DOI:
10.1039/C6RA07594K
(Paper)
RSC Adv., 2016,
6, 47249-47257
A novel method to modify the color of VO2-based thermochromic smart films by solution-processed VO2@SiO2@Au core–shell nanoparticles†
Received
23rd March 2016
, Accepted 25th April 2016
First published on 29th April 2016
Abstract
In this study, the VO2@SiO2@Au core–shell nanoparticles were synthesized by a solution process to tailor the color of VO2 films on the basis of surface plasmon resonance (SPR). The SiO2 interlayer not only provided more hydroxyl bonding for the silane coupling agent, which was conducive to metal deposition, but also significantly improved the anti-oxidation ability of VO2. We optimized the thickness of silica to maintain the silica layer uniform and thin (14 nm), which would impact on the optical properties. The VO2@SiO2@Au films with various Au particle sizes displayed a series of colors from brick red to purple and blue while maintaining the solar modulation ability (the maximum ΔTsol is up to 7.52%). Moreover, the phase transition temperature decreased by about 6 °C without doping due to electron injection from Au to VO2. This study proposed a novel and facile method to tune the color of VO2-based thermochromic smart films while improving the anti-oxidation ability and decreasing the phase transition temperature. It may constitute a great advance in the development of thermochromic smart films.
Introduction
Vanadium dioxide (VO2), with a fascinating thermochromic property, was discovered by Morin in 1959.1 VO2 undergoes a reversible metal–semiconductor transition at a critical temperature Tt (68 °C for bulk VO2) wherein there is transparent to near-infrared light (NIR) at temperatures below Tt but translucent to NIR light above Tt. Over the previous decades, it had been considered as one of the most competitive candidate materials for intelligent glass. However, related materials have not been commercialized because of the problems of high phase transition temperature, low visible transmittance (Tlum), limited solar energy modulation ability (ΔTsol), and unpleasant color. The transition temperature needs to be around room temperature (25 °C), which can be achieved by introducing dopants.2–6 For improving the luminous transmittance and the solar modulation ability, strategies, including doping,4 multilayer-stack design,7 and the formation of composite films have been employed.8
The unpleasant color of the VO2 film is also one of the matters needed to be addressed. Because the bandgap between V3d and O2p is 2.5 eV (corresponding to the visible absorption edge of 496 nm), VO2 films can absorb visible light at wavelengths below 500 nm and present a corresponding complementary color (brown-yellow).9 However, in real-world applications, the commonly used color of windows in the most modern buildings is blue or similar, which is most likely to be accepted by people. Moreover, it would satisfy different needs if the color of the VO2 films could be tailored. The issue of the color of VO2 films has been taken seriously and some solutions have been reported in recent years. Regulating the bandgap between V3d and O2p by doping with F9 or Mg10 can change the color of VO2 films. The doped VO2 film exhibits a modified comfortable color while still retaining excellent solar modulation ability. However, the color can only be tuned in a narrow spectral region because doping elements are limited.The core–shell VO2@TiO2 nanorod can also modify the color by changing the thickness of the TiO2 shell in which scttering plays an important role in the light-matter interactions. Simultaneously, the anti-oxidation ability was improved. However, the color of smaller VO2 particles (∼<50 nm) cannot be effectively modified by TiO2 coating.11 On the other hand, a great deal of progress in surface plasmon resonance (SPR) based on the VO2 matrix has been made to change the color of VO2 films. It is well known that noble metal nanoparticles present plentiful colors due to selective light absorption resulting from SPR, which usually occurs in the visible region.12,13 The peak wavelength (called λSPR in the following) depends on the size and shape of metal particles, and the dielectric properties of the matrix around them.14,15 The VO2–metal composite films with different colors were prepared by controlling the size of the metal particle. Recently, magnetron sputtering16–18 and chemical vapor deposition (CVD)19–21 were utilized to prepare VO2/Au and VO2/Ag composite films. However, whether by magnetron sputtering or CVD, harsh conditions are needed. This is disadvantageous to practical fabrication of large-scale and cost-effective smart films.
In this study, we propose a novel method based on SPR to tailor the color of VO2 films from yellow-brown to red and blue, no matter what size the VO2 nanoparticles are. At present, according to the ref. 22 and the previous study in our lab, the difficulty of producing VO2@Au core–shell particles via a solution process has been demonstrated. In contrast, the syntheses of VO2@SiO2 (ref. 23) and SiO2@Au24,25 nanoparticles have been reported. Thus, a silica layer was introduced to form the VO2@SiO2@Au structure. The silica interlayer will not only provide more hydroxyl bonding for the silane coupling agent, which is conducive to metal deposition,26 but also significantly improve the anti-oxidation ability of VO2.23 The VO2@SiO2@Au particles with varied gold sizes were synthesized by controlling the amount of gold salt in the growth process. After mixing these nanoparticles with polyvinyl alcohol (PVA) solution, we obtained composite thin films with the colors of brick red, purple and blue while maintaining the solar modulation ability (the maximum ΔTsol is up to 7.52%), decreasing the phase transition temperature and improving the anti-oxidation ability. Throughout the experiment, there was no complicated equipment, no high temperature, and no high vacuum. This method makes an important breakthrough in color modulation of VO2-based thermochromic smart films.
