DOI:
10.1039/C6RA07844C
(Paper)
RSC Adv., 2016,
6, 74186-74194
A soybean meal-based wood adhesive improved by a diethylene glycol diglycidyl ether: properties and performance
Received
25th March 2016
, Accepted 24th July 2016
First published on 29th July 2016
Abstract
A soybean meal was used to make an effective, biodegradable adhesive for plywood applications using the long-chain crosslinker diethylene glycol diglycidyl ether (DGDE) and the denaturation agent sodium dodecyl sulfate (SDS). The residual rate, functional groups, and morphological properties of the adhesive were investigated to explain the effect of DGDE on the properties and performance of the resultant adhesives. Three-ply plywood was fabricated with the resulting adhesive, and its wet shear strength was measured. The results showed that the water resistance of the adhesive with 8 g of DGDE improved by 16.8% compared to that without DGDE, and the wet shear strength of plywood bonded by the adhesive reached 1.04 MPa, meeting the interior use panel water resistance requirement. This improvement was attributed to the following: ① incorporating DGDE into the adhesive formed a dense cross-linking structure due to chemical reactions between DGDE and active groups on the protein molecules, improving the water resistance of the cured adhesive. ② Adding DGDE decreased the adhesive viscosity and increased the solid content, which increased the amount of the adhesive that penetrated the wood's surface and formed more interlocks. ③ DGDE addition increased the toughness of the adhesive, which greatly improved the impact resistance of the adhesive and decreased the interior force of the resulting plywood. ④ Using DGDE promoted a smooth and homogeneous fracture surface of the cured adhesive, which effectively prevented water intrusion and improved water resistance. The modified adhesive proved practical as a plywood adhesive for industrial use.
1. Introduction
Formaldehyde-based adhesives are widely used in the plywood industry. Many of these adhesives have significant disadvantages: first, their raw materials are unsustainably sourced, as they are mainly derived from non-renewable fossil resources; second, these adhesives and their treated products emit formaldehyde, causing environmental pollution. There have been recently increased restrictions on formaldehyde emissions;1 thus, biomass-derived adhesives have attracted considerable attention as an alternative to petro-based adhesives.2
Soybean, a renewable and abundant industrial raw material, is a promising alternative that has been studied extensively in recent years.3 Researchers have developed and used soybean meal-based adhesive in plywood fabrication.4 However, soybean meal-based adhesives, due to a low availability of reactive groups that can interact with the wood substrate, exhibit limited water resistance.5 The wet shear strength of plywood bonded by the native soybean meal-based adhesive is only approximately 0.3 MPa, which is insufficient to meet the interior use panel bond strength requirement.6
Many attempts have been made to improve the reactivity of the soy protein by altering the protein structure, such as by modification with a denaturation agent. The most common denaturation agents include alkali treatment,7 urea,8 enzymes, sodium dodecyl sulfate,9 and others. Denaturation changes the quaternary, tertiary, and secondary protein structure but rarely alters the primary structure. The stretching of the protein structure allows exposure of buried hydrophobicity groups to limit moisture intrusion. Simultaneously, protein molecules rearrange to provide more reactive sites for hydrogen bonding, therefore improving the water resistance. However, denaturation has resulted in only limited improvement of soybean meal-based adhesive water resistance due to the exposure of polar groups and the low water resistance of hydrogen bonds.10 To prolong the service life of soybean meal-based adhesive, their water resistance and bonding strength must be improved, particularly for products designed for use in harsh environments. Alternative strategies, such as cross-linking modification, synthetic resin modification, nano-scale clay modification, and biomimetic modification have been used to modify the soybean meal-based adhesives to obtain positive results. Cross-linking agents or synthesis resins, such as polyethylenimine epichlorohydrin resin,11 polymeric methane diphenyl diisocyanate,12 polyisocynates,13 epoxy resin,5 phenol-formaldehyde resin, and melamine-formaldehyde resin, greatly improved the water resistance of soybean meal-based adhesive.1 However, the use of petroleum-based resins as cross-linking agents reduced the environmental benefits and renewable advantages of the soy protein due to toxic gas emission and non-renewable materials consumption. Thus, exploration of alternative cross-linking agents to extend the applications of soybean meal-based adhesive remains an important goal.
Diethylene glycol diglycidyl ether (DGDE) is the product of the synthesis of diethylene glycol and epichlorohydrin, which has excellent reactive activity, flexibility, and thermal resistance, curing to form a chain and reticular structure.14 According to a previous report, the synthesis schemes of diethylene glycol diglycidyl ether are illustrated in Scheme 1.14,15 DGDE has two highly reactive epoxide functional groups located at both ends of each molecule.16 DGDE also exhibits good solubility in water that allows it to react with the functional groups on soy proteins. Epoxides, such as bisphenol-A epoxy resin, dimethyl hydantoin polyepoxide, and triglycidylamine, bring a rigid molecular structure into the adhesive, which greatly increases the brittleness of the cured adhesive.17 DGDE has a long-chain structure that can produce an intermolecular toughening effect, which improves the adhesive toughness and water resistance.18
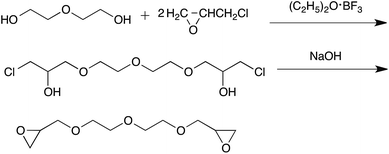 |
| Scheme 1 The synthesis procedure for diethylene glycol diglycidyl ether. | |
In this study, low-cost soybean meal flour (SM) as raw material, diethylene glycol diglycidyl ether (DGDE) as a cross-linker, and sodium dodecyl sulfate (SDS) as a denaturing agent were combined to develop soybean meal-based adhesive samples. The structures and properties of the resultant adhesives were investigated by rheology, infrared spectroscopy, and morphological studies. Three-ply plywood specimens were fabricated to measure the wet shear strength according to Chinese National Standards (GB/T 17657-1999). These results will guide research into further development and improvement of soybean meal-based adhesives.
