and c). Enhancing the ability of GDZ catalysts to convert CH3O– into CO2 will contribute to the improvement of the catalytic activity of DME SR.
3.2.2 Durability test on the Zn9Ga1O/γ-Al2O3 and CuZnAlO/γ-Al2O3 catalysts. To investigate the stability of this series of catalysts for DME SR, the Zn9Ga1O/γ-Al2O3 catalyst was selected for the prolonged durability test, which is carried out at 440 °C for 48 h. Meanwhile, an identical experiment was performed on the conventional CuZnAlO/γ-Al2O3 catalyst for a direct comparison. The results are shown in Fig. 6. For the CuZnAlO/γ-Al2O3 catalyst, the DME conversion (around 98%) shows little change in the first 8 h, but after this period it declines rapidly, giving the final conversion of 62% at 48 h. For the catalyst Zn9Ga1O/γ-Al2O3, it possesses an initial DME conversion of ∼90%, a little lower than that of CuZnAlO/γ-Al2O3, but it decreases more slowly, showing the conversion of 73% in the end. The total decreased DME conversion over the catalyst Zn9Ga1O/γ-Al2O3 during 48 h test is about 17%, much lower than that on the CuZnAlO/γ-Al2O3 catalyst (36%). We deduce that the good stability of the GDZ/γ-Al2O3 catalysts probably originates from its high thermal stability and the ability of resistance of coke deposition. As for the CuZnAlO/γ-Al2O3 catalyst, the catalytic deactivation mainly results from the readily sintered metallic Cu species and the coke deposition on the catalyst at the temperature above 300 °C, as indicated by our XRD and TG results (Fig. S3 and S4†) in ESI.
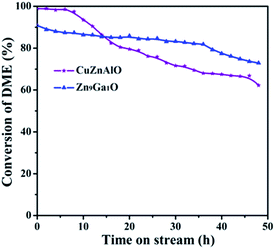 |
| Fig. 6 The stability of DME SR over the Zn9Ga1O/γ-Al2O3 and CuZnAlO/γ-Al2O3 catalysts. | |
3.3 Mechanism of DME SR over GDZ/γ-Al2O3 catalyst
3.3.1 XPS results. In order to identify the change of the distribution and content of surface oxygen species caused by Ga doping into zinc oxide, X-ray photoelectron spectroscopy (XPS) was employed. Fig. 7 shows the oxygen 1s (O 1s) peaks of ZnO and Zn9Ga1O catalysts. The O 1s signals of the Zn9Ga1O catalyst pre-reduced in 5% H2/N2 for 0.5 h before XPS test is also given in Fig. 7. The typical O 1s peak can be consistently fitted by three nearly Gaussian, centered at 532.3, 530.8 and 531.5 eV, respectively.25–27 The high binding energy component located at 532.3 eV is usually attributed to the adsorbed O2−.25 The component on the low binding energy side of O 1s spectrum (530.8 eV) is attributed to the bulk O2−.26 The medium binding energy component centered at 531.5 eV is associated with O2− ions in the oxygen deficient regions with the matrix of ZnO.27 So, changes in the intensity of this component may be connected in part to the variations in the concentration of oxygen vacancies. In this work, Fig. 7 shows that the O 1s peaks of the Zn9Ga1O catalyst slightly shifted towards higher binding energy, as compared with ZnO. The peak shift is attributed to the difference in the electronegativity of Zn and Ga. The valence electron density of Zn and O in the Zn–O–Ga bond in the Zn9Ga1O catalyst becomes lower than that in the Zn–O–Zn bond in the ZnO catalyst because of the higher electronegativity of Ga than that of Zn. As a result, the interaction between Zn and O is weakened, which leads to the increases in the binding energy of O 1s peaks.26,27
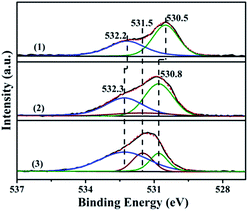 |
| Fig. 7 The XPS spectra in the O 1s region of the ZnO and Zn9Ga1O catalysts: (1) ZnO, (2) Zn9Ga1O and (3) Zn9Ga1O pretreated in 8% H2/N2 for 0.5 h before XPS test. | |
In order to compare the cases of the GDZ and ZnO catalysts, the relative intensities of the Zn9Ga1O, pretreated Zn9Ga1O and ZnO catalysts are calculated and are listed in Table 2. As described in Table 2, the Zn9Ga1O and pretreated Zn9Ga1O have higher values of the medium binding energy component than ZnO, confirming that the oxygen vacancy increased after the Ga element incorporated into zinc oxide crystal lattice. After the Zn9Ga1O catalyst was pretreated by H2, the percentage of lattice oxygen decreased continuously, and the oxygen vacancy increased. The oxygen vacancy plays an important role in the adsorption of reactants and it is very important for DME SR.
