DOI:
10.1039/C6RA06933A
(Paper)
RSC Adv., 2016,
6, 62797-62809
Hydroxyapatite doped CeO2 nanoparticles: impact on biocompatibility and dye adsorption properties†
Received
16th March 2016
, Accepted 11th June 2016
First published on 15th June 2016
Abstract
The toxicity imparted by the commercialized use of nanoparticles in environmental remediation has become a big concern. To overcome this, the current work describes the fabrication of cerium oxide (CeO2) containing hydroxyapatite (HAp) nanoparticles. The toxicity of the system was tested against the Allium cepa anaphase–telophase chromosome aberration assay. The enhanced stability and biocompatible nature of HAp widen the scope of engineered nanoproducts for wastewater treatment. Anionic Eriochrome Black T (EBT) dye was chosen as a model system for analyzing the adsorptive behavior of the engineered nanoparticles. The meticulous analysis of surface morphology, elemental composition, size and functional groups over the engineered product were ascertained by using microscopic and spectroscopic techniques. The corresponding influence of methodological aspects such as experimental pH, time, and concentration of the toxins and the nanoadsorbate toward the removal efficiency of pollutant were also quantified by using UV-Vis spectroscopic measurements. The kinetic and equilibrium adsorption analysis were also performed and a probable adsorption mechanism was determined for the prepared materials. The prepared HAp/CeO2 nanohybrid can be exploited as a competent, low-cost adsorbent for the removal of dye molecules from the aqueous environment. This piece of work validates and promotes the idea of using biocompatible and non-toxic nanomaterials for environmental remediation, with high efficiency.
1. Introduction
The last decade has witnessed a growing curiosity of researchers for developing smart, efficient and biocompatible nanomaterials with a diverse range of technological and industrial applications.1–3 Out of various well-developed nanomaterials, cerium oxide (CeO2) has generated significant interest in the scientific community.4–7 CeO2 nanoparticles have displayed unique functions as antioxidant and radioprotective agent in cancer therapy.8 CeO2 nanoparticles also have significant applications in chemical mechanical planarization, fuel catalysis, paints, etc.,9 and play an important role as exogenous pro-resolving mediators in liver disease.10 Additionally, CeO2 nanoparticles have been used in developing anti-obesity pharmaceutical formulations.11 Selvaraj et al.12 have authenticated the role of CeO2 nanoparticles in the prevention of lipopolysaccharide induced sepsis in male Sprague Dawley rats. The rising appreciation for these CeO2 nanoparticles in industries has caused worry over their probable toxicity in the environment. In literature, there are several reports that specify the potentially lethal impacts of CeO2 nanoparticles towards bacteria, fish, and mammalian cells.13,14 The probable hazardous impact of CeO2 nanoparticles to plants has also been investigated by different groups. For instance, Zhang et al.15 have reported the effect of the accumulation of CeO2 nanoparticles in the roots of cucumber (Cucumis sativus L.)
Therefore, for the safe use of CeO2 nanoparticle based materials, caution must be exercised in determining the biocompatibility of these nanoparticles. In general, the biocompatibility of nanoparticles can be achieved by many methods, such as synthesizing nanoparticles from naturally biocompatible sources,16 doping,17 and coating the materials with biocompatible agents.18–21 Due to the scarcity of the natural availability of biocompatible sources and the less usefulness of doped systems,22,23 biocompatible coatings have an advantage in the synthesis of nanoparticles. The process of fabricating a biocompatible coating is comparatively easy and has minimum effects on the properties of the parent or core nanoparticles.24 Out of various available biocompatible agents, calcium phosphate based compounds, such as hydroxyapatite (HAp), dicalcium phosphate (BCP), tricalcium phosphate (α and β-TCP), have gained significant attention from researchers, due to their extensive applications in the fields of materials science, medicine and biology.25–27 Among these, HAp (Ca10(PO4)6(OH)2) has high stability in physiological conditions.28,29 HAp is widely employed in orthopedic,30 bone cementing,27 and bone tissue engineering.28 Currently, a composite coating of HAp over mesoporous silica nanoparticles has generated a significant role in drug delivery and also opposes unwanted bone resorption and speeds up fracture healing.31
The current work describes the fabrication of CeO2 nanoparticles containing hydroxyapatite (HAp) nanoparticles in aqueous media. For the sake of comparison, the synthesis of the HAp–CeO2 nanocomposite was also done in the presence of four different cationic surfactants and commercially available CeO2 powder. The toxicity of all the studied systems was tested against the Allium cepa anaphase–telophase chromosome aberration assay. The seed germination experiments for wheat were also carried out to analyse the effect of nanoparticles on the growth inhibition rate. The enhanced stability and biocompatible nature of HAp widens the scope of CeO2 nanoparticles for wastewater treatment. Anionic Eriochrome Black T (EBT) dye was chosen as a model system for analyzing the adsorptive behavior of CeO2 nanoparticles. The meticulous analysis of surface morphology, elemental composition, size and functional groups were carried out by using microscopic and spectroscopic techniques. The as prepared nanohybrid acts as a competent, low-cost adsorbent for the adsorptive removal of dye from the aqueous environment with great efficiency.
