DOI:
10.1039/C6RA06942H
(Paper)
RSC Adv., 2016,
6, 41340-41347
An aggregation-induced emission enhancement fluorescent benzoxazine-derived macromolecule: catalyst-free synthesis and its preliminary application for the determination of aqueous picric acid†
Received
16th March 2016
, Accepted 12th April 2016
First published on 13th April 2016
Introduction
As a result of their excellent film-forming ability,1 good optical and chemical stability,2 and low toxicity,3 macromolecules with fluorescent properties have been used successfully in many research fields, such as polymer light-emitting diodes,4 waveguide materials5 and as chemo- /bio-optical probes.6 Metal-catalysed synthetic methods (e.g. the Suzuki,7 Sonogashira,8 Stille9 and Click10 reactions) have been used to prepare various macromolecules with fluorescent properties. However, these protocols have the disadvantages that the metal catalysts are expensive and the residual catalysts are difficult to completely remove from the products and may have unfavourable effects on the optical properties.11 The catalyst-free preparation of macromolecules with fluorescent properties is highly desirable in their large-scale preparation. Despite the progress achieved in the development of non-catalyst polymerization strategies for the preparation of macromolecules with fluorescent properties (e.g. Schiff base polymerization,12 Diels–Alder addition,13 thiol–Br condensation14 and thiol–ene click polymerization15), the development of other catalyst-free synthetic methods is desirable and will provide more flexibility in the preparation of novel macromolecules with fluorescent properties.
As a result of their convenient syntheses, non-catalyst thermal curability and good mechanical performance, benzoxazine derivatives have attracted increasing interest as high-performance thermoset resins.16 Benzoxazine-based small molecules can be synthesized from phenolic compounds, formaldehyde and primary amines via the Mannich reaction under mild conditions without a catalyst.17 Because of the simplicity of the preparation and the remarkable flexibility of the selection of reactants, a variety of benzoxazine derivatives bearing different functional groups have been successfully synthesized to meet different application requirements.18–20 In addition to the successful preparation of benzoxazine-based small molecules, a series of benzoxazine-based macromolecules have also been prepared using dihydric phenols and diamines as the starting monomers.21 This provides more attractive properties for the resultant benzoxazines, such as the ability to form films and other specific mechanical and physical properties.22–25 Although there have been extensive studies of the thermal and mechanical properties of benzoxazines,26 there have been few studies of fluorescent benzoxazines.27–29
Picric acid (PA), a well-known explosive, has been widely used in terrorist activities, military explosives, fireworks and the sterilization of medicines and dyes.30,31 The discharge of PA to the environment leads to serious pollution of the atmosphere and aqueous environments.30,32 PA causes many health problems, such as damage to the respiratory system, skin irritation, nausea and skin allergies.33 Infrared spectrometry, electrochemical methods, X-ray diffraction, thermal neutron analysis, transmission assays and fluorescence sensing have all been successfully used to determine PA.34–38 Among these identification methods, optical probes have some attractive advantages, such as high sensitivity, practical and simple detection procedures, and the relatively low cost of the instrumentation. However, conventional organo-soluble fluorescent PA probes have encountered an inherent aggregation-induced fluorescence quenching problem39 and are difficult to use in aqueous environments. One way of solving this issue is to use water-insoluble agents with aggregation-induced emission enhancement (AIEE)40,41 for the determination of PA in aqueous environments.
We report here the synthesis of a novel macromolecular benzoxazine derivative, TPE-BOZ, via a catalyst-free, click-type Mannich reaction. The optical properties of TPE-BOZ were investigated in mixtures of ethanol (good solvent) and water (poor solvent) to evaluate its AIEE characteristics. A preliminary investigation of the application of TPE-BOZ as an optical probe for the determination of PA in a high water fraction medium (ethanol
:
water 1
:
9 v/v) was carried out.
Experimental
General
Unless otherwise stated, all reagents were purchased from Adamas-beta Chemical and were used without further purification. Tetrahydrofuran (THF) and toluene were distilled from sodium in the presence of benzophenone and were degassed before use. Dichloromethane and chloroform were distilled from CaH2 before use. In the titration experiments, all the detected compounds (2,4-dinitrophenol (2,4-DNP), p-nitrophenol, o-nitrophenol, p-nitrotoluene, nitrobenzene, nitromethane and phenol) were dissolved in ethanol (10 mL) to give a 10−1 mol L−1 ethanol solution; concentrations of PA <10−1 mol L−1 were dissolved in distilled water to obtain the corresponding solution. The tetraphenylethylene (TPE) derivative containing two hydroxyl groups (M2) was synthesized according to a previously reported procedure.42 Caution: PA is highly explosive and should be handled carefully and only in very small quantities.
Instrumentation
1H NMR and 13C NMR spectra were recorded on a Varian INOVA-400 spectrometer operating at 400 MHz (for 1H) and 100 MHz (for 13C) in a deuterated chloroform solution with tetramethylsilane (TMS, δ = 0) as the internal reference. FT-IR spectra were obtained on an Equinox 55 FTIR spectrometer with KBr pellets. Matrix-assisted laser desorption–ionization time-of-flight mass spectrometry (MALDI-TOF-MS) spectra were recorded on a Bruker Autoflex mass spectrometer. Scanning electron microscopy (SEM) was carried out using a Zeiss EVO MA 15 scanning electron microscope. UV-visible and fluorescence spectrum was determined using a Shimadzu UV-2450 UV-visible spectrometer and a Shimadzu RF-5301PC fluorescence spectrometer at room temperature.