Table 2 Relative intensity of O 1s peaks of ZnO and Zn9Ga1O catalysts, evaluated by XPS
Catalyst |
Fraction O state |
532.3 eV (%) |
531.5 eV (%) |
530.8 eV (%) |
The Zn9Ga1O was reduced in 8% H2/N2 for 0.5 h before characterized by XPS measurement. |
ZnO |
44.5 |
0 |
55.4 |
Zn9Ga1O |
42.9 |
9.3 |
47.8 |
Zn9Ga1Oa |
56.8 |
21.4 |
21.8 |
To confirm the effect of oxygen vacancies, the catalytic tests of the Zn9Ga1O/γ-Al2O3 pretreated in different atmospheres were also evaluated. As shown in Fig. 8, at 425 °C the conversion of DME over the pre-reduced Zn9Ga1O/γ-Al2O3 catalyst can reaches 67.8%, while it is only 48.5% and 44.3% over the Zn9Ga1O/γ-Al2O3 catalyst pretreated in pure N2 and the fresh one, respectively. Apparently, the pre-reduction treatment can significantly improve the catalytic activity of Zn9Ga1O/γ-Al2O3. So, it indicates that the increase of the number of oxygen vacancies can improve the catalytic activity for DME SR over the GDZ/γ-Al2O3 catalyst.
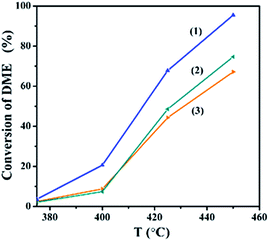 |
| Fig. 8 The conversion of DME over the Zn9Ga1O/γ-Al2O3 catalyst under different pretreatments: (1) pre-reduced in 8% H2/N2 mixture gas, (2) pretreated in pure N2, (3) un-pretreated. | |
3.3.2 In situ FTIR of CH3OH results. In order to infer the reaction route, the thermal evolution of the adsorbed CH3OH and other species on the Zn9Ga1O catalyst surface was monitored by in situ FTIR spectroscopy. The temperature-dependent IR spectra and the assignments of bands in the spectra are shown in Fig. 9 and Table 3, respectively. At 50 °C, a series of the characteristic peaks corresponding to molecularly adsorbed methanol can be observed. The peaks at 3709 cm−1 and 3664 cm−1 are assigned to the O–H stretching vibration [ν (OH)] of methanol molecules.28 The signal at 3006 cm−1 belongs to the C–H stretching vibration in the methyl group [ν (CH3)] of methanol molecules,29 and the band around 1032 cm−1 is attributed to the C–O stretching vibration [ν (CO)] of CH3OH molecule.21 Additionally, methoxy species are also detectable, with the bands appearing at 3000–2800 cm−1 (2961, 2926, 2868, 2821 cm−1), ∼1453 cm−1 and 1100–1000 cm−1 (1070 and 1030 cm−1), which correspond to ν (CH),29,30 δs (CH3)29 and ν (CO),21,30 respectively. The detected methoxy species at the low temperature of 50 °C are mainly assigned to the methoxy in methanol molecule. With the increase of temperature to 100 °C, the absorption intensity of adsorbed methanol molecule is weakened. A broad band in the region of 3600–3300 cm−1 assigned to the ν (OH) of adsorption H2O29 and an absorption band at ∼1610 cm−1 assigned to the δ (HOH) of adsorption H2O appeared.31 Negative signals between 3750 and 3580 cm−1 also appeared, indicating that a perturbation or a consumption of the surface –OH groups on the catalyst occurred after the methanol adsorption. These results indicate that the hydroxyl groups on the surface of GDZ catalyst react with methanol to give