2. Experimental
2.1. Materials
Cerium(III) nitrate hexahydrate (99%), cerium oxide powder (99.99%), calcium hydroxide (≥95.0%), o-phosphoric acid, CTAB (hexadecyl trimethyl ammonium bromide), CTAC (hexadecyl trimethyl ammonium chloride), CPB (cetyl pyridinium bromide hydrate 98%), CPC (cetyl pyridinium chloride), acetocarmine and mercury chloride were purchased from Sigma Aldrich. NH4OH (ammonia solution, ∼25%) was purchased from Merck. Absolute ethanol was obtained from Changshu Yangyuan Chemicals, China. Cationic Eriochrome Black T (EBT) was chosen as the adsorbate dye for evaluating the selective adsorption behavior over the as prepared nano hybrid of CeO2/HAp. All the chemicals were used as purchased without any further purification. The standardization experiments were conducted using double distilled water, and real sample analysis was done on water collected from a hand pump; the Western Yamuna canal, Rohtak Division; tube well; tap and well water from Kiloi village, District Rohtak Haryana, India.
2.2. Synthesis of CeO2 nanoparticles
2.2.1. Bare and surfactant-modified CeO2 nanoparticles. Cerium nitrate hexahydrate was chosen as a starting material for the preparation of CeO2 nanoparticles. In a typical experiment, 0.03 M cerium nitrate hexahydrate (Ce(NO3)3·6H2O) was dissolved in 50 ml of ethanol. About 25% aqueous solution of NH4OH was added to the above solution in a drop-wise manner. The obtained mixture was aged for 24 hours. The resultant yellow dispersion was centrifuged and properly washed, dried and collected for further analysis. For preparing surfactant modified CeO2 nanoparticles, the corresponding aqueous precipitation method was used.32 The synthesis was similar to that explained for bare nanoparticles; the only difference was the addition of 0.03 M Ce(NO3)3·6H2O in 0.05 M aqueous surfactant solution of CTAB, CTAC, CPC and CPB, followed by the addition of 1.3 M ethanol, and 0.25 M NH4OH in 50 ml distilled water.
2.2.2. Impregnation of hydroxyapatite (HAp) nanoparticles over CeO2 nanostructures. HAp loaded bare CeO2 nanoparticles and surfactant functionalized CeO2 nanoparticles were synthesized by the impregnation method. Briefly, a suspension of Ca(OH)2 and CeO2 nanoparticles (synthesized previously) was mixed in water under stirring conditions. The obtained suspension was subsequently heated to 80 °C under stirring. About 0.75 M of o-phosphoric acid (80%) was then added dropwise to the mixture. The obtained suspension was further kept under stirring for 2 h at 80 °C. The formed mixture contained HAp loaded CeO2 nanoparticles. The resulting precipitates were separated from the solution by centrifugation and then washed with distilled water, followed by ethanol to removal all ionic impurities and then dried at 70 °C.
2.3. Allium cepa chromosome aberration assays
The presence of different types of chemical agents and nanoparticles has the ability to damage the chromosomal DNA of the living species. The variations further lead to genetic or mutagenic disorders in living organisms and lead to serious health hazards.33 Therefore, the assessment of genotoxicity of the as prepared nanomaterials is essential for ensuring their use in environmental remediation, without causing damage to the biota.
In the current study, Allium cepa (onion) chromosomal aberration assay was used for analyzing the genotoxic effect of bare and functionalized CeO2 nanoparticles. For the sake of comparison of toxicity generated by bulk and nanometric particles, trials with commercial CeO2 powder have also been carried out. For experimentation, onion bulbs were cultivated without the application of any external herbicides or fungicides in the growing media. The bulbs were scraped from the root to facilitate the emergence of new roots. To obtain the rootlets, the onions were allowed to grow in 100 ml solutions containing 100 ppm of different types of nanoparticles and CeO2 powder for 3–4 days, or until 2–3 cm rootlets emerged. Water was used as a negative control in the subsequent analysis. In order to exclude the effect of onion quality, 5 groups of Allium cepa were used for each treatment of the as fabricated nanoparticles.
The representative roots of 1 cm length were cut by using sterilized scissors, and transferred to a plastic microtube containing 1 N HCl. The resultant was then incubated at 60 °C for 12 minutes over a water bath. The obtained roots were then carefully transferred to a Petri dish and washed with distilled water. The root tips were stained with acetocarmine dye for the specific visualization of chromosomal aberrations in Allium cepa. Slides were prepared by cutting the stained root tip over glass slides. The cover slip was gently placed over the slide and tapped to spread the cells and viewed under an electron microscope. The corresponding value of mitotic index (MI) was calculated according to following equation:
|
 | (1) |
where P + M + A + T = total number of cells in Prophase, Metaphase, Anaphase and Telophase, respectively. The relative mitotic index (RMI) and the chromosomal aberration index (CAI) were also calculated in accordance with
eqn (2) and
(3):
|
 | (2) |
where MI is the mitotic index for each treatment and MI
C is the MI value of the control process.
|
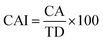 | (3) |
where CA is the number of divisions with chromosomal aberrations and TD is the total number of divisions. All the calculations were made for 5 groups and the average values were taken with respective standard deviations.
2.4. Seed germination bioassay
The inhibitory effect of CeO2 nanoparticles and commercially available CeO2 powder on wheat seed germination has been used to monitor the cytotoxic and anti-proliferative effects in the presence of nanoparticles. The main objective of this study is to provide an alternative to the animal or human bioassay model.34 The presence of nuclei in plants makes them comparable to animal and human cells; as a result, the variations in the biochemical expression of plants in the presence of different analytes can be correlated, to a great extent, with animals as well as humans beings.