Experimental
The synthetic route of VO2@SiO2@Au is shown in Scheme 1. More details are given in the following.
 |
| Scheme 1 Synthesis procedure for VO2@SiO2@Au. | |
Preparation of VO2 nanoparticles
The VO2 nanoparticles were obtained by the typical process as follows: 0.2 M NH4HCO3 aqueous solution was dropped into 0.1 M VOSO4 aqueous solution. The grey-white precipitate was centrifuged and washed with copious amounts of water. Subsequently, the precipitation was heated in an autoclave at 280 °C for 24 h to acquire VO2 nanoparticles. For more detail, please refer to ref. 27.
Preparation of VO2@SiO2 nanoparticles
First, the VO2 nanoparticles were dispersed in deionized water with ultrasonic-assisted treatment. Then, the VO2 aqueous solution and the PVP aqueous solution were mixed under vigorous stirring for 24 h at room temperature to ensure that PVP was adsorbed onto VO2.28 The PVP-functionalized VO2 nanoparticles were transferred to 30 mL ethanol and stirred continuously. 10 mL ethanol solution containing the appropriate amount of tetraethoxysilane (TEOS) and 10 mL dilute aqueous solution of ammonia were added to the VO2 solution sequentially. Finally, the mixture was stirred for a period of time at room temperature. When the reaction was completed, the samples were washed with deionized water and ethanol several times, and dispersed in ethanol for the next reaction step.23
Preparation of VO2@SiO2@Au
Preparation of ultrafine Au nanoparticles. All the containers and stirrers were cleaned with aqua regia before use. The standard process is as follows: 1.5 mL aqueous solution of sodium hydroxide (0.2 M) and 1 mL tetrakis(hydroxymethyl) phosphonium chloride (THPC) solution (0.96 wt%) were added into a three-necked flask and stirred. Then, 2 mL chloroauric acid (25 mM) was injected into the flask under a nitrogen atmosphere to obtain the ultrafine Au nanoparticles. Finally, the Au nanoparticles were washed with acetone several times and dispersed in 5 mL water. Please observe the ref. 29 for further information.
Functionalization of VO2@SiO2. The functional groups at the surface of the silica are predominantly silanol (Si–OH) or ethoxy (Si–OCH2CH3) groups. These groups were treated with 3-aminopropyltrimethoxysilane (APTMS) to modify the surface such that amine-terminated functional groups were exposed.24 The functionalization was carried out by mixing the solution of VO2@SiO2 nanoparticles with a certain amount of APTMS (sufficient to provide five monolayer coatings on the VO2@SiO2 particles). The area on the particle surface covered by each APTMS molecule was assumed to be nominally 0.6 nm2.30 However, considering the hydrolysis condensation of APTMS with itself and the deviation of the surface area, the excess of APTMS was used (typically 150 μL APTMS for VO2@SiO2 prepared by 0.005 g VO2). The mixture was stirred for 12 h and then refluxed at 80 °C for 1 h to promote covalent bonding between the APTMS and the VO2@SiO2 nanoparticles. Finally, the treated VO2@SiO2 particles were washed with ethanol for at least five times to remove excess APTMS and dispersed in 10 mL ethanol for subsequent reaction.
Preparation of VO2@SiO2@Au-seeds. 2.5 mL of ultrafine Au nanoparticles solution mentioned above was diluted with 37.5 mL water and then injected into VO2@SiO2 solution at the rate of 20 mL h−1 under stirring. When the injection completed, the mixture solution was stirred for 1 h and aged for another 2 h. Finally, the VO2@SiO2@Au-seeds particles were obtained, centrifuged and then redispersed in water at least five times to make sure free Au particles were removed.
Growth of Au seeds. 1.74 mL HAuCl4-aqueous solution (25 mM) was diluted with 97.26 mL of water and then 1 mL K2CO3 aqueous solution (1.8 mM) was added. The mixture was aged for at least 1 day in the dark under refrigeration.31 The precursor particles (VO2@SiO2@Au-seeds) were dispersed into the HAuCl4-aged solution. The volume of the gold salt solution depends on the desired size of the gold particles. A freshly prepared hydroxylamine hydrochloride (NH2OH·HCl) solution (130 mg L−1), as reducing agent, was injected at a rate of 1 mL min−1. The volume ratio of the salt solution and reducing solution was 1
:
1. The mixture was stirred for 1 h and aged for another 2 h. The whole reaction of gold seed growth was undertaken in a nitrogen atmosphere.