methoxy and water molecules, which is in accordance with the results of TPD-MS-CH3OH. With the temperature further increased to 150 °C or higher, the methoxy species gradually decrease. When the temperature reaches 200 °C, some new bands emerge at the positions of 2961, 1580, and 1369 cm−1, which can be attributed to formate species. More specifically, the peak at 2961 cm−1 is ascribed to stretching of carboxylate and bending vibration of C–H mode (HCOO–).30 The bands at about 1580 cm−1 can be assigned to νas (COO) and 1369 cm−1 assigned to νs (COO), respectively.32 With the temperature increasing to 250 °C the intensity of the absorption bands for formate species is strengthened, suggesting the transformation of more CH3O– to HCOO–. At this temperature the peaks of HCOO– shift to lower wavenumbers, and wavenumber of the νas (COO) shifts from 1580 cm−1 to 1568 cm−1. Gaseous CO2 peaks centered at 2372 and 2337 cm−1 emerged at 300 °C.31 This means that the formate species and carbonate species turned into carbon dioxide, and this is coincident with the TPD-MS-CH3OH. The appearance of gaseous CO2 absorption bands further demonstrates that the formed intermedium HCOO– species prefers to turn into CO2. The amount of HCOO– species increases with the temperature elevated from 250 to 300 °C. Above 300 °C, the amount of HCOO– species decreases with the temperature further increasing, but the intensity of gaseous CO2 is continuously strengthened, which suggests that more and more HCOO– species are transformed into CO2.
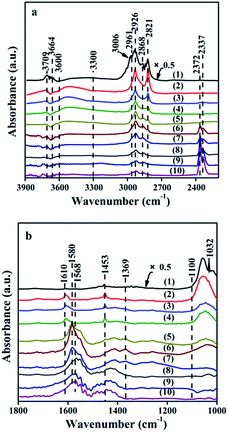 |
| Fig. 9 In situ FTIR spectra of adsorbed CH3OH at different temperatures over the Zn9Ga1O catalyst: (1) 50 °C, (2) 100 °C, (3) 150 °C, (4) 200 °C, (5) 250 °C, (6) 300 °C, (7) 325 °C, (8) 350 °C, (9) 375 °C and (10) 400 °C. | |
Table 3 In situ FTIR assignments of surface species of adsorbed CH3OH over Zn9Ga1O catalyst
Groups |
Vibrational mode |
Wavenumber (cm−1) |
Ref. |
H2O |
δ (H2O) |
1610 |
31 |
–OH |
ν (OH) |
3664, 3709 |
28 |
3600–3300 |
29 |
CH3O– |
ν (CO) |
1032 |
21 |
1100–1000 |
21 and 30 |
νas (CH3) |
3006 |
29 |
2961, 2926 |
30 |
νs (CH3) |
2868 |
29 |
2821 |
30 |
δs (CH3) |
1453 |
29 |
HCOO– |
δ (CH) |
2961 |
30 |
νs (COO–) |
1369 |
32 |
νas (COO–) |
1580 |
32 |
CO2 |
Physisorbed CO2 |
2372, 2337 |
31 |
3.3.3 The pathway of DME SR over the GDZ/γ-Al2O3 catalyst. The GDZ catalysts mechanically mixed with γ-Al2O3 have been proved to be active for the DME SR reaction, especially the extremely high CO2 selectivity. In order to understand DME SR over the catalysts, we deduce a reaction mechanism according to the results of XPS, in situ FTIR of CH3OH and the TPD-MS-CH3OH. The proposed mechanism is shown in Fig. 10. It has been proved that the DME SR reaction consists of two successive steps, namely DME hydrolysis to methanol (DME H) (eqn (2)) and methanol steam reforming (MSR) (eqn (3)). In this work, the DME hydrolysis is catalyzed by γ-Al2O3, which