2. Experimental
2.1. Materials
Soybean meal (43–48% soybean meal, 30–34% polysaccharide, 8–10% moisture, 3–5% fiber, 5–7% ash, and 0.2–0.8% fat) was obtained from Xiang chi Grain and Oil Company in Shang dong Province, China. Diethylene glycol diglycidyl ether (DGDE) and sodium dodecyl sulfate (SDS) were obtained from Tianjin Chemical Reagent Co. Poplar veneer (40 cm × 40 cm × 1.5 cm, 8% moisture content) was obtained from We Nan, Hebei Province, China.
2.2. Preparation of soybean meal-based adhesive
Soybean meal was milled to 200-mesh flour (SM) using a laboratory grinder. Soybean meal (SM) adhesive was prepared by adding soybean meal to water and then stirred for 30 min at 20 °C to form a homogeneous system. Sodium dodecyl sulfate was added to the SM adhesive and mixed for 30 min at 20 °C to create the SDS-fortified adhesive. The PH was maintained under neutral conditions. The relative humidity of the mixing environment was stabilized at approximately 45%. To create the DGDE-fortified adhesive, different amounts of DGDE were dissolved in the water and mixed for 10 min before the soybean meal and the SDS were added to the solution, followed by pH adjustment to 6.8–7.2. Then, the soybean meal and sodium dodecyl sulfate were added and stirred for 10 min at 20 °C to develop the soybean meal-based adhesive. The adhesive formulations are shown in Table 1.
Table 1 Different adhesive formulations
Sample |
Adhesive formulation |
a (SM adhesive) |
Soybean meal flour (28 g); deionized water (72 g) |
b (SM/SDS adhesive) |
Soybean meal flour (28 g); deionized water (72 g); sodium dodecyl sulfate (1.5 g) |
c (SM/PAM/SDS adhesive-2 adhesive) |
Soybean meal flour (28 g); deionized water (72 g); sodium dodecyl sulfate (1.5 g); DGDE (2 g) |
d (SM/PAM/SDS/DGDE-5 adhesive) |
Soybean meal flour (28 g); deionized water (72 g); sodium dodecyl sulfate (1.5 g); DGDE (5 g) |
e (SM/PAM/SDS/DGDE-8 adhesive) |
Soybean meal flour (28 g); deionized water (72 g); sodium dodecyl sulfate (1.5 g); DGDE (8 g) |
f (SM/PAM/SDS/DGDE-11 adhesive) |
Soybean meal flour (28 g); deionized water (72 g); sodium dodecyl sulfate (1.5 g); DGDE (11 g) |
2.3. Solid content measurement
The solid contents of adhesive were determined using an oven-drying method according to the description in China National Standards (GB/T 17657-1999). Approximately 3 g (weight α) of the adhesive was placed into an oven and dried at 105 °C for multiple hours until a constant weight (weight β) was obtained. The value of the solid content was calculated using the following equation. The value of the solid content was calculated from the average of three parallel samples. |
 | (1) |
2.4. Dynamic viscoelastic measurement
The viscosity of the fresh adhesives was determined using a rheometer with a parallel plate fixture (20 mm diameter). The distance was set to 1 mm for all of the measurements. The experiments were conducted under a steady shear flow at 25 °C. The shear rates ranged from 0.1 to 300 s−1 in 10 s−1 increments. All of the measurements were conducted in triplicate, and the average value was reported.
2.5. Moisture uptake measurement and toughness measurement of cured adhesives
The moisture uptake measurement of the cured adhesive was determined by gravimetric analysis. Six pieces of cured adhesive were placed into a constant temperature and humidity chamber set to 80% relative humidity and 50 °C. The weights of the adhesive chunks were measured every two hours until a constant value was obtained. The moisture uptake value was calculated using the following equation (α: the adhesive before treatment; β: the adhesive after treatment) |
 | (2) |
To observe the surface morphology, prepared adhesives were uniformly coated on glass slides and then placed in an oven at 120 ± 2 °C for 2 h. After the adhesives were cured, coated glass slides were placed in a desiccator for 30 min. The cooled glass slides were photographed using a Digital Single Lens Reflex.
2.6. Preparation of plywood samples
Three-ply plywood samples were prepared under the following conditions: 180 g m−2 glue spreading for a single surface, 120 °C hot pressing temperature, and 1.0 MPa hot pressing pressure. After hot pressing, the plywood samples were stored under ambient conditions for at least 12 h before testing.