Preparation of TPE-hexylamine-derived benzoxazine (M3)
N-Hexylamine (0.08 g, 0.8 mmol) and formaldehyde (37% by weight in water, 0.13 g, 1.6 mmol) were dissolved in various solvents (Table 1) (10 mL) and stirred at RT under a nitrogen atmosphere. After half an hour, M2 (0.145 g, 0.4 mmol) was added to the reaction mixture. After reacting for 6 h, the solution was washed three times with distilled water, the organic phase was collected and the solvent was removed using a rotary evaporator. The crude product was purified on a silica gel column using ethyl acetate
:
petroleum ether (1
:
20 v/v) as the eluent. Table 1 lists the yields of product obtained using different solvents.
Table 1 Effect of solvent on the synthesis of M3
Run |
Solvent |
Temperature (°C) |
Yielda (%) |
Reaction time fixed at 6 h. |
1 |
Chloroform |
Reflux |
93 |
2 |
Toluene |
90 |
90 |
3 |
1,4-Dioxane |
90 |
88 |
4 |
DMSO |
90 |
70 |
5 |
DMF |
90 |
67 |
6 |
Ethanol |
Reflux |
58 |
7 |
THF |
Reflux |
73 |
1H NMR (400 MHz, CDCl3) δ (TMS, ppm) 7.17–6.97 (m, 10H), 6.78–6.67 (m, 2H), 6.62–6.40 (m, 4H), 4.79 (d, 4H), 3.72 (d, 4H), 2.71–2.57 (m, 4H), 1.76–1.33 (m, 5H), 1.33–1.23 (m, 11H), 0.96–0.81 (m, 6H). 13C NMR (100 MHz, CDCl3) δ (TMS, ppm) 152.60, 144.03, 139.69, 135.89, 131.37, 130.72, 130.47, 127.54, 126.13, 119.06, 115.31, 82.61, 51.35, 50.01, 31.74, 28.05, 26.94, 22.64, 14.07. FTIR (KBr, cm−1): 921, 1490 (trisubstituted benzene ring), 1230, 1069 (C–O–C of oxazine ring), 2987–3077 (Ar-H), 2857–2960 (–C6H13).
Preparation of TPE-polyetheramine-derived macromolecular benzoxazine (TPE-BOZ)
Poly(propylene glycol)bis(2-aminopropylether) (PEA) (Mn = 230, 0.092 g, 0.4 mmol) and formaldehyde (37% by weight in water, 0.13 g, 1.6 mmol) were dissolved in chloroform (10 mL) and stirred at RT under a nitrogen atmosphere. After half an hour, M2 (0.146 mg, 0.4 mmol) was added to the reaction tube. After refluxing for 6 h, the solution was washed three times with distilled water and the chloroform was evaporated. The crude product was re-dissolved in toluene and re-precipitated from petroleum ether to give the final TPE-BOZ product (yield 98%).
1H NMR (400 MHz, CDCl3) δ (TMS, ppm) 7.13–6.44 (16H, Ar-H), 5.11–4.62 (2H, N–CH2–O), 3.90–3.61 (2H, C–CH2–N), 3.61–3.22 (4H), 3.18–2.97 (m, 2H), 1.32–0.77 (9H, alkyl H). FTIR (KBr, cm−1): 925, 1490 (trisubstituted benzene ring), 1262, 1072 (C–O–C of oxazine ring), 3000–3083 (Ar-H). MALDI-TOF-MS (m/z): 1512.50.