acted as a solid acid catalyst. We mainly focus on the MSR step. As shown in XPS, the reduced GDZ catalyst has a lot of oxygen vacancies under reducing atmosphere, and these oxygen vacancies usually are considered as the active sites for the chemisorption of methanol and water. The in situ FTIR shows that when the temperature reaches 100 °C, methoxy species and water appear. The signals of desorbed water also have been observed in TPD-MS-CH3OH above 50 °C. We infer that methanol molecules are adsorbed on the oxygen vacancies of GDZ catalyst, and it dissociate to methoxy species and adsorbed hydrogen atoms (Ha) (eqn (7)), then the adsorbed hydrogen atoms react with the surface hydroxyls to form water molecules. The dissociation of methanol molecules to methoxy species is a fast step since it can occur at a low temperature. We infer that the adsorbed water molecules can also dissociate to form the adsorbed hydroxyls (HOa) and adsorbed hydrogen atoms (eqn (8)) on the oxygen vacancies, and this process is a fast reversible process due to the easy desorption of water. As shown in Fig. 4(d), we infer that the adsorbed hydroxyls can further dissociate to form the adsorbed hydrogen atoms and adsorbed oxygen atoms (eqn (9)). The adsorbed hydrogen atoms can combine to form the hydrogen molecules (eqn (10)), which have been detected by TPD-MS-CH3OH above 220 °C. |
CH3OH + □ → CH3Oa + Ha
| (7) |
□: oxygen vacancy.
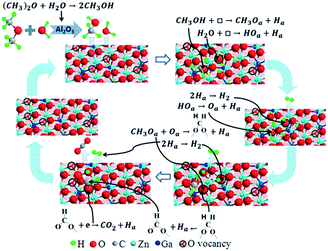 |
| Fig. 10 The proposed mechanism for DME SR over the GDZ/γ-Al2O3 catalyst. | |
The appearance of signals of HCOO– in in situ FTIR at 200 °C proved that the CH3O– species transformed into HCOO–. We infer the equations (eqn (11) and (12)) for this process.
|
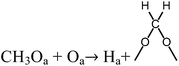 | (11) |
|
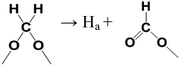 | (12) |
The CO2 signals appeared in TPD-MS-CH3OH test at about 270 °C. Moreover, at 300 °C, new bands corresponding to gaseous CO2 (2372, 2337 cm−1) are observed in in situ FTIR spectra. The appearance of the gaseous CO2 demonstrates that the formed intermedium HCOO– prefers to turn into CO2 (eqn (13)), which can provide a strong evidence for the high CO2 selectivity of DME SR on the GDZ/γ-Al2O3 catalysts (Fig. 4(d)).
|
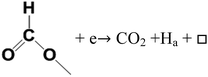 | (13) |
We found that CO2 was generated at the highest temperature in all steps. Meanwhile, the temperature of CO2 desorption showed in TPD-MS-CH3OH has a similar trend with the conversion of DME. Thus, we deduce that the transformation from HCOO– to CO2 is the controlling step for MSR over the GDZ catalyst. As discussed in 3.2.1, the rate-controlling step of DME SR over GDZ/γ-Al2O3 catalysts is an n-type reaction. Therefore, we deduce the transformation from HCOO– to CO2 is an n-type reaction, which can be accelerated by doping donor in the lattice of n-type semiconductor, and we have accelerated the transformation from HCOO– to CO2 by the doping of ZnO with Ga.