2.7. Wet shear strength measurement
The shear strength requirement of the interior use plywood (Type II plywood) was determined using a wet shear strength test in accordance with the description in China National Standards (GB/T 17657-1999). Twelve plywood specimens (2.5 cm × 10 cm) were cut from two plywood panels, submerged into water at 63 ± 2 °C for 3 h, and then dried at room temperature for 10 min before a tension test. The wet shear strength was calculated by the following equation. |
 | (3) |
2.8. Residual rate measurement
The adhesive samples were placed in an oven at 120 ± 2 °C until a constant weight (M) was obtained. The cured adhesives were soaked in tap water for 24 h at ambient temperature and then oven-dried at 105 ± 2 °C for 5 h until a constant weight was obtained (m). The residual rate is defined as m divided by M, as shown in eqn (4). The average value of the residual rate was calculated from six parallel samples. |
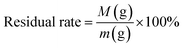 | (4) |
2.9. Fourier transform infrared (FTIR) spectroscopy
The adhesives were cured in an oven at 120 ± 2 °C until a constant weight was obtained and then ground into a powder. FTIR spectra of the cured adhesives were recorded on a Nicolet 7600 spectrometer (Nicolet Instrument Corporation, Madison, WI) from 500 to 4000 cm−1 with a 4 cm−1 resolution using 32 scans.
2.10. X-ray diffraction (XRD)
The adhesives were placed in an oven at 120 ± 2 °C to cure for 2 h and then ground into 200-mesh powder (0.075 mm) by a ceramic mortar. Powder X-ray diffraction (XRD) patterns were recorded on an XRD diffractometer (D8 ADVANCE, BRUKER, German) using a cobalt source and 0.2 theta scan ranging from 5° to 60° at 45 kV and 30 mA. The crystallinity of the powdered samples was calculated using MDI Jade 6.0.
2.11. Scanning electron microscopy (SEM)
Adhesive samples were placed in an oven at 120 ± 2 °C until a constant weight was reached. To begin testing, the samples were placed on an aluminum stub, and a Hitachi S-3400N (Hitachi Science System, Ibaraki, Japan) scanning electron microscope was used to observe the fractured surface of the adhesive. The surface was sputter coated with gold prior to examination under the microscope.
2.12. Thermal stability measurement
The different samples were placed in an oven at 120 ± 2 °C until a constant weight was obtained and then ground into a powder. The thermal stability of cured adhesive samples was tested using a thermogravimetric analyzer (TA Q50, WATERS Company, USA). Approximately 5 mg of powdered sample was weighed in a platinum cup and scanned from room temperature to 600 °C at a heating rate of 10 °C min−1 in a nitrogen environment while recording the weight change.
3. Results and discussion
3.1. Solid content measurement
The solid content of soybean meal-based adhesives affects performance during hot pressing.19 When an adhesive has a low solid content, a lot of water is brought into the plywood and needs to be removed during the hot press process, wasting energy. Furthermore, water vaporization increases the internal stress and can damage bonds and reduce the dimensional stability of the plywood. Therefore, a high solid content improves the water resistance of the adhesive. Fig. 1 shows the solid contents of the different adhesive samples. The solid content of the SM adhesive was 30.41% and presented a very high viscosity, which caused a flow issue. A further increase in the solid content of the adhesive was determined by the SDS additive in the SM adhesive system.20 When adding SDS in the adhesive, the solid content of the adhesive was increased from 30.41% to 33.71%. The solid content of the adhesive gradually increased from 33.71 to 40.48% when DGDE addition was increased from 2 to 11 g. This increase was expected because the addition of SDS and DGDE was equivalent to increasing the mass content of the adhesive. According to previous research, the elevated solid content (≥35%) could promote interlocking between the wood and adhesive. It could also reduce water evaporation to enhance the adhesion.21 As DGDE augmentation rose to 8 g, the solid content of the adhesive increased significantly (28.7%) over the SM adhesive, reaching 39.13% (≥35%), a level at which the plywood was suitable for industrial application.
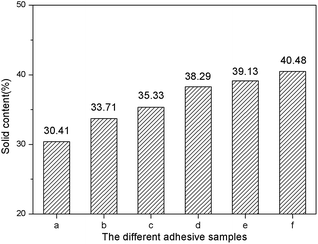 |
| Fig. 1 The solid content of different adhesive samples: a (SM adhesive), b (SM/SDS adhesive), c (SM/SDS/DGDE-2 adhesive), d (SM/SDS/DGDE-5 adhesive), e (SM/SDS/DGDE-8 adhesive), f (SM/SDS/DGDE-11 adhesive). | |
3.2. Dynamic viscoelastic measurement
An appropriate viscosity range is required for adherence to industry standards in wood composites. An adhesive with a viscosity that is too high does not allow mechanical bonding between veneers and the adhesive. An adhesive with a low viscosity allows easy handling and good flow across the wood surface, but if the viscosity is too low, then there can be an over-penetration into the wood veneer, resulting in bond breaking.
The initial apparent viscosity was recorded and presented in Table 2. The viscosity of adhesive a was 40
530 mPa s, causing an adhesive flow and distribution issue. At high viscosity, the adhesive had difficulty penetrating into the wood surface and formed a mechanical inter-lock between the veneers and adhesive during hot pressing, thus resulting in low bond strength. After adding SDS, the viscosity of the adhesives was increased to 96
240 mPa s. Addition of SDS caused a swelling and unfolding of the protein molecules, which decreased the distance between protein molecules and thus increased the apparent viscosity of the adhesive. After incorporating DGDE, the apparent viscosity of adhesive a decreased by 29.2% to 68
170 mPa s. When the content of DGDE reached 11 g, the viscosity of the DGDE adhesive further decreased to 21
960 mPa s. DGDE dispersed well and embedded between the soy protein molecules, reducing the frictional resistance within the liquid and resulted in a low viscosity of the adhesive.