2.4.1. Experiment plant (Triticum aestivum). Best quality wheat (Triticum aestivum) seeds of uniform weight (55 ± 4 mg), were purchased from the grain market in Chandigarh, India. The seeds were subjected to viability testing by submerging them in water and the floating seeds were discarded. The submerged seeds were dried and then sterilized with 0.1% mercuric chloride solution for 5 minutes, followed by 5–6 washings with distilled water.The as dried seeds were soaked in 30 ml of distilled water for the control, and in an aqueous solution of nanoparticles and CeO2 powder for 48 h. The seeds were then transferred to Petri dishes with their respective solutions. The seeds were allowed to grow for 4 days under dark and warm conditions. The sprout length was measured with thread. All experiments were carried out in triplicate and the average was taken for further calculations. The obtained results were expressed in terms of percentage inhibition growth (PIG).
|
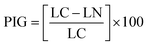 | (4) |
where, LC is the average length in the control and LN is the average length in the nanoparticles solution.
3. Characterizations
The optical properties of the as-prepared nanoparticles were analyzed by using a LABINDIA UV-Vis spectrophotometer. The fluorescence analysis was done by using a Hitachi F-7000 spectrophotometer. The crystallographic structural analysis was carried out on a Panalytical D/Max-2500 powder diffractometer with monochromatic CuKα radiation (λ = 1.5406 Å) over the 2θ range of 5–90° at a scan rate of 2 deg min−1. The operational voltage and the currents were 40 kV and 30 mA, respectively. Fourier transform infrared (FT-IR) spectroscopy (Perkin-Elmer (RX1)) was used to identify the functional groups in the working range of 4000–400 cm−1, with 2 cm−1 resolution, averaging 100 scans. The morphological analysis was carried out by TEM (Hitachi (H-7500)) and SEM (Hitachi (SU8010)) analysis. The measurements of particle size (90° optics) and zeta potential studies were done on a Nano S 90 ((Red badge), Malvern Instruments Corporation, model no. ZEN 1690). The thermal analysis of the as-prepared nanoparticles was done with a TG–DTG (thermogravimetric analysis) on SDT Q-600, from TA Instruments. The surface area of the as prepared nanoparticles was estimated by using BET analysis with N2 adsorption analyzer (NOVA 2000e) USA. Chromosomal aberration experiments were carried out using a Nikon Eclipse E-200 light microscope with an attached camera. The images were visualized and captured at 100× magnification by using acetocarmine stain. The loading of the EBT dye molecules over the nanoparticles in adsorption experiments were analyzed by elemental mapping through SEM analysis.
4. Results and discussion
4.1. Structural characterization
In the current work, hydroxyapatite (HAp) was chosen as a safe alternative for synthesizing biocompatible CeO2 nanoparticles in aqueous media. The structural evolution of the as obtained particles was carried out by a series of experiments. The resultant morphologies of pure HAp and HAp–CeO2 were investigated by SEM and TEM analysis (Fig. 1).
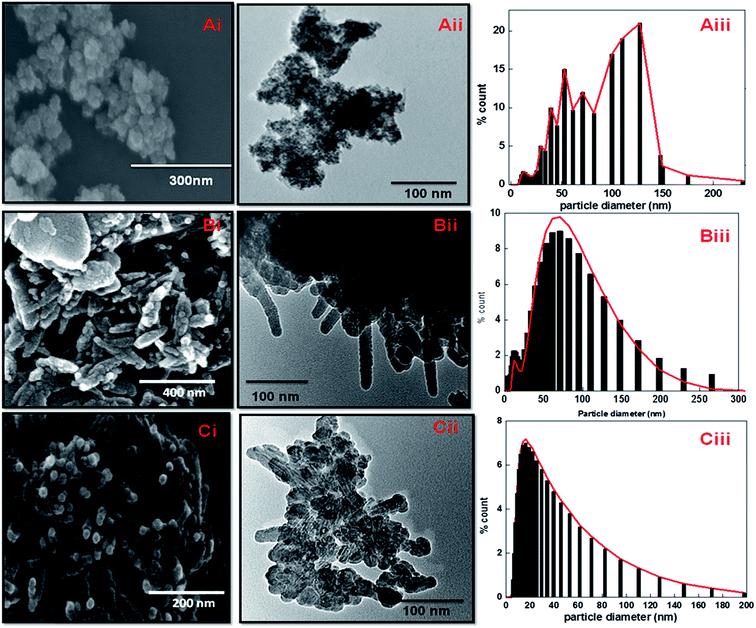 |
| Fig. 1 FESEM, TEM and particle size distribution of (Ai–Aiii) bare CeO2, (Bi–Biii) pure HAp and (Ci–Ciii) HAp coated CeO2 nanoparticles. | |
The pure HAp nanoparticles show irregular, rod-like structures with length of around 50–100 nm. In the presence of CeO2, the particles appeared to be less distorted and the obtained size was lower than that of the pure HAp nanoparticles. The bare CeO2 nanoparticles also show the higher rate of agglomeration. The obtained values for aggregation size of bare CeO2, pure HAp and HAp coated nanoparticles were 80 nm, 96 nm and 76 nm, respectively. The obtained surface area for bare CeO2, pure HAp and HAp coated nanoparticles were 9.15 m2 g−1, 6.03 m2 g−1 and 7.65 m2 g−1, respectively. The respective elemental mapping of HAp coated CeO2 nanoparticles are shown in Fig. 2. The irregularity in pure HAp was associated with the quick interaction of freely available Ca2+ ions with PO43− ions. This leads to a higher precipitation rate of HAp in reaction media. On the other hand, in the presence of CeO2 nanoparticles, the corresponding rate of interaction between the two ions was decreased and led to the confined growth of HAp nanoparticles.