4. Conclusion
A series of gallium-doped zinc oxide (GDZ) mixed with γ-Al2O3 catalysts for DME SR to produce H2 was prepared. Compared with the conventional catalyst, GDZ/γ-Al2O3 catalysts exhibited higher carbon dioxide selectivity and better stability. XRD results indicated that Ga atoms incorporated into the crystal lattice of ZnO. With the increase of gallium content, the conductivity of GDZ catalysts increased and then decreased, similar with the conversion of DME trend and the yield of hydrogen trend. When the molar ratio of Ga
:
Zn is 1
:
9, the GDZ catalyst has the highest conductivity, and the corresponding Zn9Ga1O/γ-Al2O3 catalyst has the highest conversion of DME (95.4%) and yield of hydrogen (95%). The results of conductivity indicate that the rate-controlling step of DME SR over the GDZ catalysts is an n-type reaction. XPS results indicated that doping of gallium introduced a large number of oxygen vacancies into the catalyst, and the vacancies were beneficial to the reaction. According to the results of XPS, TPD-MS-CH3OH, in situ FTIR of CH3OH and the proposed mechanism with HCOO– as an intermedium, we speculated that the formation of carbon dioxide might be the rate-controlling step for DME SR over GDZ/γ-Al2O3 catalysts. We deduce the transformation from HCOO– to CO2 is an n-type reaction. The good conversion of DME, high selectivity of CO2 and high stability of GDZ/γ-Al2O3 catalysts makes it promising for DME SR both in research and industry.
Acknowledgements
This work is financially supported by National Natural Science foundation of China (No. 21476159, 21476160), the Natural Science Foundation of Tianjin (No. 15JCZDJC37400), the 973 program (2014CB932403), and the Program for Introducing Talents of Discipline to Universities of China (No. B06006).
Notes and references
- B. Chutichai, S. Authayanun, S. Assabumrungrat and A. Arpornwichanop, Energy, 2013, 55, 98–106 CrossRef CAS.
- K. Faungnawakij, R. Kikuchi, T. Matsui, T. Fukunaga and K. Eguchi, Appl. Catal., A, 2007, 333, 114–121 CrossRef CAS.
- K. Faungnawakij, Y. Tanaka, N. Shimoda, T. Fukunaga, S. Kawashima, R. Kikuchi and K. Eguchi, Appl. Catal., A, 2006, 304, 40–48 CrossRef CAS.
- T. A. Semelsberger, K. C. Ott, R. L. Borup and H. L. Greene, Appl. Catal., B, 2006, 65, 291–300 CrossRef CAS.
- Z. Sun, M. Meng, L. Zhang, Y. Zha, X. Zhou, Z. Jiang, S. Zhang and Y. Huang, Int. J. Hydrogen Energy, 2012, 37, 18860–18869 CrossRef CAS.
- L. Zhang, M. Meng, S. Zhou, Z. Sun, J. Zhang, Y. Xie and T. Hu, J. Power Sources, 2013, 232, 286–296 CrossRef CAS.
- N. Shimoda, K. Faungnawakij, R. Kikuchi, T. Fukunaga and K. Eguchi, Appl. Catal., A, 2009, 365, 71–78 CrossRef CAS.
- X. Zhou, M. Meng, Z. Sun, Q. Li and Z. Jiang, Chem. Eng. J., 2011, 174, 400–407 CrossRef CAS.
- D. Feng, Y. Wang, D. Wang and J. Wang, Chem. Eng. J., 2009, 146, 477–485 CrossRef CAS.
- H. Lorenz, M. Friedrich, M. Armbrüster, B. Klötzer and S. Penner, J. Catal., 2013, 297, 151–154 CrossRef CAS PubMed.
- M. Yang, Y. Men, S. Li and G. Chen, Int. J. Hydrogen Energy, 2012, 37, 8360–8369 CrossRef CAS.
- L. Zhang, M. Meng, X. Wang, S. Zhou, L. Yang, T. Zhang, L. Zheng, J. Zhang and T. Hu, J. Power Sources, 2014, 268, 331–340 CrossRef CAS.