Table 2 The apparent viscosity of the adhesive samples: a (SM adhesive), b (SM/SDS adhesive), c (SM/SDS/DGDE-2 adhesive), d (SM/SDS/DGDE-5 adhesive), e (SM/SDS/DGDE-8 adhesive), f (SM/SDS/DGDE-11 adhesive)
Sample |
a |
b |
c |
d |
e |
f |
Initial viscosity (mPa s) |
40 530 |
96 240 |
68 170 |
51 140 |
34 570 |
21 960 |
3.3. Water resistance measurement
Fig. 2 and 3 show the residual rate of the cured adhesive and the wet shear strength of plywood bonded with different adhesives. For the SM adhesive, the wet shear strength of the resultant plywood specimens was 0.37 MPa, which is much lower than the interior use panel bond strength requirement. The residual rate of SM adhesive was also the lowest, 62.6%, indicating that the SM adhesive had the lowest cross-linking structure among the tested adhesives. During the curing process, the bond properties of the SM adhesive depended on the protein molecules twining with each other and the interaction of functional groups with wood, such as van der Waals force, hydrogen bonding, and hydrophobic interactions. However, this type of bond was easily broken by moisture, which resulted in the low water resistance.22 The wet shear strength of the plywood increased by 29.7%, from 0.37 to 0.48 MPa, upon addition of 1.5 g of SDS. SDS was a superior dispersant and denaturing agent. Its addition into the soybean meal-based adhesive caused an unfolding of the soy protein molecules and exposed additional hydrophobic side chains. Together, the contact area was increased, strengthening the interaction between the soybean meal and wood during the curing process and further improving the water resistance of the adhesive.23 Additionally, the unfolded protein molecule rearranged during the curing process and formed a more crystalline structure, which improved the residual rate of the adhesive from 62.6 to 65.5% compared with the SM adhesive.20
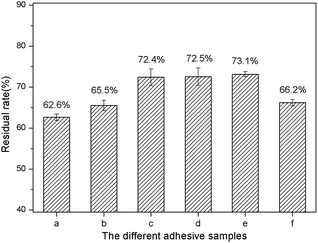 |
| Fig. 2 The residual rate of the different adhesive samples: a (SM adhesive), b (SM/SDS adhesive), c (SM/SDS/DGDE-2 adhesive), d (SM/SDS/DGDE-5 adhesive), e (SM/SDS/DGDE-8 adhesive), f (SM/SDS/DGDE-11 adhesive). | |
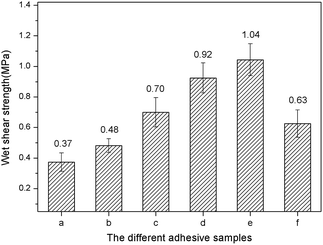 |
| Fig. 3 Wet shear strength of plywood panels with the different adhesives: a (SM adhesive), b (SM/SDS adhesive), c (SM/SDS/DGDE-2 adhesive), d (SM/SDS/DGDE-5 adhesive), e (SM/SDS/DGDE-8 adhesive), f (SM/SDS/DGDE-11 adhesive). | |
With the addition of DGDE, the wet shear strength of plywood bonded by the different adhesive formulations increased gradually. The wet shear strength increased by 45.8% to 0.70 MPa upon addition of 2 g of DGDE, which met the interior use panel bond strength requirement. The residual rate of the adhesive was improved by 15.7% with DGDE addition of 2%. This may be due to two reasons: first, the introduction of DGDE enhanced adhesive flowability, promoting formation of interlocking to improve the water resistance of the adhesive. Second, DGDE could have improved the water resistance by a reaction between the highly reactive epoxy groups at opposite ends of the resin molecule and reactive groups in the soy protein. After further increasing the DGDE addition to 5 g, the wet shear strength was improved to 0.92 MPa, an increase of 149% compared to adhesive a. At 8 g DGDE, the wet shear strength was 1.04 MPa, and the residual rate of the adhesive was the highest at 73.1%, indicating additional cross-linking in the adhesive. A higher concentration of DGDE resulted in increased molecular weight and cross-linking density with superior water resistance.
However, addition of excess DGDE did not lead to further improvements in cross-linking. When the DGDE content was increased to 11 g, the bond strength decreased to 0.63 MPa, which is less than the interior use panel bond strength requirement (0.70 MPa). This was partly due to the depressed physical viscosity of the adhesive, which resulted in over penetration into the veneer and low bond strength. Additionally, excess DGDE may have remained in the adhesive, affecting the formation of cross-linking structures and a reduction in strength. The residual rate decreased 9.4% when DGDE content was increased to 11 g. The residual rate of the adhesive further decreased by 8.6 to 66.2% when compared with adhesive c. This is because the reactive groups on the soy protein reacted completely, and the excess DGDE dissolved into water. The cross-linking network structure for SM/SDS/DGDE is illustrated in Scheme 2.