Preparation of VO2@SiO2@Au coating
To test the thermochromic properties, we used a simple method to fabricate the VO2@SiO2@Au coating on a glass substrate because the amount of the resulting sample was very little. After mixing the VO2@SiO2@Au aqueous solution with polyvinyl alcohol (PVA) solution, the mixture was coated on the glass and then heated at 65 °C until the moisture evaporated completely.
Characterization
The crystalline phases of the nanoparticles were determined by X'Pert Pro MPD diffractometer with Cu-Kα radiation with current and voltage of 40 mA and 40 kV, respectively, at a scan rate of 0.1° 2θ s−1. A Hitachi S-4800 scanning electron microscope (SEM) was used to obtain SEM images. The morphology and elemental composition of the nanoparticles were determined by a JEOL 2100F transmission electron microscope (TEM) with an energy dispersive spectrometry (EDS) attachment. The optical properties were measured on a Lambda 750 UV-Visible-near-IR spectrophotometer equipped with a heating unit and in the wavelength range of 400–2500 nm.
Results and discussion
Preparation of VO2@SiO2 nanoparticles
Fig. 1 shows the XRD pattern (Fig. 1a) and SEM image (Fig. 1b) of the original VO2 nanoparticles. In the XRD pattern, the four strongest peaks at 27.8°, 36.9°, 42.1°, and 55.4° are ascribed to the (110), (200), (210) and (220) crystal planes of VO2 (M) (JCPDS 43-1051), respectively. No impurity peaks appear, which demonstrates that the particles are pure VO2 (M). From Fig. 1b, the shape of the VO2 particles can be seen with an average size of 49 nm. These VO2 particles are coalesced. Dispersion of the VO2 nanoparticles is therefore indispensable before silica coating.
 |
| Fig. 1 (a) XRD pattern and (b) SEM image of the original VO2 nanoparticles. | |
As we all know, the haze (equal to the ratio of the diffuse transmittance and total transmittance) of the VO2 film increases with the particle size. Therefore, the expected size of VO2@SiO2 particles is 100 nm or even smaller. There are two factors controlling the thickness of the SiO2 shell: one is the ratio of the amount of TEOS and VO2 and the other is the reaction time. The synthetic conditions for different samples are listed in Table 1 and the corresponding sample SEM images are shown in Fig. 2. It can be found that the size of the VO2@SiO2 particles decreased from 160 nm to 153 nm (samples a–c) by reducing the amount of TEOS from 150 μL to 50 μL, while leaving other parameters fixed. Because the size was not significantly decreased, the reaction time was reduced to further tune the particle size (samples c–g). The size was decreased from 153 nm to 116 nm (samples c–e) after the soaking time was cut from 4 h to 1 h. However, if the time was further reduced, the size of the particles was not reduced (sample f and g). This implies that TEOS was still in excess. In addition, it was found that the particles began to coalesce if the reaction time was too short (Fig. 2g). Therefore, the reaction time was set to 1 h in the following experiment considering the time saving. Subsequently, the amount of TEOS was reduced from 50 μL to 5 μL. Finally, the average size was decreased to 95 nm when the amount of TEOS was reduced to 10 μL (Fig. 2i). It was estimated that the thickness of the silica shell was 23 nm (considering the diameter of the VO2 is 49 nm). When the amount of TEOS was 5 μL, the structure of the VO2@SiO2 particle tended to become rod-like with an average length of approximately 187.6 nm (Fig. 2j), which would lead to an increase in the haze. Thus, sample i was the one we expected.