Results and discussion
Synthesis
The synthesis of the benzoxazine-derived macromolecule (TPE-BOZ) was fulfilled according to the synthetic route shown in Scheme 1. A small molecular benzoxazine model compound, M3, was initially synthesized using a phenolic TPE-based compound (M2), n-hexylamine and formaldehyde as the starting materials (Scheme 1) to evaluate the feasibility of the design. The preparation of M3 was conducted in a series of solvents at a fixed reaction time (6 h) to investigate the influence of the reaction solvent. Table 1 shows that the reaction in chloroform gave the highest yield among these systems. This may be because non-polar solvents are beneficial to the ring closure of the intermediate Mannich base, whereas polar solvents tend to induce an open loop.43,44 The immiscibility of chloroform with water (introduced with the aqueous formaldehyde solution and as a condensate during the reaction) can also promote the reaction, as reported previously.45
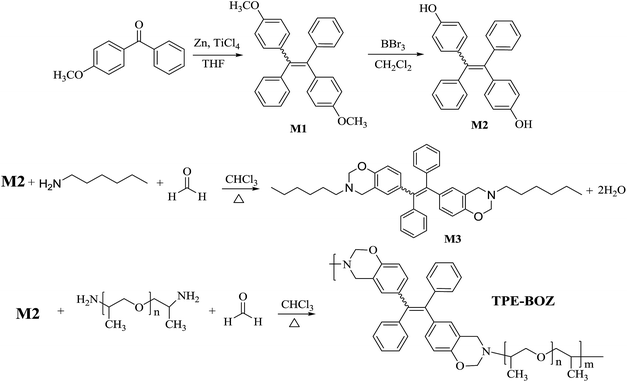 |
| Scheme 1 Synthetic route of benzoxazines. | |
The chemical structure of M3 was characterized by 1H NMR (Fig. S1†), 13C NMR (Fig. S2†) and FT-IR (Fig. S3†) analyses. In Fig. S1,† the resonance peaks at 4.79 and 3.72 ppm can be assigned to the characteristic protons of the benzoxazine ring (marked as protons g and h).46,47 Signals at δ 2.62 ppm correspond to another set of methylene protons adjacent to N-atoms (marked as f). Aromatic protons were seen at 7.17–6.97 ppm. As shown in the 13C NMR spectrum (Fig. S2†), the characteristic carbon signals of the benzoxazine ring (O–C–N and N–C–Ar, marked as 8 and 7) appear at 82.61 and 51.35 ppm, respectively. The signal of the aromatic carbon atom, which links directly to the O-atom in the TPE skeleton (marked as 10 in Fig. S2†), appears at 152.60 ppm. The assignment of the other carbons can also be traced in Fig. S2.† The FTIR spectrum of M3 (Fig. S3†) shows the characteristic absorption signals of the benzoxazine ring at c. 921 and 1490 cm−1 (corresponding to the trisubstituted benzene ring).47 The signals at 1230 and 1069 cm−1 correspond to the asymmetrical and symmetrical stretching of C–O–C of the oxazine ring, respectively.47 The absorption signals at 2987–3077 cm−1 can be assigned to the stretching of the aromatic protons on TPE and the peaks at 2857–2960 cm−1 were assigned to the absorption of alkyl protons. These characterizations indicated the successful synthesis of M3 through the catalyst-free synthetic protocol under mild reaction conditions.
Given the successful synthesis of M3, we further extended our attention to preparing macromolecular benzoxazine with M2 (dihydric phenols), PEA (diamines) and formaldehyde as the starting monomers (Scheme 1) in refluxing chloroform without the use of a catalyst. The obtained product (TPE-BOZ) was soluble in common organic solvents – such as THF, chloroform, N,N-dimethylformamide, DMSO and ethanol – but was insoluble in water and petroleum ether. To verify the chemical structure of TPE-BOZ, the 1H NMR spectra of TPE-BOZ and PEA are compared in Fig. S4,† which shows that there was a significant difference between the 1H NMR spectra of TPE-BOZ and PEA. Compared with the spectrum of pristine PEA (Fig. S4a†), new resonance peaks appeared at 5.11–4.62 and 3.90–3.61 ppm, which could be assigned to the characteristic protons located at the benzoxazine ring (marked as protons d and e) as reported previously.46,47 Apart from these protons, aromatic proton signals appeared exclusively in Fig. S4b,† which provided straightforward evidence for the successful introduction of the aromatic TPE segment into TPE-BOZ. The FTIR analysis also strongly supported the successful preparation of TPE-BOZ. The absorption signal at c. 3367 cm−1, which can be assigned to the –NH2 groups in PEA (Fig. S5a†), weakened significantly in the spectrum of TPE-BOZ (Fig. S5b†), suggesting the appreciable consumption of –NH2 during the coupling reaction between M2 and PEA. In Fig. S5b,† the characteristic absorption signals of the benzoxazine ring appear at c. 925, 1490, 1262 and 1027 cm−1, respectively. Absorption signals in the range 3000–3083 cm−1, which correspond to the stretching of aromatic protons, appeared clearly in the spectrum of TPE-BOZ (Fig. S5b†). These analyses strongly demonstrate that TPE-BOZ was successfully synthesized.
The molecular weight of TPE-BOZ was first characterized by gel permeation chromatography using THF as the eluent. Unfortunately, we did not obtain a reliable result, which might be due to the strong adsorption of TPE-BOZ on the column during the test, possibly a result of the presence of polar polyether groups in the structure of TPE-BOZ. We then used MALDI-TOF-MS to characterize the molecular weight of TPE-BOZ. MALDI-TOF-MS showed that TPE-BOZ was an oligomer-like molecule with a molecular weight of c. 1500 (Fig. S6†), which corresponds to three repeating units in its backbone. Despite the relatively low molecular weight of TPE-BOZ, we found that it had a good ability to form films.
Optical properties
As described previously,48 TPE is a typical AIEE agent. Based on the successful introduction of TPE into TPE-BOZ, TPE-BOZ was expected to also show AIEE characteristics. The UV-visible (Fig. S8†) and fluorescence (Fig. 1) changes of TPE-BOZ in mixed solvents of ethanol (good solvent) and water (poor solvent) at different composition ratios were therefore investigated. TPE-BOZ emitted a faint luminescence in pure ethanol (Fig. 1a); the fluorescence intensity of TPE-BOZ remained almost unchanged in solvent mixtures with a water fraction <40% and increased steadily with the addition of more water (Fig. 1b). Such fluorescence alteration was observed visually (Fig. 1c), confirming that TPE-BOZ is a typical AIEE-type molecule. To find straightforward evidence for the poor solvent-induced intermolecular aggregation of TPE-BOZ, SEM analysis of TPE-BOZ was conducted in ethanol
:
water (1
:
9 v/v). Fig. S7† shows that molecules of TPE-BOZ assembled to form sphere-like nanoparticles, suggesting the occurrence of aggregation in this medium.