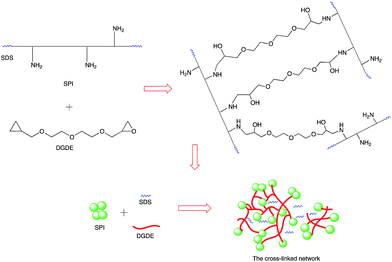 |
| Scheme 2 The cross-linking network structure of modified soybean meal based adhesive. | |
3.4. FTIR spectroscopic analysis
To characterize the possible interactions between the different adhesives, FTIR spectroscopy was used as shown in Fig. 4. A peak observed at approximately 3278 cm−1 was assigned to the free bound O–H and N–H bending vibrations, which formed hydrogen bonds with the carbonyl group of the peptide linkage in the protein.24 A typical soy protein spectrum shows three major peaks at 1660, 1533, and 1234 cm−1.25 These peaks were assigned to C
O stretching (amide I), N–H deformation (amide II), and C–N stretching and N–H vibration (amide III) respectively.26 The bands corresponding to the stretching vibration of COO– were located at 1395 cm−1.21
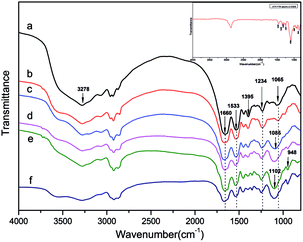 |
| Fig. 4 FTIR spectra of different adhesive samples: a (SM adhesive), b (SM/SDS adhesive), c (SM/SDS/DGDE-2 adhesive), d (SM/SDS/DGDE-5 adhesive), e (SM/SDS/DGDE-8 adhesive), f (SM/SDS/DGDE-11 adhesive). | |
With DGDE addition increasing from 0 to 11 g in the adhesive formulation, the peak of amides II and III (1536 and 1395 cm−1) were gradually weakened, which was due to the reaction between the epoxy groups of DGDE and the N–H groups on the soy protein molecule. After adding DGDE, amide I, II, and III shifted from 1660 to 1665 cm−1, 1533 to 1550 cm−1, and 1234 to 1248 cm−1 respectively. The blue shift in the spectrum of the adhesive with DGDE, indicated that the soy protein molecule had denser structure than the SM adhesive. This dense structure was caused by the chemical reaction between the soy protein molecule and DGDE, which increased the cross-linking degree of the adhesive. The main difference between the spectrum of the soy protein adhesive with or without DGDE was the absorption at 1065 cm−1, which was assigned C–O stretching.27 In the spectra of c and d, the peak at 1065 cm−1 gradually increased, which suggested the homogeneous dispersion of DGDE in the adhesive system. DGDE could have reacted with the soy protein to form a solid network to increase the cross-linking degree and improve water resistance of the adhesive. One would expect an epoxy group characteristic peak at 846 cm−1, but as shown in Fig. 4, there was no peak at 846 cm−1 in the spectrum of the SM/SDS/DGDE-2 and SM/SDS/DGDE-5 adhesive. This phenomenon suggests that the epoxy group of DGDE reacted with the active hydrogen on the –NH2 groups in the protein molecules during the curing process in a ring-opening reaction (the likely specific reaction discussed in Scheme 3). With increased DGDE levels, the peak at 846 cm−1 was observed again, suggesting an excess of DGDE in the formulation of the SM/SDS/DGDE-8 and SM/SDS/DGDE-11 adhesives. Due to the water solubility of the DGDE, the water resistance of the adhesive was reduced, which caused the low wet shear strength of the resulting plywood.
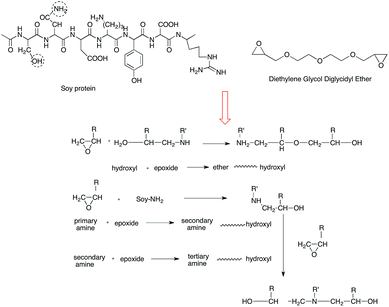 |
| Scheme 3 Illustration of the cross-linking reactions between DGDE and soy protein molecule. | |
3.5. X-ray diffraction analysis
X-ray diffraction patterns of the various cured adhesives are presented in Fig. 5, and the crystallinity of the different adhesive samples is calculated and presented in Table 3. As seen in Fig. 5, the SM adhesive exhibited two strong characteristic peaks at 2θ values of approximately 9° and 20°, which belong to the α-helix and β-sheet structure of protein molecules.28 However, adding DGDE in the disappearance of the crystallization peak at 9°, indicating that a chemical reaction had occurred between the DGDE and soy protein molecules.29 This confirms the results of the FTIR analysis discussed previously.