Table 1 Summary of the conditions for VO2@SiO2 synthesis
Sample |
VO2 (mg) |
TEOSa (μL) |
NH4OHb (mL) |
Time (h) |
Size (nm) |
TEOS: purity > 97%. NH4OH : NH3 = 25 wt%. |
a |
5 |
150 |
2 |
4 |
160 |
b |
5 |
100 |
2 |
4 |
146 |
c |
5 |
50 |
2 |
4 |
153 |
d |
5 |
50 |
2 |
2 |
143 |
e |
5 |
50 |
2 |
1 |
116 |
f |
5 |
50 |
2 |
2/3 |
144 |
g |
5 |
50 |
2 |
1/3 |
126 |
h |
5 |
25 |
2 |
1 |
109 |
i |
5 |
10 |
2 |
1 |
95 |
j |
5 |
5 |
2 |
1 |
— |
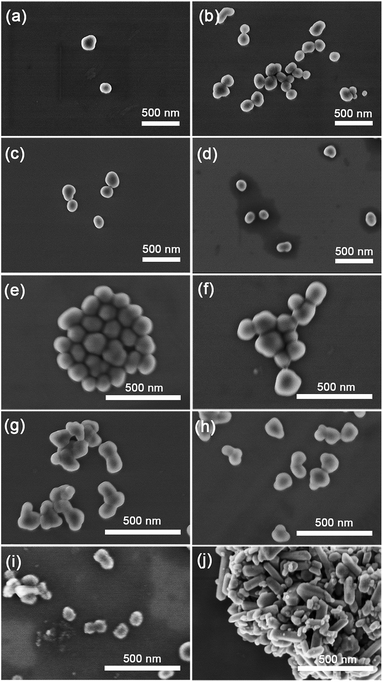 |
| Fig. 2 The SEM images of VO2@SiO2 prepared with the different experimental parameters. | |
The SiO2 shell thickness of sample i was determined by TEM. The VO2 particle was covered by a uniform and complete silica shell (Fig. 3a). The HRTEM (Fig. 3b) indicates interplanar distances of d = 0.320 nm, corresponding to monoclinic VO2 (011) planes (JCPDS 43-1051). It can be observed in Fig. 3c and d that the thickness of the silica shell is ∼14 nm, which is close to the estimated value from the SEM images (23 nm). The compositional scanning curves of the single VO2@SiO2 particle illustrate that the contents of Si were slightly higher at both ends than that inside of the particle (Fig. 3d), which further confirms that the VO2 particle was coated with a complete silica shell. Sample i was used in the subsequent reactions.
 |
| Fig. 3 (a) TEM and (b) HRTEM images of a VO2@SiO2 particle; (c) the STEM image and (d) the energy dispersive X-ray spectroscopy (EDS) line scan across the single particle. | |
Preparation of SiO2@Au nanoparticles
Before the preparation of the whole structure of VO2@SiO2@Au, we investigated and optimized the synthetic conditions of SiO2@Au. The Stöber method was used to prepare monodisperse SiO2 nanospheres with an average diameter of about 95 nm (Fig. 4a). The ultrafine Au nanoparticles as seeds with diameter under 5 nm (Fig. 4b) were synthesized by reducing HAuCl4 with THPC.31
 |
| Fig. 4 (a) SEM image of SiO2 particles and (b) TEM image of Au particles. | |
The SiO2@Au-seeds particles could be obtained by mixing the APTMS-functionalized SiO2 and the Au seeds in an aqueous solution. Before growth of Au seeds, it is necessary that all the free Au seeds are removed from the solution of the SiO2@Au-seeds particles. NH2OH·HCl, as a gentle reducing agent, was used to maintain the newly added gold ions reduced on the Au seeds only while new nucleation was prevented.
If the gold coating was formed in one step, it is difficult to remove the non-attached Au seeds for large particles. In addition, the gold coverage is usually low (<30%) because of the electrostatic repulsion between gold particles.32 Therefore, we utilized a two-step process to synthesize SiO2@Au (and VO2@SiO2@Au).
The SEM images of SiO2@Au nanoparticles with various sizes of Au are shown in Fig. 5. The size of the Au particle was easily altered by the amount of HAuCl4-aged solution. When the amount of gold salt solution was 10 mL, 30 mL, and 50 mL, the gold particle size on SiO2 particles was 5.6 nm (Fig. 5a and b), 8.8 nm (Fig. 5c and d), and 21.8 nm (Fig. 5e and f), respectively. It can be found that a monolayer of Au particles was well-distributed and coated the silica surface.
 |
| Fig. 5 The SEM images of SiO2@Au nanoparticles with different amounts of gold salt solution: (a and b) 10 mL, (c and d) 30 mL and (e and f) 50 mL. | |
The color of the solutions (Fig. 6a) can be altered from red to blue. The absorption spectra of three different SiO2@Au solutions are shown in Fig. 6b. It is well known that Au nanoparticles display various colors due to different λSPR. However, there is no absorption peak at the spectrum of Au seeds solution (red line) because of their small size (<5 nm)33 and chemical interface damping (capped by the P-containing organic groups, which come from THPC).34 Similarly, there is no peak in the spectrum of SiO2@Au-seeds solution (orange line), even though the dielectric properties around the Au particle have changed. After the Au growth, the absorption peak is present. When the size of Au particle on the silica is 5.6 nm, the λSPR locates at 540 nm (green line) and the λSPR shifts to 574 nm (purple line) and 664 nm (black line) as the size of Au particles increase to 8.8 nm and 21.8 nm, respectively. Moreover, it is found that the full width at half maximum (FWHM) becomes greater with the size increase.