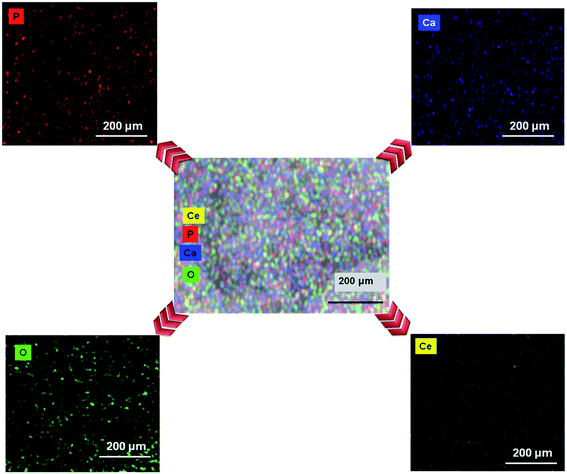 |
| Fig. 2 The elemental mapping of HAp coated CeO2 nanoparticles, showing the individual and combined presence of Ce, O, P and Ca atoms in the system. | |
The shape of the obtained nanoparticles was further reformed in the presence of HAp nanoparticles over surfactant capped CeO2 nanoparticles (Fig. 3). The surfactant chosen was cationic in nature, which enhances the adsorption of negatively charged dye used in this work. The four types of surfactants used in this work have the same chain length, but differ in the counterions, as well as the head groups of the surfactant. Therefore, the current work gives the detailed comparison of the effect of surface coating on the dye removal efficiency in the presence and absence of HAp. Moreover, the presence of surfactant template also influenced the interaction rate of Ca2+ ions with PO43− ions.
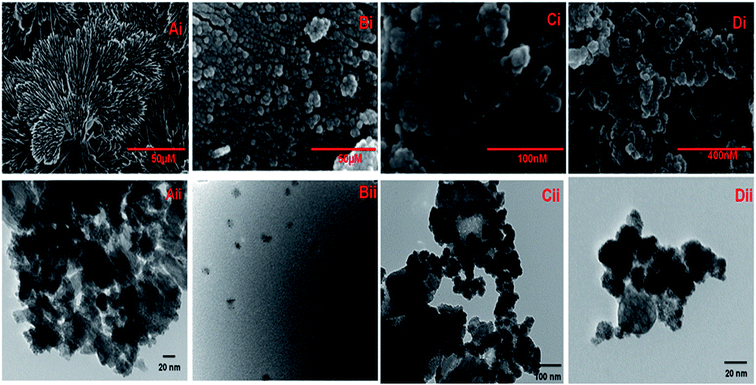 |
| Fig. 3 The corresponding FESEM and TEM images of (Ai and Aii) CTAB, (Bi and Bii) CTAC, (Ci and Cii) CPB and (Di and Dii) CPC functionalized CeO2 nanoparticles in the presence of HAp. | |
The rod-shaped particles were less abundant in the presence of surfactant capped CeO2 nanoparticles. The sharp multiple fiber-like morphology was obtained from the central point in the case of HAp coated CTAB functionalized CeO2 nanoparticles (Fig. 3Ai). The corresponding length of such structures varied at around 500 nm with the corresponding width of less than 20 nm. The close inspection of the TEM image (Fig. 3Aii) showed the presence of two well-defined types of particles. One depicted the presence of small sized CeO2 nanoparticles (dark contrast) over the surface of HAp nanoparticles (low contrast); the variations were associated with the directional growth of HAp in the presence of functionalized nanoparticles. However, in the case of CTAC, CPC and CPB functionalized CeO2 nanoparticles, there was the abundance of more spherical particles. The obtained particles showed more uniformity in the structures. These behavioral changes are mainly associated with the selective growth of nanoparticles along the preferential crystallographic direction in the presence of surfactants.32 The corresponding particle diameter frequency distribution plots for HAp–CeO2 nanoparticles in the presence of surfactants are shown in Fig. S1 (ESI†). The EDS elemental analysis was also carried out to check the purity and stoichiometry of the formed nanoparticles (Fig. S2 (ESI†)).
The corresponding changes in the morphological structures were further investigated through FTIR analysis (Fig. 4). The pure HAp nanoparticles show the characteristic peaks of the phosphate group. The asymmetric stretching peak for the P–O band was observed at 1066 cm−1. The respective O–P–O, P–H and P
O bands appeared between 560 and 500, 2340 and 1140 cm−1. The hydroxyl band was evident at 3400 cm−1. The absorption peak below 700 cm−1 corresponds to the Ce–O peaks in the case of HAp–CeO2 nanoparticles.32 The P–O band appeared at 1072 cm−1 in the presence of CeO2, and was also blue shifted by 13 cm−1. The marked differences are detected between 600 and 400 cm−1 in the presence of bare and surfactant functionalized CeO2 nanoparticles. The intensities of the peaks at 558 and 520 cm−1 also show variations. Such changes are associated with the difference in the crystalline growth of HAp nanoparticles in different reaction media.