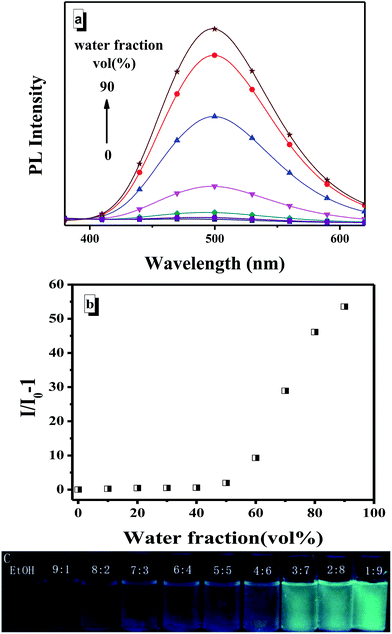 |
| Fig. 1 Emission spectra of TPE-BOZ (1.0 × 10−5 M) in EtOH/water mixtures with different fractions of water (a) excitation at 320 nm. (b) Variation in (I/I0 − 1) of TPE-BOZ (1.0 × 10−5 M) with the fraction of water in EtOH/water mixtures. (c) Visual images of TPE-BOZ (1.0 × 10−5 M) under a hand-held UV lamp (365 nm) in EtOH/water mixtures with different solvent ratios. | |
The fluorescence of TPE-BOZ in the film state was investigated by spin-coating its ethanol solution onto a glass substrate (Fig. 2). Although the molecular weight of TPE-BOZ was not very large, it had a satisfactory film-forming ability. The resultant film was uniform and fluorescent (Fig. 2, inset). The fluorescence spectrum of TPE-BOZ film peaked at c. 498 nm (Fig. 2) and the whole spectrum resembled that seen in ethanol–water (1
:
9 v/v), which might be a result of intermolecular aggregation in both instances.
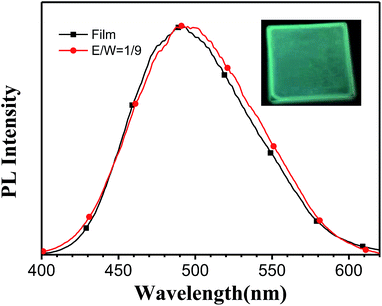 |
| Fig. 2 Fluorescence spectra (λex = 320 nm) of TPE-BOZ (1.0 × 10−5 M) in ETOH : water mixture (1 : 9 v/v) and in a solid film; inset is a photograph of the film under a hand-held UV lamp (365 nm). | |
Preliminary investigation of the optical response of TPE-BOZ to PA
The intriguing AIEE characteristics of TPE-BOZ prompted us to explore its potential applications as an optical probe in aqueous environments. Considering the presence of electron-rich ether (C–O) and TPE segments in the structure of TPE-BOZ, we speculated that its fluorescence might be altered by the introduction of an electron-withdrawing agent into the probing medium. Based on previous reports that have shown macromolecule aggregates to significantly improve the sensitivity of the corresponding probes,49,50 we used the macromolecular aggregate of TPE-BOZ in an ethanol/water mixture (with a water fraction of 90%) and used the well-known explosive, toxic and electron-deficient agent, PA, as the target.
As expected, the fluorescence of TPE-BOZ decreased gradually with the addition of incremental amounts of PA (Fig. 3a). Detectable emission quenching could be realized with PA concentrations as low as 10 nM, which quenched c. 4% of the initial emission intensity of TPE-BOZ. When 5 × 10−4 M PA was added to the probing platform, almost all of the emission of TPE-BOZ was quenched (Fig. 3a, inset). The quenching efficiency was evaluated by the slope of the linear region of the quenching plot (Fig. 3b) in the low concentration region (with PA concentrations <0.4 μM, ksv = 1.134 × 107 M−1). The detection limit c. 4.47 × 10−9 M (according to 3σ rule,51 y = 11.34x + 3.90, R2 = 0.9866) showed the high sensitivity of TPE-BOZ towards PA. In the UV-visible spectrum (Fig. 3c), the subsequent addition of PA to the solution of aggregates of TPE-BOZ resulted in an increase in the absorption band at 368 nm. There was an apparent colour change during the probing process. Fig. 3d shows that both TPE-BOZ and PA were colourless, whereas their mixture was pale yellow. Information about the preparation and performance of TPE-BOZ and some previously reported macromolecule-based PA probes are summarized in Table S2† to directly compare TPE-BOZ with other probes. Table S2† shows that TPE-BOZ had a detection limit that was comparable with the corresponding values of other probes and that the catalyst-free procedure and shorter polymerization time were preferable from the synthetic point of view.48,52–56
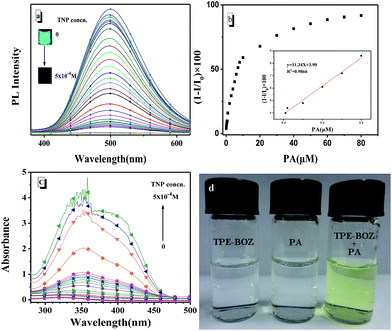 |
| Fig. 3 (a) PL intensity and (c) UV-visible spectra. (b) Relationship between (1 − I/I0) × 100 and [PA] (inset is the fitted curve with [PA] < 0.4 μM). (d) Photographs of TPE-BOZ (1.0 × 10−5 M) and PA in an EtOH : water mixture (1 : 9 v/v). | |
To evaluate the selectivity of TPE-BOZ towards PA, the change in emission caused by other nitro compounds (2,4-DNP, p-nitrophenol, o-nitrophenol, p-nitrotoluene, nitrobenzene and nitromethane and the referential agent, phenol) were investigated. PA showed remarkably more fluorescence quenching (Fig. 4a) than the other compounds. The further addition of PA to these systems containing background analytes resulted in quenching of the emission (Fig. 4b). For further comparison, the fluorescence quenching of TPE-BOZ by these compounds was evaluated at different concentrations of the quencher. Fig. 4c shows that although the fluorescence of TPE-BOZ could be partially quenched by other nitro aromatic compounds, the quenching efficiency was much smaller than that of PA in all instances. Fig. 4d shows that the degree of quenching reached a constant value (c. 97%) after incubating with 10 equiv. of PA for about 2 min.