 |
| Fig. 6 (a) Images and (b) absorption spectra of different solutions. | |
The red shift of λSPR and the increase of FWHM are related to the size effect of Au nanoparticles. According to the modified equation given by Bohren and Huffman35 based on Mie's theory,18,36 the dipolar oscillation of a spherically shaped metal particle with radius (a) is:
|
 | (1) |
where
εm is the dielectric constant of the surrounding medium and
ε1 and
ε2 are the real and imaginary parts of the complex dielectric function for the material itself. The optical response condition is fulfilled when the denominator in
eqn (1) is roughly equal to 0:
|
 | (2) |
After using the equation ε1 = 1 − ωp2/ω2 (plasma frequency ωp = 8.9 eV for bulk gold37) based on the Drude model and converting frequency to wavelength via λ = 2πc/ω, the wavelength of a resonance peak (λSPR) can be given by:
|
 | (3) |
It can be found that the λSPR can be tuned by the size of metal nanoparticles and the dielectric constant of the surrounding medium because the increase of metal nanoparticles' size from 5.6 nm to 21.8 nm led to the red shift of the SPR band from 540 nm to 664 nm. According to eqn (3), VO2@SiO2@Au particles with different λSPR could be designed and thus VO2-based films exhibiting various colors could be produced. In addition, for gold particles larger than ∼20 nm, the dipole resonances approximation is no longer valid, whereas the higher order resonances play an important role.38 The retardation effects of the electromagnetic field across the particle can cause huge red shifts and increase in FWHM, as we found in Fig. 6b.
Properties of VO2@SiO2@Au films
By combining the two methods mentioned above, VO2@SiO2@Au particles were synthesized. Fig. 7a displays the HRTEM image of a VO2@SiO2@Au-seeds particle. The interplanar distance of d = 0.32 nm indicates that the core is VO2. The yellow line marks the boundary between VO2 and amorphous silica. It can also be found that the Au seeds (the darker areas marked with red circles in Fig. 7a) were distributed on the VO2@SiO2 particle surface. The SEM images of VO2@SiO2@Au particles are shown in Fig. 7b and c. As we can observe, the VO2@SiO2 particles are coated with gold particles, which is similar to SiO2@Au (Fig. 5). After mixing the particles with PVA solution, the VO2@SiO2@Au films were prepared. These films display a series of colors from yellow to blue and are shown in Fig. 8. There are obvious differences in color between the VO2 films (sample I) and VO2@SiO2@Au films (sample II, III, IV).
 |
| Fig. 7 (a) HRTEM image of VO2@SiO2@Au-seeds nanoparticle and (b and c) SEM images of VO2@SiO2@Au nanoparticles. | |
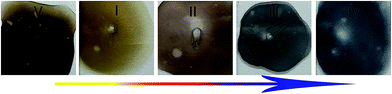 |
| Fig. 8 The films with a variety of color. From left to right: sample V: VO2@SiO2@Ag film, sample I: VO2 film, sample II: VO2@SiO2@Au film grown in 10 mL HAuCl4, sample III: VO2@SiO2@Au film grown in 30 mL HAuCl4, and sample IV: VO2@SiO2@Au film grown in 50 mL HAuCl4. | |
The transmittance spectra of the VO2 film and three different VO2@SiO2@Au films at 25 °C and 100 °C are shown in Fig. 9a–e. There is an extra shoulder at 525 nm (Fig. 9b), 555 nm (Fig. 9c) and 640 nm (Fig. 9d), corresponding to samples II, III, and IV, respectively. Even though the resonance peak of sample II is not obvious, the film exhibits a brick red color (Fig. 8, sample II), which great differs from the color of VO2 film (Fig. 8, sample I). When the λSPR shifts to 555 nm and 640 nm, due to the volume of HAuCl4 increasing to 30 mL and 50 mL, the color is tuned to purple (Fig. 8, sample III) and blue (Fig. 8, sample IV), respectively. We also prepared the VO2@SiO2@Ag film by modifying our method. There is also an extra shoulder at 440 nm (Fig. 9e), which corresponds to the color that should be yellow. The VO2@SiO2@Ag film (Fig. 8, sample V) shows a black color because of its high thickness, but a brown color can be found at the edges wherein the film is relatively thin. This result demonstrates that our method can be applied in other noble metal coatings, which infers that the λSPR can be modulated in almost the whole visible spectrum. As a result, the color of the VO2-based film can be tailored as desired.