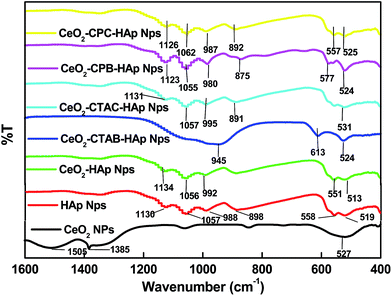 |
| Fig. 4 FTIR spectra of HAp nanoparticles synthesized in the absence and presence of bare and surfactant functionalized CeO2 nanoparticles. | |
In order to determine the growth mechanism of HAp in the presence of CeO2 nanoparticles, the respective phase analysis of particles was conducted using the XRD studies. Fig. 5 shows the typical XRD profile for the as obtained nanoparticles. The strong intense peaks at 2θ = 26.67°, 30.36°, 32.05°, 32.86° and 33.05° correspond to the 〈002〉, 〈211〉, 〈112〉, 〈300〉 and 〈202〉 planes of the crystalline structure for bare HAp nanoparticles.34 The obtained results were comparable to the database of the Joint Committee on Powder Diffraction Standards (JCPDS) card number: 9-432. The XRD spectra of bare CeO2 nanoparticles displayed characteristic peaks at 28.4° and 47.5°. On another hand, the XRD profiles of HAp coated CeO2 nanoparticles in the presence and absence of surfactants show relatively broader peaks. The intensities of the peaks also show significant variations in the presence of surfactant modified CeO2 nanoparticles. The corresponding comparison for the peak intensities of 3 planes, i.e. 〈002〉, 〈211〉 and 〈112〉 have been tabulated in Table S1 (ESI†). An estimation related to the crystallite size for nanoparticles, according to Scherrer's formula35,36 further reveals a proportional increase in the crystallite size of pure HAp nanoparticles, as compared to HAp coated CeO2 nanoparticles, in the presence and absence of surfactants (Table S1†).
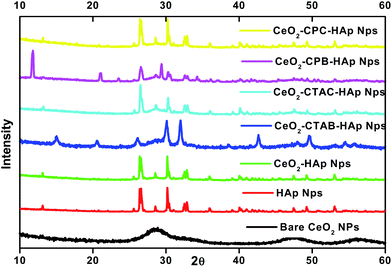 |
| Fig. 5 XRD spectra of pure HAp nanoparticles, HAp coated CeO2 nanoparticles, HAp coated CTAB–CeO2 nanoparticles, HAp coated CTAC–CeO2 nanoparticles, HAp coated CPB–CeO2 nanoparticles and HAp coated CPC–CeO2 nanoparticles. | |
From the data, it has been anticipated that the intensity ratio of the peaks at the 〈211〉 and 〈002〉 planes was more in the case of HAp coated CTAB and CPB functionalized CeO2 nanoparticles. This variation explained the greater crystalline growth of HAp in the 〈211〉 plane, as compared to the 〈002〉 plane. On the other hand, pure HAp, HAp coated CeO2 in the absence of surfactants, and in the presence of CPC, show equivalence in intensity ratio. The results were interpreted via the slower diffusion of PO43− ions in the presence of surfactants; the presence of surfactants over the surface of CeO2 nanoparticles acts as a hindrance barrier for the PO43− and Ca2+ ions. The presence of surfactants in the reaction media further lowers the addition rate of PO43− ions over the Ca2+ ions. This decrease in diffusion rate enhances the crystalline growth of nanoparticles in a preferential direction. The presence of N+ charge in the head group of surfactants causes the formation of bonds with PO43− ions. Such electrostatic interactions provided the additional alignment of growing nanoparticles along the specified axis during crystal growth of HAp in the presence of surfactants. The corresponding ε (strain), σ (stress) and μ (energy density) for the as functionalized nanoparticles have also been calculated by using well defined Williamson–Hall methods.37 The used value of Young's modulus of elasticity was taken as 3.9 GPa for the current study.38 The obtained results are tabulated in Table S1 (ESI†). Fig. S3† has shown the comparative thermal gravimetric analysis for pure HAp, CeO2 and CeO2 impregnated HAp nanoparticles. In the spectra, the small weight losses up to 200 °C correspond to the evaporation of residual water or moisture from the as formed nanoparticles. In the case of pure HAp nanoparticles, the first 10% weight loss was associated with the dehydration of HPO42− ions to P2O72− ions; whereas, surfactant modified CeO2 in the absence and presence of HAp possess two main weight losses in the temperature range of 300–500 °C. These losses were associated with the exclusion of surfactant templates, followed by the HAp layer from the exterior surface of CeO2 nanoparticles. The optical and luminescence properties of as-synthesized nanoparticles were also analyzed using UV-Vis and fluorescence studies (Fig. S4, ESI†). The photoluminescence (PL) spectrum of the as-synthesized nanoparticles was measured by exciting the samples with λ = 290 nm (Fig. S4a†). All the water dispersed solutions containing CeO2 nanoparticles have shown a blue emission peak in the range of 350 to 410 nm. These peaks were attributed to the surface defects and dislocations, which are helpful for fast oxygen transportation in CeO2 nanoparticles.32 In the case of pure HAp, no such peak was visible in this range. In the presence of HAp over CeO2 nanoparticles, there was reduced luminescence intensity for this peak. The intensity variation over this peak in the presence of HAp with CeO2 was related to the suppression of surface defects of CeO2. These results were further verified by carrying out the UV-Vis analysis of as prepared samples in the presence and absence of HAp (Fig. S4b†). These shifts in the peaks were mainly associated with the suppression of surface defects of CeO2. Moreover, the size variation was also responsible for the changes in the peak positions of the UV-Vis spectra.