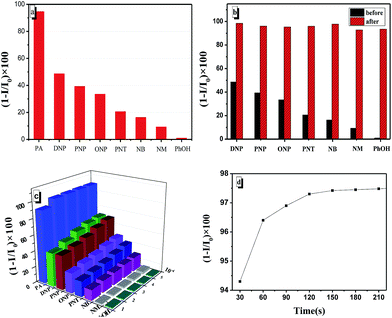 |
| Fig. 4 (a) Quenching efficiency of TPE-BOZ (1.0 × 10−5 M) for different analytes (1.0 × 10−4 M). (b) Quenching efficiency of TPE-BOZ (1.0 × 10−5 M) with background analytes (1.0 × 10−4 M) and with the successive addition of 1.0 × 10−4 M PA. (c) Concentration-dependent fluorescence quenching of TPE-BOZ (1.0 × 10−5 M) for different analytes. (d) Time-dependent fluorescence quenching of TPE-BOZ for PA (1.0 × 10−4 M) in EtOH : water (1 : 9 v/v). | |
The fluorescence alteration of TPE-BOZ in the presence of common solvents was also investigated. Acetonitrile, toluene, THF, dimethyl sulfoxide, N,N-dimethylformamide, dichloromethane, chloroform, ether, methyl alcohol, ethyl acetate and acetone were introduced as competing agents. As shown in Fig. 5, the addition of these solvents exerted a negligible influence on the fluorescence of TPE-BOZ and the following addition of PA caused remarkable fluorescence quenching in all instances. This shows that the determination of PA by TPE-BOZ was not significantly affected by the solvent residues in the probing medium.
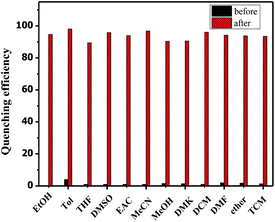 |
| Fig. 5 Quenching efficiency of TPE-BOZ (1.0 × 10−5 M) with different solvents (1.0 × 10−3 M) in EtOH : water (1 : 9 v/v) before and after the addition of PA (1.0 × 10−4 M). | |
Fluorescence quenching mechanism
To investigate the mechanism of fluorescence quenching of TPE-BOZ towards nitro compounds, a theoretical calculation using density functional theory with the B3LYP/DNP basis set using the DMol3 code was carried out. The highest occupied molecular orbital (HOMO) and lowest unoccupied molecular orbital (LUMO) levels of TPE-BOZ and the nitro compounds were calculated and the results are summarized in Table S1.† Fig. 6 shows the energy levels for the different molecules. As shown by the density functional theory calculation, the electrons in TPE-BOZ mainly spread on the TPE skeleton in both the HOMO and LUMO with values of −4.90 eV (HOMO) and −1.32 eV (LUMO). The energy gap (0.71 eV) between the HOMO of TPE-BOZ (−4.90 eV) the LUMO of PA (−4.19 eV) was smaller than the corresponding value (3.58 eV) between the HOMO (−4.90 eV) and LUMO (−1.32 eV) of TPE-BOZ, which supports electron transfer from TPE-BOZ to PA.57 In addition, the energy difference between the HOMO of TPE-BOZ and the LUMO of PA is smaller than the corresponding values between the HOMO of (TPE-BOZ) and the LUMO of other nitro compounds, showing the preference of TPE-BOZ for PA over other nitro compounds. The UV-visible spectra for the PA titration process in Fig. 3c show that the absorption intensity at c. 350 nm increased steadily with the introduction of incremental amounts of PA, with the onset of absorption extending to c. 450 nm. This is also a reflection of the electron transfer between TPE-BOZ and PA, as observed previously for the probing of PA using other chromophores.56 In addition, as reflected by the SEM image of TPE-BOZ (Fig. S7†), some small cavities were seen between the molecular aggregates. This could allow the small-sized PA molecules to enter and offer more diffusion channels for the excitons to migrate, allowing them to be more efficiently annihilated by the quenchers.58–60
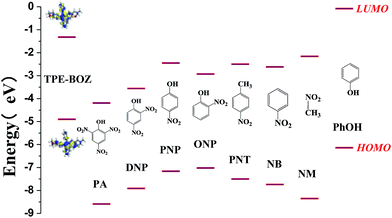 |
| Fig. 6 Graphical representation of the calculated energy levels of TPE-BOZ and other analytes. | |
This analysis theoretically supports the photo-induced electron transfer mechanism and we investigated whether the other mechanism (energy transfer between TPE-BOZ and PA) also contributed to fluorescence quenching. The absorption and fluorescence spectra of PA and TPE-BOZ were used to explore the possibility of energy transfer between TPE-BOZ and PA. Fig. 7 shows that the absorption of PA overlaps with the emission of TPE-BOZ and there is therefore a possibility of resonance energy transfer from fluorescent TPE-BOZ to the non-emissive PA.61