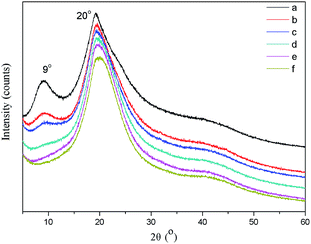 |
| Fig. 5 The X-ray diffraction patterns of different adhesive samples: the crystallinity of different cured adhesive samples: a (SM adhesive), b (SM/SDS adhesive), c (SM/SDS/DGDE-2 adhesive), d (SM/SDS/DGDE-5 adhesive), e (SM/SDS/DGDE-8 adhesive), f (SM/SDS/DGDE-11 adhesive). | |
Table 3 The crystallinity of different cured adhesive samples: a (SM adhesive), b (SM/SDS adhesive), c (SM/SDS/DGDE-2 adhesive), d (SM/SDS/DGDE-5 adhesive), e (SM/SDS/DGDE-8 adhesive), f (SM/SDS/DGDE-11 adhesive)
Adhesive sample |
a |
b |
c |
d |
e |
f |
The crystallinity (%) |
14.26 |
14.40 |
10.06 |
8.69 |
8.30 |
7.91 |
As shown in Table 3, crystallinity increased from 14.26% to 14.40% after adding SDS, which could be attributed to the unfolded protein molecules rearranging and forming a more crystalline domain during the hot press process.20 This elevated crystalline domain improved the water resistance of the adhesive. When introducing the DGDE, the crystallinity decreased to 10.06%, and it further decreased to 8.30% in adhesive f. Generally, a high cross-linking of the adhesive leads to decrease in crystallinity.30 From this result, it could be concluded that the cross-linking reaction between DGDE and the soy protein has increased the cross-linking density of the cured adhesive, thus decreasing the crystallinity of the adhesive (as shown in Fig. 5).
When Fig. 2 and 5 were compared, a similar correlation was shown between adhesive crystallinity and residual rate. The SM adhesive had a lowest residual rate of 62.6% and a high crystallinity of 14.20%. With the addition of DGDE, the residual rate of the adhesive was improved due to the reaction between DGDE and soy protein molecules, which increased the cross-linking degree and reduced the crystallinity of the cured adhesive. Further addition of DGDE increased the cross-linking degree of the adhesive system and led to a crystallinity reduction and thus also increased the residual rate of the cured adhesive.
3.6. Toughness measurements of cured adhesives
Fig. 6 illustrates the toughness of the different cured adhesives. The SM adhesive was more brittle and showed a mass of small cracks upon fracture. After introduction of the SDS, the cured adhesive showed few cracks and became more compact, increasing the brittleness. This was consistent with the SDS causing rearrangement of the protein molecule during the curing process, which increased its crystallinity and led to increased brittleness.31 With the addition of the DGDE, the fracture surface became more compact and homogeneous. The inherent brittleness of the cured adhesive decreased, which improved toughness and the impact resistance strength of the adhesive. When bonding plywood, an adhesive with elevated toughness will reduce the interior force and present a high water resistance.
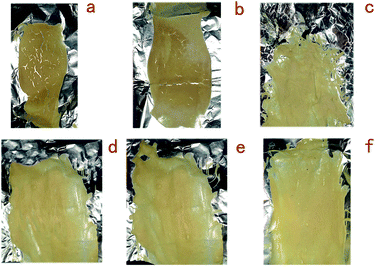 |
| Fig. 6 The toughness measurement of the cured different adhesive samples: a (SM adhesive), b (SM/SDS adhesive), c (SM/SDS/DGDE-2 adhesive), d (SM/SDS/DGDE-5 adhesive), e (SM/PAM/SDS/DGDE-8 adhesive), f (SM/SDS/DGDE-11 adhesive). | |
3.7. SEM analysis
Scanning electron micrographs of the fracture surface of the different cured adhesives are presented in Fig. 7. Irregularity of a large area with a large number of holes was observed on the fracture surface of the SM adhesives. The entire fracture surface appeared cluttered and disordered due to the evaporation of moisture from the adhesive during the hot press process. Therefore, the water resistance and the bond strength of the plywood were relatively low.29
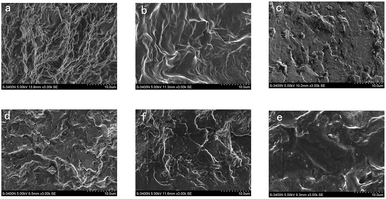 |
| Fig. 7 The fracture surface micrograph of different cured adhesive samples: a (SM adhesive), b (SM/SDS adhesive), c (SM/PAM/SDS/DGDE-2 adhesive), d (SM/SDS/DGDE-5 adhesive), e (SM/SDS/DGDE-8 adhesive), f (SM/SDS/DGDE-11 adhesive). | |
With the incorporation of SDS, fewer holes were observed on the fracture surface; indicating that the addition of SDS reduced the water gasification in the adhesive. This phenomenon may be due to the unfolded protein molecules rearranging to form a more crystalline domain, resulting in increased density. After introduction of 2 g DGDE, the cross-linking structure destroyed the crystalline domain, which caused the surface to become rougher. Further addition of DGDE from 2 to 5 g, resulted in a reaction that increased the cross-linking structure and improved the smoothness and compaction of the fracture surface of the cured adhesives. This could be caused by the reaction between the DGDE and soy proteins to form a network structure, inhibiting moisture intrusion and improving water resistance of the adhesive. Incorporation of 11 g of DGDE in adhesive f resulted in a more homogeneous fracture surface with fewer holes due to residual DGDE in the adhesive. The DGDE filled holes and increased the cross-linking density by strongly interacting with the soy proteins. As mentioned above, DGDE also improved the wet shear strength. Table 4 shows the moisture uptake of different cured adhesive samples. After adding DGDE, the moisture uptake of the cured adhesive gradually decreased, suggesting that the adhesive containing DGDE efficiently prevented moisture intrusion and formed the cross-linking network to improve water resistance.32 Comparison of adhesives a and f showed that the moisture uptake decreased from 6.1% to 2.4%. As seen in Fig. 7 and Table 4, the decrease in moisture uptake created a compact surface that improved the water resistance of the cured adhesive. The smooth surface of the cured adhesive with DGDE was likely due to increased adhesive flowability and toughness. DGDE cross-linked soy protein molecules formed a network system, reinforcing the structure and relieving the inherent brittleness of the adhesive. These findings were consistent with the results of the bonding strength and SEM analysis.