 |
| Fig. 9 The transmittance spectra of the different samples: (a) sample I, (b) sample II, (c) sample III, (d) sample IV, (e) sample V, for both the semiconductor phase measured at 25 °C (black curves) and the metal phase measured at 100 °C (red curves). The resonance positions are marked with a blue solid circle. (f) The hysteresis loops at 2000 nm of sample I (black-square dotted line), II (red-circle dotted line), III (green-triangle dotted line) and V (blue-start dotted line). | |
To further quantify the potential application of the films as coatings on smart windows, the solar modulation ability (ΔTsol) was calculated. The ΔTsol is defined in eqn (4):
|
 | (4) |
where
TL(
λ) and
TH(
λ) denote the transmittance of low temperature and high temperature phases, respectively, at wavelength
λ,
φsol is the solar spectral irradiance for the air mass 1.5. The Δ
Tsol of all the samples are summarized in
Table 2. It is worth noting that the Δ
Tsol has a significant decrease as the
λSPR red shifts. The possible reason could be that VO
2 was dissolved during the growth of Au seeds. In order to identify what agent would react with VO
2, VO
2 powder was put into the NH
2OH·HCl and the HAuCl
4-aged solution with the same concentration as in the preparation of VO
2@SiO
2@Au. After stirring the mixture for 30 min, the VO
2 powder dissolved in the HAuCl
4-aged solution and the color of the solution altered from colorless to blue, while there was no significant change in the NH
2OH·HCl solution. This proved that a reaction occurred between HAuCl
4 and VO
2 during the growth process. Moreover, the dissolution could be slowed down by the SiO
2 shell, but not prevented completely because the growth time of sample III is 30 min, but the Δ
Tsol is still 2.64%, not zero. With respect to sample IV, the Δ
Tsol is very close to 0, whereas the growth time is 50 min. In the gold growth process, the VO
2@SiO
2@Au-seeds particles were dispersed in HAuCl
4 solution at first and then the NH
2OH·HCl solution was injected at a rate of 1 mL min
−1. At the beginning of this process VO
2 was undesirably dissolved by HAuCl
4 due to the lack of reductant. If Au particles grew to larger size, more HAuCl
4 and a longer reaction time were needed, so that more VO
2 would be dissolved. In addition, the
λSPR blue shift is observed in the spectra of VO
2@SiO
2@Au (525 nm, 555 nm, and 640 nm) compared to that in the case of SiO
2@Au (540 nm, 574 nm, and 664 nm). The
λSPR should be equal because the same amount of gold salt was added. The blue shift results from decrease of the gold size caused by the loss of HAuCl
4. Therefore, it is important to note that the reduction process should be completed within 10 min to avoid the serious loss of VO
2.
Table 2 Summary of the optical properties of different samples
Sample |
λSPR |
Color |
ΔTsol |
I |
— |
Brown-yellow |
11.93% |
II |
525 nm |
Brick-red |
7.52% |
III |
555 nm |
Purple |
2.64% |
IV |
640 nm |
Blue |
0.90% |
V |
440 nm |
Brown |
4.705 |
The hysteresis curves of sample I, sample II, sample III, and sample V were investigated by recording the temperature-dependent optical transmittance during the heating–cooling cycles at 2000 nm, as illustrated in Fig. 9f. The d(Tr)/d(T)–T curve was used to describe the definition of the transition parameters of heating and cooling branches (Fig. 10). Each of the d(Tr)/d(T)–T lines has been fitted with a Gaussian function using the peak fitting module of the Origin Pro 2015 software. The temperature corresponding to the maximum d(Tr)/d(T) is defined as the phase transition temperature of the heating and cooling branches, denoted as Th and Tc, respectively. For cooling branches with the appearance of two peaks in the d(Tr)/d(T)–T curves, the Tc values are determined by the main peak. The phase transition temperature (Tt) of the film is defined as:
and the hysteresis loop width (Δ
Tt) is defined as the difference between
Th and
Tc.
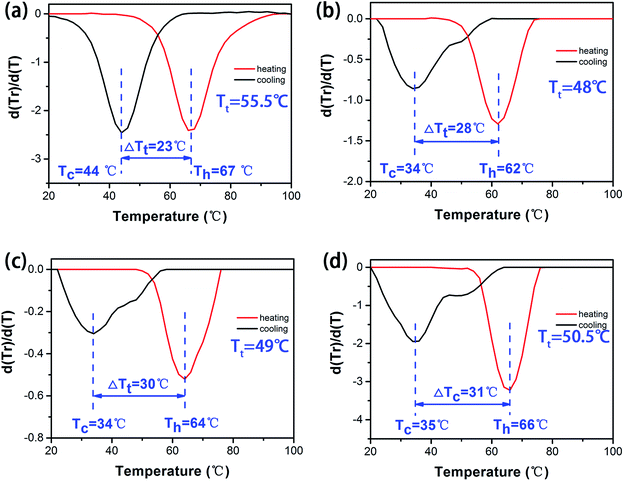 |
| Fig. 10 The d(Tr)/d(T)–T lines of the films to describe the hysteresis of heating and cooling branches at 2000 nm. (a) Sample I, (b) sample II, (c) sample III; and (d) sample V. | |
The transition temperature Tt is reduced by ∼6 °C and the hysteresis loop width becomes greater (∼7 °C), compared with the intrinsic VO2 film (Fig. 10). This result may be understood on the basis of the theory of Zylbersztejn and Mott.39 The work function of Au or Ag (ΦAu = 5.1 eV, ΦAg = 4.26 eV) is lower than that of VO2 (Φs = 5.4 eV), as shown in Fig. 11a. Although there is a silica layer (∼14 nm) between the VO2 and Au, it is very possible that the electrons in VO2 particles would be driven across the silica layer to Au until their Fermi levels become aligned due to the quantum tunneling effect (Fig. 11b),40 which is similar to the model proposed by Xu et al.41 The electron injection leads to a increase of electron concentration in the VO2 surface, which causes the phase transition to become more flexible. This implies that the phase transition may be triggered at a relatively lower temperature, rather than at the intrinsic Tt. As a result, the VO2@SiO2@Au (and VO2@SiO2@Ag) structure can reduce the intrinsic transition temperature.