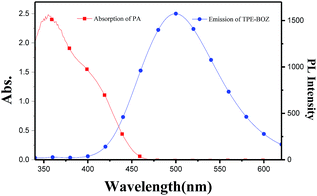 |
| Fig. 7 Spectral overlap of the absorption spectra of PA and the emission spectrum of TPE-BOZ in EtOH/water (v/v = 1/9) mixtures. | |
We carried out a fluorescence titration of TPE-BOZ with fixed amounts of PA at various excitation wavelengths (260–440 nm). The degree of quenching of PA was slightly affected by the excitation wavelength (Fig. S9†). This rules out the possibility of the decrease in the fluorescence intensities of TPE-BOZ being a result of masking by PA and experimentally supports the previously proposed energy transfer mechanism.61,62
On the basis of this discussion, we deduced that the specific selectivity and sensitivity of TPE-BOZ towards PA might be due to the synergetic effect of photo-induced electron transfer (PET) and energy transfer between TPE-BOZ and PA.
Visual detection of PA in the solid state
PA was also determined in the solid state. A coating of TPE-BOZ on a thin-layer chromatography plate showed a strong emission (Fig. S10a†). When drops of pure water (Fig. S10b†) and PA (Fig. S10c†) were placed separately on the coating of TPE-BOZ, the emission of TPE-BOZ was unaltered by the water, but significantly quenched by PA.
We also used test strips as a solid support to prepare a solid probe based on TPE-BOZ. Filter paper was cut into 2 cm2 pieces and dipped into an ethanolic solution (10−3 M) of TPE-BOZ for 10 min and were then dried under reduced pressure at 40 °C for 2 h. To investigate the sensitivity of the paper strip towards PA, different concentrations of PA were dropped onto the test strips. Fig. 8 shows that the fluorescence intensity in the spotted area decreased gradually with increasing concentrations of PA. These results support the suggestion that TPE-BOZ has the potential to be used as solid-state probe to determine PA.
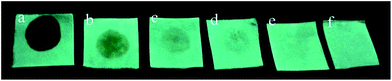 |
| Fig. 8 Paper strips of TPE-BOZ for the determination of PA under 365 nm UV light at different concentrations: (a) 1 × 10−3 M; (b) 1 × 10−4 M; (c) 1 × 10−6 M; (d) 1 × 10−7 M; (e) water; and (f) none. | |
Conclusion
Novel fluorescent benzoxazine derivatives were successfully synthesized by a catalyst-free method under mild reaction conditions. The corresponding benzoxazine-derived macromolecule (TPE-BOZ) had a good ability to form films and had AIEE characteristics in a mixture of ethanol (good solvent) and water (poor solvent). The optical response of TPE-BOZ towards PA was investigated to explore its potential application as an optical probe. TPE-BOZ showed sensitive fluorescence quenching towards PA in aqueous environments with a high water fraction (ethanol
:
water 1
:
9 v/v), which might be a result of photo-induced electron transfer and resonance energy transfer between TPE-BOZ (electron donor) and PA (electron acceptor). The degree of quenching of TPE-BOZ by PA was much higher than that of other nitro compounds and common solvents. This concept of a simple preparation method without the involvement of a catalyst is important in terms of the preparation of novel optoelectronic materials.
Acknowledgements
The authors greatly appreciate financial support from the National Natural Science Foundation of China (Project No. 21364013, 21262034). We acknowledge the National Supercomputing Center in Shenzhen for providing the computational resources and materials studio (version 7.0, DMol3 module).
Notes and references
- X. Chen, S. Fischer and Y. Men, Langmuir, 2011, 27, 12807–12814 CrossRef CAS PubMed.
- J. Dejeu, S. Diziain, C. Dange, F. Membrey, D. Charraut and A. Foissy, Langmuir, 2008, 24, 3090–3098 CrossRef CAS PubMed.
- A. Das and P. Theato, Chem. Rev., 2016, 116, 1434–1495 CrossRef CAS PubMed.