Table 4 Moisture uptake of different cured adhesive samples: a (SM adhesive), b (SM/SDS adhesive), c (SM/SDS/DGDE-2 adhesive), d (SM/SDS/DGDE-5 adhesive), e (SM/SDS/DGDE-8 adhesive), f (SM/SDS/DGDE-11 adhesive)
Sample |
a |
b |
c |
d |
e |
f |
Equilibrium moisture uptake (%) |
6.1 ± 0.3 |
5.1 ± 0.2 |
4.8 ± 0.05 |
4.5 ± 0.1 |
4.2 ± 0.2 |
2.4 ± 0.4 |
3.8. Thermal stability of adhesives
The thermal stabilities of the soy protein adhesive and modified soy protein adhesive were characterized using TGA analysis. Fig. 8a and b shows the TG and DTG curves of different adhesive samples. For a better view of the weight loss, TG curves were transformed into their first derivative (weight loss/temperature) curves (DTG) using TA software Universal Analysis (version 2000). The thermal degradation of the adhesive could be divided into three stages. The first stage (I), observed between 70 °C and 200 °C, was primarily associated with the evaporation of residual moisture. In this stage, adhesive a showed no degradation of the soy protein and a small weight loss ratio. The second and third stages were associated with the thermal degradation of the components in the adhesives. The second stage (II) was the initial degradation stage from 200 °C to 280 °C, which mainly was considered as the weight loss due to small molecule subject degradation and the break of some unstable chemical bonds.33 The third stage (III), over a temperature range of 280 to 360 °C, was the skeleton structure degradation stage, such as the breakage of C–C, C–O, and C–N.
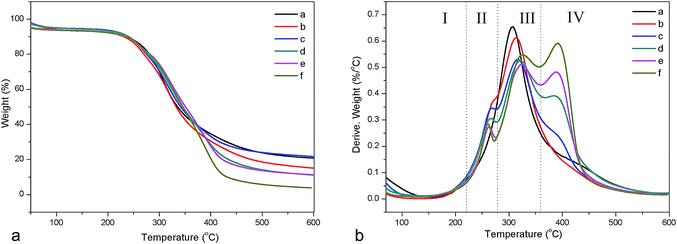 |
| Fig. 8 (a) Thermogravimetric curves of the different adhesive samples: a (SM adhesive), b (SM/SDS adhesive), c (SM/SDS/DGDE-2 adhesive), d (SM/SDS/DGDE-5 adhesive), e (SM/SDS/DGDE-8 adhesive), f (SM/SDS/DGDE-11 adhesive). (b) Derivate thermogravimetric (DTG) curves for soy protein adhesives: a (SM adhesive), b (SM/SDS adhesive), c (SM/SDS/DGDE-2 adhesive), d (SM/SDS/DGDE-5 adhesive), e (SM/SDS/DGDE-8 adhesive), f (SM/SDS/DGDE-11 adhesive). | |
Comparing adhesives a and b, adhesive b showed a new peak at stage (II), which was attributed to an increase in the random state and in the small molecular substances in the soy protein caused by the denaturing effect of SDS.34 After using DGDE in the adhesive formulation, the peaks of the DTG curve in the second stage were stronger than those in the SM adhesive. The peak at stage (III) decreased and moved to a high temperature, and a new peak was observed at approximately 380 °C, indicating that there was a reaction between the DGDE and soy protein molecules. In this process, the cross-linked protein molecular network played a major role in the adhesive system, improving the water resistance of the adhesive. Upon increasing the DGDE further, the peak at stage (II) decreased, and the peak at approximately 380 °C increased, suggesting the thermostability of the adhesive increased with the DGDE addition. This elevated thermostability was due to the cross-linked structure formation, resulting in improved adhesive water resistance.
4. Conclusions
The DGDE, as a long-chain cross-linker, effectively improved the properties and performance of soybean meal-based adhesive. The wet shear strength of plywood bonded by the adhesive with 8 g of DGDE reached 1.04 MPa, meeting the interior use plywood water resistance requirement. This improvement was attributed to the following reasons: (1) the formation of a cross-linking network from the reaction between the epoxy groups and the protein functional groups; (2) incorporation of DGDE increased the toughness, resulting in an interior force reduction; (3) creation of a smooth and homogenous fracture surface that prevented moisture intrusion; (4) DGDE-dependent increase in the adhesive solid content to 39.13% and decrease in the adhesive viscosity to 34
570 mPa s. These improvements made the bonded plywood suitable for industrial application.
Acknowledgements
The authors are grateful for financial support from Beijing Natural Science Foundation (2151003) and the Special Fund for Forestry Research in the Public Interest (Project 201404501).