- T. Minami, MRS Bull., 2000, 25, 38–44 CrossRef CAS.
- T. Wolkenstein, Adv. Catal., 1960, 12, 189–264 Search PubMed.
- W. Göpel and U. Lampe, Phys. Rev. B: Condens. Matter Mater. Phys., 1980, 22, 6447 CrossRef.
- K. Maeda, K. Teramura and K. Domen, J. Catal., 2008, 254, 198–204 CrossRef CAS.
- X. Wang, S. Shen, S. Jin, J. Yang, M. Li, X. Wang, H. Han and C. Li, Phys. Chem. Chem. Phys., 2013, 15, 19380–19386 RSC.
- T. Mathew, Y. Yamada, A. Ueda, H. Shioyama, T. Kobayashi and C. S. Gopinath, Appl. Catal., A, 2006, 300, 58–66 CrossRef CAS.
- W. Tong, K. Cheung, A. West, K.-M. Yu and S. C. E. Tsang, Phys. Chem. Chem. Phys., 2013, 15, 7240–7248 RSC.
- H. Wei, M. Li, Z. Ye, Z. Yang and Y. Zhang, Mater. Lett., 2011, 65, 427–429 CrossRef CAS.
- S. E. Collins, L. E. Briand, L. A. Gambaro, M. A. Baltanás and A. L. Bonivardi, J. Phys. Chem. C, 2008, 112, 14988–15000 CAS.
- D. Chadwick and K. Zheng, Catal. Lett., 1993, 20, 231–242 CrossRef CAS.
- K. A. Pokrovski and A. T. Bell, J. Catal., 2006, 241, 276–286 CrossRef CAS.
- K. Faungnawakij, T. Fukunaga, R. Kikuchi and K. Eguchi, J. Catal., 2008, 256, 37–44 CrossRef CAS.
- P.-T. Hsieh, Y.-C. Chen, K.-S. Kao and C.-M. Wang, Appl. Phys. A: Mater. Sci. Process., 2008, 90, 317–321 CrossRef CAS.
- G. C. Park, S. M. Hwang, J. H. Lim and J. Joo, Nanoscale, 2014, 6, 1840–1847 RSC.
- X. Duan, C. Song, F. Yu, D. Yuan and X. Li, Appl. Surf. Sci., 2011, 257, 4291–4295 CrossRef CAS.
- A. Vimont, J. Lavalley, A. Sahibed-Dine, C. Otero Areán, M. Rodríguez Delgado and M. Daturi, J. Phys. Chem. B, 2005, 109, 9656–9664 CrossRef CAS PubMed.
- M. M. Branda, S. E. Collins, N. J. Castellani, M. A. Baltanás and A. L. Bonivardi, J. Phys. Chem. B, 2006, 110, 11847–11853 CrossRef CAS PubMed.
- P. H. Matter and U. S. Ozkan, J. Catal., 2005, 234, 463–475 CrossRef CAS.
- R. W. Stevens Jr, R. V. Siriwardane and J. Logan, Energy Fuels, 2008, 22, 3070–3079 CrossRef.
- M. Calatayud, S. Collins, M. Baltanas and A. Bonivardi, Phys. Chem. Chem. Phys., 2009, 11, 1397–1405 RSC.
Footnote |
† Electronic supplementary information (ESI) available: TPD-MS-CH3OH with the adsorption of CH3OH at 100 °C on ZnO; Arrhenius plots for DME SR reaction over GDZ/γ-Al2O3 catalysts; XRD of the CuZnAlO/γ-Al2O3 and Zn9Ga1O/γ-Al2O3 catalysts before and after stability test; TG of the CuZnAlO/γ-Al2O3 and Zn9Ga1O/γ-Al2O3 catalysts before and after stability test; catalyst characterization of NH3-TPD and TG. See DOI: 10.1039/c6ra07940g |
|
This journal is © The Royal Society of Chemistry 2016 |