- A. van Dijken, J. J. Bastiaansen, N. M. Kiggen, B. M. Langeveld, C. Rothe, A. Monkman, I. Bach, P. Stossel and K. Brunner, J. Am. Chem. Soc., 2004, 126, 7718–7727 CrossRef CAS PubMed.
- E. Kim, S. Y. Cho, D. M. Yeu and S. Y. Shin, Chem. Mater., 2005, 17, 962–966 CrossRef CAS.
- Y. B. Chen, W. Shi, Y. H. Hui, X. Sun, L. X. Xu, L. Feng and Z. F. Xie, Talanta, 2015, 137, 38–42 CrossRef CAS PubMed.
- J. Milani, N. E. Pridmore, A. C. Whitwood, I. J. S. Fairlamb and R. N. Perutz, Organometallics, 2015, 34, 4376–4386 CrossRef CAS.
- N. Kutsumura, K. Niwa and T. Saito, Org. Lett., 2010, 12, 3316–3319 CrossRef CAS PubMed.
- S. R. Dubbaka and P. Vogel, J. Am. Chem. Soc., 2003, 125, 15292–15293 CrossRef CAS PubMed.
- A. Meyer, N. Spinelli, P. Dumy, J. J. Vasseur, F. Morvan and E. Defrancq, J. Org. Chem., 2010, 75, 3927–3930 CrossRef CAS PubMed.
- L. Trotochaud, T. J. Mills and S. W. Boettcher, J. Phys. Chem. Lett., 2013, 4, 931–935 CrossRef CAS PubMed.
- X. Q. Zhang, X. Y. Zhang, B. Yang and Y. Wei, Chin. J. Polym. Sci., 2014, 32, 1479–1488 CrossRef CAS.
- A. Gandini, Prog. Polym. Sci., 2013, 38, 1–29 CrossRef CAS.
- B. Yao, J. Mei, J. Li, J. Wang, H. Wu, J. Z. Sun, A. Qin and B. Z. Tang, Macromolecules, 2014, 47, 1325–1333 CrossRef CAS.
- S. Cheawchan, Y. Koyama, S. Uchida and T. Takata, Polymer, 2013, 54, 4501–4510 CrossRef CAS.
- J. Liu, X. Lu, Z. Xin and C. Zhou, Langmuir, 2013, 29, 411–416 CrossRef CAS PubMed.
- F. W. Holly and A. C. Cope, J. Am. Chem. Soc., 1944, 66, 1875–1879 CrossRef CAS.
- Y. Si, T. Ren, Y. Li, B. Ding and J. Yu, Carbon, 2012, 50, 5176–5185 CrossRef CAS.
- M. Ito, T. Kawauchi, K. Sakajiri and T. Takeichi, React. Funct. Polym., 2013, 73, 1223–1230 CrossRef CAS.
- S. Wang, W. C. Li, G. P. Hao, Y. Hao, Q. Sun, X. Q. Zhang and A. H. Lu, J. Am. Chem. Soc., 2011, 133, 15304–15307 CrossRef CAS PubMed.
- N. N. Ghosh, B. Kiskan and Y. Yagci, Prog. Polym. Sci., 2007, 32, 1344–1391 CrossRef CAS.
- K. Zhang, Q. Zhuang, X. Liu, G. Yang, R. Cai and Z. Han, Macromolecules, 2013, 46, 2696–2704 CrossRef CAS.
- B. Kiskan, N. N. Ghosh and Y. Yagci, Polym. Int., 2011, 60, 167–177 CrossRef CAS.
- F. Hong, C. Yan, Y. Si, J. He, J. Yu and B. Ding, ACS Appl. Mater. Interfaces, 2015, 7, 20200–20207 CAS.
- K. Chiou, P. Froimowicz, K. Landfester, A. Taden and H. Ishida, Macromolecules, 2014, 47, 3297–3305 CrossRef CAS.
- M. Arslan, B. Kiskan and Y. Yagci, Macromolecules, 2015, 48, 1329–1334 CrossRef CAS.
- H. A. Molla, R. Bhowmick, A. Katarkar, K. Chaudhuri, S. Gangopadhyay and M. Ali, Anal. Methods, 2015, 7, 5149–5156 RSC.
- H.-C. Liu, W.-C. Su and Y.-L. Liu, J. Mater. Chem., 2011, 21, 7182 RSC.
- C. H. Lin, Y. S. Shih, M. W. Wang, C. Y. Tseng, T. Y. Juang and C. F. Wang, RSC Adv., 2014, 4, 8692 RSC.
- S. S. Nagarkar, B. Joarder, A. K. Chaudhari, S. Mukherjee and S. K. Ghosh, Angew. Chem., Int. Ed. Engl., 2013, 52, 2881–2885 CrossRef CAS PubMed.
- Y. Peng, A. J. Zhang, M. Dong and Y. W. Wang, Chem. Commun., 2011, 47, 4505–4507 RSC.
- G. He, H. Peng, T. Liu, M. Yang, Y. Zhang and Y. Fang, J. Mater. Chem., 2009, 19, 7347 RSC.
- J. F. Wyman, M. P. Serve, D. W. Hobson, L. H. Lee and D. E. Uddin, J. Toxicol. Environ. Health, 1992, 37, 313–327 CrossRef CAS PubMed.