4.2. Allium cepa chromosome aberration assays
The genotoxic and mutagenic effects of harmful toxins have also been determined from their damaging impact on the DNA. From the literature, it is quite evident that nanoparticles have the tendency to trigger free radical formation in cells.39 The small size of the particles support their diffusion inside the cell wall, or these particles can be actively taken up by phagocytosis.40 The formation of reactive oxygen species, which are mainly responsible for the disruption of cell function, was mainly dependent on the particle size and structure. In the current work, genotoxic studies of the as formed nanoparticles and commercially available CeO2 powder (1000 ppm) were carried out by using Allium cepa. Fig. 6a and b show the results of the different percentages of cells in the prophase, metaphase, anaphase and telophase of Allium cepa after treating it with different nano formulations. The corresponding results of % mitotic index and % relative mitotic index values are presented in Fig. 6c and d in the presence and absence of HAp. The respective chromosomal aberration index values are presented in Table S2 (ESI†). The chromosome aberration indices were absent in the case of control bulbs (Fig. 7). The observed mitotic index value for the blank was 80.36%. On the other hand, bare CeO2 nanoparticles showed an increased number of aberrations with a mitotic index value of 18.5% (Fig. 8).
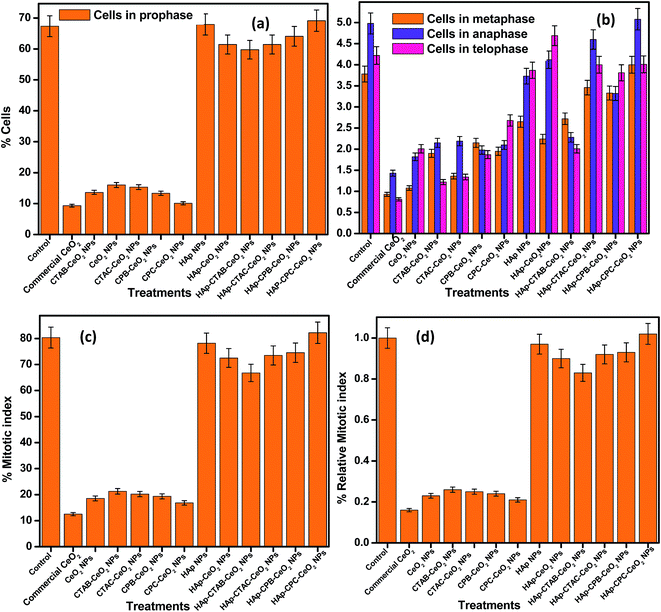 |
| Fig. 6 The variation of percentage cell values as a function of (a) cells in prophase, (b) cells in metaphase, anaphase, telophase (c) percentage mitotic index and (d) relative mitotic index for control, pure HAp and bare and surface functionalized CeO2 nanoparticles in the absence and presence of HAp coating, with concentration equivalent to 1000 ppm. | |
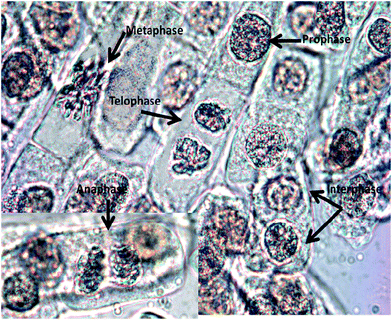 |
| Fig. 7 Different stages of mitotic division such as interphase, prophase, metaphase, anaphase and telophase in control treatment. | |
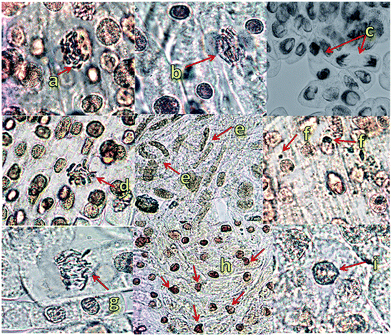 |
| Fig. 8 Chromosomal aberrations observed in Allium cepa root tips in the presence of CeO2 nanoparticles (a) clumped chromosomes, (b) anaphase bridge, (c) disturbed anaphase, (d) sticky chromosomes, (e) laggard chromosomes, (f) micronuclei formation, (g) disturbed metaphase (h) nuclear notch and (i) binucleate cell. | |
The chromosome aberration indices were also evident in the presence of surfactant capping over the CeO2 nanoparticles. The respective MI values varied between 16.8 and 21.8% (Fig. 6c) for surfactant functionalized CeO2 nanoparticles. Alternatively, the nano formulations containing HAp at low concentrations generated less chromosomal aberrations, compared to bare and surfactant coated CeO2, signifying that the association of the biocompatible nanoparticles with the system was able to reduce the harm to the DNA of the Allium cepa cells. However, the coating of HAp over bare CeO2 nanoparticles also displayed less chromosomal aberrations with MI values of 72.5%, as compared to the 18.5% for bare CeO2 in absence of HAp. The MI values of surfactant functionalized CeO2 nanoparticles have also shown significant improvement in the presence of HAp. The CeO2 nanoparticles have a smaller size, compared to HAp doped CeO2 nanoparticles, which leads to the larger production of ROS by the un-doped CeO2 nanoparticles.40 Due to the larger surface area to mass ratio of smaller particles, more ROS is formed.41 The HAp grafting over the CeO2 nanoparticles surface blocks the nanoparticle surface–cytosol interaction and creates a steric barrier. Also, the biomimetic nature of HAp avoids the interruption of redox homeostasis of the cell by HAp doped CeO2 nanoparticles, thus reducing its genotoxicity. The highest toxicity imparted by bulk CeO2 powder could be related to the extra damage caused to cells, due to the irregular shape and huge size of the particles. These particles could also block the cell wall channels and inhibit the proper functioning and proliferation of the cells. The results clearly indicate the utilities of developed HAp formulations with minimum damage to the environment.