 |
| Fig. 11 Energy diagrams of Au and VO2 (a) before and (b) after approach across the SiO2 layer. | |
Conclusions
In this study, we have developed a novel and facile method to tailor the unpleasant color of VO2 films. VO2@SiO2@Au core–shell structures with applicability and versatility have been synthesized via a solution process. A uniform silica shell, which could improve the anti-oxidation of VO2, was formed around the VO2 particle first and then ultrafine Au particles were embedded on the surface of SiO2. The size of the Au particles displayed a dependence on the amount of gold salt used. Larger Au particles obtained a red shift of the resonance peak (from 540 nm to 664 nm). The VO2@SiO2@Au film's color could be tuned from brick red to blue. Furthermore, a VO2@SiO2@Ag film with a brown color was also prepared, the resonance peak of which was at 440 nm. This result implied that not only Au coating, but also other noble metal coatings could be synthesized by our strategy. By changing the type of metal and particle size, the resonance peak can be tuned in a rather large spectral region, which means that the color of VO2 smart films can be tailored based on the demands of practical applications. The phase transition temperature was reduced by ∼6 °C due to the metal coating. This study will pave the way for the application of VO2-base thermochromic smart films.
Acknowledgements
This study was supported by the Major Science and Technology Projects of Guangdong Province (No. 2013A011401011), Science and Technology Project of Guangdong Province (No. 2014A010106018), Pearl River Star of Science and Technology (No. 2014J2200078), CAS Key Laboratory of Renewable Energy Fund of GIEC (No. y507j41001), the National Natural Science Foundation of China (No. 51506205), Guangdong Joint Innovation Project in Guangdong Province (No. 2014B050505015), Construction Project of Guangdong Solar Thermal and Solar Thermal Advanced Materials Engineering Technology Research Center (No. 2014B090904071).
References
- F. Morin, Phys. Rev. Lett., 1959, 3, 34 CrossRef CAS.
- W. Burkhardt, T. Christmann, B. Meyer, W. Niessner, D. Schalch and A. Scharmann, Thin solid films, 1999, 345, 229–235 CrossRef CAS.
- T. D. Manning and I. P. Parkin, J. Mater. Chem., 2004, 14, 2554–2559 RSC.
- N. Mlyuka, G. Niklasson and C. G. Granqvist, Appl. Phys. Lett., 2009, 95, 171909 CrossRef.
- D. Li, M. Li, J. Pan, Y. Luo, H. Wu, Y. Zhang and G. Li, ACS Appl. Mater. Interfaces, 2014, 6, 6555–6561 CAS.
- N. Shen, S. Chen, Z. Chen, X. Liu, C. Cao, B. Dong, H. Luo, J. Liu and Y. Gao, J. Mater. Chem. A, 2014, 2, 15087–15093 CAS.
- P. Jin, G. Xu, M. Tazawa and K. Yoshimura, Appl. Phys. A, 2003, 77, 455–459 CrossRef CAS.
- H. K. Chen, H. C. Hung, T. C. K. Yang and S. F. Wang, J. Non-Cryst. Solids, 2004, 347, 138–143 CrossRef CAS.
- L. Dai, S. Chen, J. Liu, Y. Gao, J. Zhou, Z. Chen, C. Cao, H. Luo and M. Kanehira, Phys. Chem. Chem. Phys., 2013, 15, 11723–11729 RSC.
- J. Zhou, Y. Gao, X. Liu, Z. Chen, L. Dai, C. Cao, H. Luo, M. Kanahira, C. Sun and L. Yan, Phys. Chem. Chem. Phys., 2013, 15, 7505–7511 RSC.
- Y. Li, S. Ji, Y. Gao, H. Luo and M. Kanehira, Sci. Rep., 2013, 3, 6603–6614 Search PubMed.
- U. Kreibig and M. Vollmer, Optical Properties of Metal Clusters, Springer, Berlin, 1995 Search PubMed.
- E. Hutter and J. H. Fendler, Adv. Mater., 2004, 16, 1685–1706 CrossRef CAS.
- K. L. Kelly, E. Coronado, L. L. Zhao and G. C. Schatz, J. Phys. Chem. B, 2003, 107, 668–677 CrossRef CAS.