- S. J. Toal and W. C. Trogler, J. Mater. Chem., 2006, 16, 2871–2883 RSC.
- Y. Salinas, R. Martinez-Manez, M. D. Marcos, F. Sancenon, A. M. Costero, M. Parra and S. Gil, Chem. Soc. Rev., 2012, 41, 1261–1296 RSC.
- S. F. Hallowell, Talanta, 2001, 54, 447–458 CrossRef CAS PubMed.
- M. E. Germain and M. J. Knapp, Chem. Soc. Rev., 2009, 38, 2543–2555 RSC.
- T. J. B. Jeffrey, A. Caulfield and K. E. Miller, J. Chem. Eng. Data, 2009, 54, 1814–1822 CrossRef.
- Y. Hong, J. W. Y. Lam and B. Z. Tang, Chem. Commun., 2009, 32, 4332–4353 RSC.
- Y. Hong, J. W. Lam and B. Z. Tang, Chem. Soc. Rev., 2011, 40, 5361–5388 RSC.
- D. Ding, K. Li, B. Liu and B. Z. Tang, Acc. Chem. Res., 2013, 46, 2441–2453 CrossRef CAS PubMed.
- C. Yu, Y. Wu, F. Zeng, X. Li, J. Shi and S. Wu, Biomacromolecules, 2013, 14, 4507–4514 CrossRef CAS PubMed.
- J. Wang, T. T. Ren, Y. D. Wang, X. Y. He, W. B. Liu and X. D. Shen, React. Funct. Polym., 2014, 74, 22–30 CrossRef CAS.
- C. H. Lin, Y. S. Shih, M. W. Wang, C. Y. Tseng, T. C. Chen, H. C. Chang and T. Y. Juang, Polymer, 2014, 55, 1666–1673 CrossRef CAS.
- N. Xin and H. Ishida, J. Polym. Sci., Part A: Polym. Chem., 1994, 32, 1121–1129 CrossRef.
- T. Agag and T. Takeichi, Macromolecules, 2003, 36, 6010–6017 CrossRef CAS.
- J. Shi, X. Zheng, L. Xie, F. Cao, Y. Wu and W. Liu, Eur. Polym. J., 2013, 49, 4054–4061 CrossRef CAS.
- J. Wang, J. Mei, W. Yuan, P. Lu, A. Qin, J. Sun, Y. Ma and B. Z. Tang, J. Mater. Chem., 2011, 21, 4056 RSC.
- A. Qin, T. Li, J. W. Y. Lam, C. K. W. Jim, Y. Yong, Z. Hui, S. Jingzhi and T. Ben Zhong, Adv. Funct. Mater., 2009, 19, 1891–1900 CrossRef CAS.
- J. Liu, Y. Zhong, P. Lu, Y. Hong, J. W. Y. Lam, M. Faisal, Y. Yu, K. S. Wong and B. Z. Tang, Polym. Chem., 2010, 1, 426–429 RSC.
- V. Thomsen, D. Schatzlein and D. Mercuro, Spectroscopy, 2003, 18, 112–114 CAS.
- S. Mukherjee, A. V. Desai, B. Manna, A. I. Inamdar and S. K. Ghosh, Cryst. Growth Des., 2015, 15, 4627–4634 CAS.
- A. Chowdhury and P. S. Mukherjee, J. Org. Chem., 2015, 80, 4064–4075 CrossRef CAS PubMed.
- V. Bhalla, S. Kaur, V. Vij and M. Kumar, Inorg. Chem., 2013, 52, 4860–4865 CrossRef CAS PubMed.
- V. Bhalla, A. Gupta and M. Kumar, Org. Lett., 2012, 14, 3112–3115 CrossRef CAS PubMed.
- V. Vij, V. Bhalla and M. Kumar, ACS Appl. Mater. Interfaces, 2013, 5, 5373–5380 CAS.
- R. Chopra, P. Kaur and K. Singh, Anal. Chim. Acta, 2015, 864, 55–63 CrossRef CAS PubMed.
- Y. R. Ren, X. S. Jiang, G. L. Yin and J. Yin, J. Polym. Sci., Part A: Polym. Chem., 2010, 48, 327–335 CrossRef CAS.
- B. Yu, X. Jiang, G. Yin and J. Yin, J. Polym. Sci., Part A: Polym. Chem., 2010, 48, 4252–4261 CrossRef CAS.
- T. Agag, S. Geiger, S. M. Alhassan, S. Qutubuddin and H. Ishida, Macromolecules, 2010, 43, 7122–7127 CrossRef CAS.
- A. H. Malik, S. Hussain, A. Kalita and P. K. Iyer, ACS Appl. Mater. Interfaces, 2015, 7, 26968–26976 CAS.
- V. Bhalla, A. Gupta, M. Kumar, D. S. S. Rao and S. K. Prasad, ACS Appl. Mater. Interfaces, 2013, 5, 672–679 CAS.
Footnote |
† Electronic supplementary information (ESI) available. See DOI: 10.1039/c6ra06942h |
|
This journal is © The Royal Society of Chemistry 2016 |
Click here to see how this site uses Cookies. View our privacy policy here.