4.3. Seed germination assay
The antiproliferative activity of the as formed nanoparticles with 1000 ppm concentration showed higher PIG values, ranging from 96.16 to 100% (Fig. 9 and Table S3†), whereas, at the same concentration the HAp coated nanoparticles showed 0.00 to 7.7% PIG. These results have clearly indicated that there was a huge difference in the antiproliferative activity of CeO2 nanoparticles in the absence and presence of HAp coating. The reason could be assigned to the biocompatible nature of HAp and the size enhancement with HAp coating over the surface of nanoparticles (Fig. S5†).
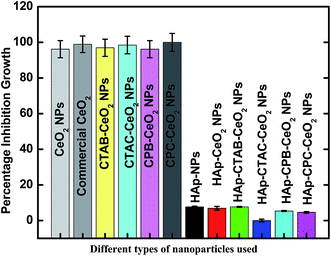 |
| Fig. 9 The percentage inhibition growth of Triticum aestivum in the presence of different nanoparticles. | |
4.4. Adsorption studies
The characteristic properties of nano-adsorbents, including the nature of functionalization, size and their interaction efficacy with the adsorbates, are the principal factors that govern the adsorptive removal efficiency of the system.32b The HAp functionalized CeO2 nanoparticles in the absence and presence of surfactants have also been tested for their sorption capacity against Eriochrome Black T (EBT) dye as a model system in this work. The adsorption of dye molecules has been achieved via the electrostatic interactions between the surface functionalized nanoparticles and dye molecules. The presence of surfactants over the surface of nanoparticles has further enhanced the adsorption efficiency of the nanoparticles by modulating their size and also through aromatic interactions between the head groups of surfactants and hydrophobic dye molecules. The batch experiments for the adsorptive removal of Eriochrome Black T (EBT) from its aqueous solution were conducted with all the prepared nano-formulations. The optimization of the data was done by elucidating the influence of different parameters, including nanoparticle dosage amount, initial dye concentration, pH and contact time for all the respective systems. The digital imaging of HAp–CTAB coated CeO2 nanoparticles as a function of pH, nanoparticles dosage amount and contact time is shown in Fig. S6 (ESI†).
4.4.1. Influence of pH. The chemical interactions of dye in nano-formulations and the respective ionization behavior of sorbate (dye) and sorbents are influenced by the pH of the reaction media. The zeta potential measurements for the as synthesized nanoparticles were also done to find the exact mechanism of dye adsorption over the nanoparticles. For this purpose, 5 mg of each type of nanoparticles were dispersed in 5 ml of buffer solution using an ultrasonic bath. Afterwards the nanoparticles were centrifuged and redispersed in 5 ml of triply distilled water. Different pH values of 2, 4, 6, 7, 8, 10 and 12 were maintained using 1 N HCl and 1 N NaOH. At low pH values, the nanoparticles possess positive values of zeta potential, due to the adsorption of H+ ions from aqueous media. At high pH values, the surface of the nanoparticles has the tendency to adsorb OH− ion and possess negative zeta potential values. Fig. S7† shows the variation of zeta potential values as a function of pH for different types of nanoparticles under study. For bare CeO2 nanoparticles, the pHPZC (zero point charge value) lies between 6.37 and 6.56, whereas, HAp functionalized nanoparticles have pHPZC at ∼6.46 to 6.6. This similarity in pHPZC with and without functionalization enabled us to carry out adsorption studies for all these nanoparticles at the same pH value (Table S4†). Therefore, in the current study, the working pH for EBT adsorption was taken as 5. In the case of surfactant coating along with HAp functionalization, there was a higher value of pHPZC. This behavioral variation was the main reason for the higher adsorption efficiency of EBT dye over surfactant-coated nanoparticles at low pH values.The adsorption efficiency of EBT on different nano formulations was tested as a function of pH variations. The adsorption batches were carried with 50 ppm of EBT dye in 50 ppm concentration of nanoparticles in the pH range from 2–12 at room temperature (Fig. 10a). The corresponding effect of bare CeO2 nanoparticles as a function of pH is included in Fig. S8.† The supernatant and solid residues were separated by centrifugation at 4000 rpm for 10 min. The concentration of the dye was investigated by measuring the absorption value at λmax = 520 nm.