- W. A. Murray and W. L. Barnes, Adv. Mater., 2007, 19, 3771–3782 CrossRef CAS.
- G. Xu, C. M. Huang, P. Jin, M. Tazawa and D. M. Chen, J. Appl. Phys., 2008, 104, 053101 CrossRef.
- G. Xu, C. M. Huang, M. Tazawa, P. Jin and L. H. Chen, Opt. Commun., 2009, 282, 896–902 CrossRef CAS.
- H. Zhou, X. Cao, M. Jiang, S. Bao and P. Jin, Laser Photonics Rev., 2014, 8, 617–625 CrossRef CAS.
- R. Binions, C. Piccirillo, R. G. Palgrave and I. P. Parkin, Chem. Vap. Deposition, 2008, 14, 33–39 CrossRef CAS.
- M. Saeli, C. Piccirillo, I. P. Parkin, I. Ridley and R. Binions, Sol. Energy Mater. Sol. Cells, 2010, 94, 141–151 CrossRef CAS.
- A. I. Maaroof, D.-g. Cho, B. J. Kim, H. T. Kim and S. Hong, J. Phys. Chem. C, 2013, 117, 19601–19605 CAS.
- M. Cortie, A. Dowd, N. Harris and M. Ford, Phys. Rev. B: Condens. Matter Mater. Phys., 2007, 75, 113405 CrossRef.
- Y. Gao, S. Wang, H. Luo, L. Dai, C. Cao, Y. Liu, Z. Chen and M. Kanehira, Energy Environ. Sci., 2012, 5, 6104–6110 CAS.
- S. L. Westcott, S. J. Oldenburg, T. R. Lee and N. J. Halas, Langmuir, 1998, 14, 5396–5401 CrossRef CAS.
- C. Graf and A. van Blaaderen, Langmuir, 2002, 18, 524–534 CrossRef CAS.
- R. D. Badley, W. T. Ford, F. J. McEnroe and R. A. Assink, Langmuir, 1990, 6, 792–801 CrossRef CAS.
- X. Xiao, H. Zhang, G. Chai, Y. Sun, T. Yang, H. Cheng, L. Chen, L. Miao and G. Xu, Mater. Res. Bull., 2014, 51, 6–12 CrossRef CAS.
- C. Graf, D. L. Vossen, A. Imhof and A. van Blaaderen, Langmuir, 2003, 19, 6693–6700 CrossRef CAS.
- D. G. Duff, A. Baiker and P. P. Edwards, Langmuir, 1993, 9, 2301–2309 CrossRef CAS.
- T. G. Waddell, D. E. Leyden and M. T. DeBello, J. Am. Chem. Soc., 1981, 103, 5303–5307 CrossRef CAS.
- D. G. Duff, A. Baiker, I. Gameson and P. P. Edwards, Langmuir, 1993, 9, 2310–2317 CrossRef CAS.
- K. C. Grabar, K. J. Allison, B. E. Baker, R. M. Bright, K. R. Brown, R. G. Freeman, A. P. Fox, C. D. Keating, M. D. Musick and M. J. Natan, Langmuir, 1996, 12, 2353–2361 CrossRef CAS.
- M. M. Alvarez, J. T. Khoury, T. G. Schaaff, M. N. Shafigullin, I. Vezmar and R. L. Whetten, J. Phys. Chem. B, 1997, 101, 3706–3712 CrossRef CAS.
- M. Garcia, J. De la Venta, P. Crespo, J. Llopis, S. Penadés, A. Fernández and A. Hernando, Phys. Rev. B: Condens. Matter Mater. Phys., 2005, 72, 241403 CrossRef.
- C. F. Bohren and D. R. Huffman, Absorption and Scattering of Light by Small Particles, Wiley-VCH, Weinheim, 2008 Search PubMed.
- G. Mie, Ann. Phys., 1908, 330, 377–445 CrossRef.
- P. B. Johnson and R. W. Christy, Phys. Rev. B: Solid State, 1972, 6, 4370 CrossRef CAS.
- S. K. Ghosh and T. Pal, Chem. Rev., 2007, 107, 4797–4862 CrossRef CAS PubMed.
- A. Zylbersztejn and N. Mott, Phys. Rev. B: Solid State, 1975, 11, 4383 CrossRef CAS.
- S. Fonash, Solar Cell Device Physics, Elsevier, Amsterdam, 2012 Search PubMed.
- G. Xu, C. M. Huang, M. Tazawa, P. Jin, D. M. Chen and L. Miao, Appl. Phys. Lett., 2008, 93, 1911 Search PubMed.
Footnote |
† Electronic supplementary information (ESI) available. See DOI: 10.1039/c6ra07594k |
|
This journal is © The Royal Society of Chemistry 2016 |