Notes and references
- H. Lei, G. Du, Z. Wu, X. Xi and Z. Dong, Int. J. Adhes. Adhes., 2014, 50, 199–203 CrossRef CAS.
- N. Chen, Q. Lin, J. Rao and Q. Zeng, Ind. Crops Prod., 2013, 50, 44–49 CrossRef CAS.
- G. Qi, N. Li, D. Wang and X. S. Sun, J. Am. Oil Chem. Soc., 2011, 89, 301–312 CrossRef.
- X. Mo and X. S. Sun, J. Adhes. Sci. Technol., 2013, 27, 2014–2026 CrossRef CAS.
- N. Chen, Q. Lin, Q. Zeng and J. Rao, Ind. Crops Prod., 2013, 51, 267–273 CrossRef CAS.
- E. Lépine, B. Riedl, X.-M. Wang, A. Pizzi, L. Delmotte, J.-M. Hardy and M. J. R. Da Cruz, Int. J. Adhes. Adhes., 2015, 63, 74–78 CrossRef.
- G. Wang and H. Chen, Ind. Crops Prod., 2014, 53, 93–101 CrossRef.
- Q. Gao, J. Li, S. Q. Shi, K. Liang and X. Zhang, BioResources, 2012, 7, 5622–5633 Search PubMed.
- W. Huang and X. Sun, J. Am. Oil Chem. Soc., 2000, 77, 705–708 CrossRef CAS.
- G. A. Amaral-Labat, A. Pizzi, A. R. Goncalves, A. Celzard, S. Rigolet and G. J. M. Rocha, J. Appl. Polym. Sci., 2008, 108, 624–632 CrossRef CAS.
- K. Gu and K. Li, J. Am. Oil Chem. Soc., 2011, 88, 673–679 CrossRef CAS.
- Y. Zhang, W. Zhu, Y. Lu, Z. Gao and J. Gu, Ind. Crops Prod., 2014, 57, 35–42 CrossRef CAS.
- R. Deng, Y. Chen, P. Chen, L. Zhang and B. Liao, Polymer, 2006, 91, 2189–2197 CAS.
- F. Liu, K. Guo and J. Yuan, High Perform. Polym., 2013, 26, 326–334 CrossRef.
- M. A. Caenahan and M. W. Grinstaff, J. Am. Chem. Soc., 2001, 123, 2905–2906 CrossRef.
- P. Krafft, P. Gilbeau, B. Gosselin and S. Claessens, US Pat, 8 415 509, 2013.
- G. Vargas, J. L. Acevedo, J. López and J. Romero, Mater. Lett., 2008, 62, 3656–3658 CrossRef CAS.
- N. Vasylieva, B. Barnych, A. Meiller, C. Maucler, L. Pollegioni, J.-S. Lin, D. Barbier and S. Marinesco, Biosens. Bioelectron., 2011, 26, 3993–4000 CrossRef CAS PubMed.
- H. Li, C. Li, Q. Gao, S. Zhang and J. Li, Ind. Crops Prod., 2014, 59, 35–40 CrossRef CAS.
- J. Luo, C. Li, X. Li, J. Luo, Q. Gao and J. Li, RSC Adv., 2015, 5, 62957–62965 RSC.
- Q. Gao, S. Q. Shi, J. Li, K. Liang and X. Zhang, BioResources, 2012, 7, 0946–0956 CAS.
- C. R. Frihart and M. J. Birkeland, J. Am. Chem. Soc., 2014, 1178, 167–192 CAS.
- H. Xu, J. Luo, Q. Gao, S. Zhang and J. Li, BioResources, 2014, 9, 4667–4678 Search PubMed.
- R. M.
D. Soares, F. F. Scremin and V. Soldi, Macromol. Symp., 2005, 229, 258–265 CrossRef CAS.
- J. T. Kim and A. N. Netravali, J. Agric. Food Chem., 2010, 58, 5400–5407 CrossRef CAS PubMed.
- T. Ghosh Dastidar and A. N. Netravali, Green Chem., 2013, 15, 3243 RSC.
- R. James, X. Geng and K. Li, Wood Sci. Technol., 2004, 36, 186–194 Search PubMed.
- J. Chen, X. Chen, Q. Zhu, F. Chen, X. Zhao and Q. Ao, J. Sci. Food Agric., 2013, 93, 1687–1691 CrossRef CAS PubMed.
- L. Chen and M. Subirade, Biomacromolecules, 2009, 10, 3327–3334 CrossRef CAS PubMed.
- M. Rubinstein and R. Colby, Polymers Physics, Oxford, 2003 Search PubMed.
- J. Luo, J. Luo, C. Yuan, W. Zhang, J. Li, Q. Gao and H. Chen, RSC Adv., 2015, 5, 1000849–1100855 Search PubMed.
- W. P. F. Neto, H. A. Silvério, N. O. Dantas and D. Pasquini, Ind. Crops Prod., 2013, 42, 480–488 CrossRef.
- R. Kumar, V. Choudhary, S. Mishra and I. K. Varma, J. Adhes. Sci. Technol., 2004, 18, 261–273 CrossRef CAS.
- V. Schmidt, C. Giacomelli and V. Soldi, Polym. Degrad. Stab., 2005, 87, 25–31 CrossRef CAS.
|
This journal is © The Royal Society of Chemistry 2016 |