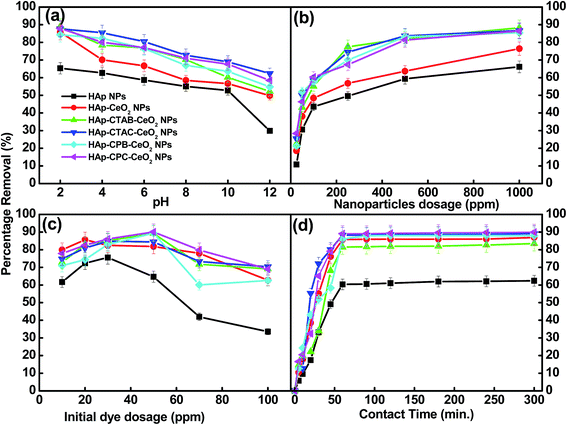 |
| Fig. 10 Effects of different parameters on the dye removal efficiency of pure HAp and bare and surface functionalized CeO2 nanoparticles in the absence and presence of HAP coating: (a) pH, (b) nanoparticles dosage, (c) initial dye dosage and (d) contact time. | |
The percentage removal of EBT dye was calculated by using the following relationship:
|
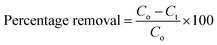 | (5) |
where
Co is the initial concentration of dye in ppm and
Ct is the equilibrium sorbate concentration. From
Fig. 10a, it is evident that the uptake of EBT decreased as the pH of the solution increased in the reaction media. The behavioral changes were associated with protonation of the phosphate groups present over the surface of the HAp coated nanoparticles in acidic media (pH = 2–5). The as prepared H
2PO
4− ions further facilitated the binding of anionic EBT dye with nanoparticles. On further increasing the pH from 6 to 7, the formation efficiency of HPO
42− was affected, which further contributed to the decrease in the adsorptive removal of dye molecules from aqueous media. On further increasing the pH to 12, the deprotonated form of the phosphate group
i.e. PO
43− was formed, which showed highly repulsive behavior towards the anionic dye, EBT. These results further suggested that the maximum adsorptive removal efficiency of EBT was achieved at pH = 5, and this pH was further employed to optimize other parameters for dye removal applications.
4.4.2. Influence of adsorbent dosage. The respective amount of sorbent employed to remove dye molecules from its per unit volume solution is a significant parameter to check the economics of the prepared method.32b The corresponding plots of adsorptive removal efficiency vs. adsorbent dosages at room temperature for different nanoformulations are presented in Fig. 10b with fixed dye concentration of 50 ppm at pH = 5. As evident for the fixed concentration of dyes, the adsorption capacity varied linearly with the amount of adsorbate added in the reaction system. These results were explained on the basis of higher available surface area and respective enhanced adsorption sites for the removal of dye molecules.
4.4.3. Effect of initial dye concentration. The effect of different initial dye dosages (i.e. 10, 20, 30, 50, 70 and 100 ppm) was also tested against the nanoparticle dosage amount of 50 ppm at pH = 5 for all the prepared nanoparticles (Fig. 10c). The percentage removal efficiency of EBT increased with increase in EBT concentration until a saturation point of 50 ppm was attained. Beyond this concentration, the percentage removal of EBT decreased due to the unavailability of vacant adsorption sites over the surface of the nanoparticles.
4.4.4. Effect of contact time. The corresponding influence of the adsorptive removal efficiency of EBT dye over the surface of the nanoparticles was also tested as a function of contact time (Fig. 10d) in the presence of 100 ppm of nanoparticles and 50 ppm EBT dye at pH = 5. As evident from the results, the removal efficiency of the dye shows a linear increase with increasing contact time. The distinct increasing percentage removal efficiency occurred up to 60 min and attained constancy after 1 hour. These findings further confirmed that a minimum contact time of 60 minutes was sufficient to effectively remove more than 95% dye from its aqueous media.
4.5. Adsorption isotherms
The respective interactional behavior of dye molecules with nanoparticles at a given temperature under equilibrium conditions was further investigated by isothermal analysis.
The equilibrium adsorption data of the EBT dye was analyzed by applying the well acknowledged Langmuir and Freundlich model. The linearized form of the Langmuir isotherm is expressed as follows:
|
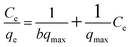 | (6) |
where
Ce is the equilibrium concentration of dye,
qe the amount of dye adsorbed (mol g
−1),
qmax and
b are Langmuir constants related to the adsorption capacity (mol g
−1) and energy (L mol
−1), respectively (Fig. S9
†). The Langmuir model assumes the monolayer adsorption of dye molecules (
i.e. one molecule thick adsorbed layer) at a fixed number of localized sites over nanoparticles. This model has also assumed that no lateral interaction and hindrance between the adsorbed molecules occurred with adjacent sites over adsorbate. The obtained values of
qmax and
R2 for different nanoparticles are tabulated in Table S5.
† On the other hand, the Freundlich equation was based on a heterogeneous surface:
|
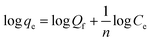 | (7) |
where
Qf and
n are empirical constants and their values are obtained by plotting log
qe vs. Ce. The value of the log
Qf is obtained from the intercept and
n is the slope of linear fitting (Fig. S10
†). The assessment of estimated coefficients (
R2) of EBT indicates that adsorption over HAp in the absence and the presence of CeO
2 nanoparticles can be better explained by the Freundlich isotherm equation, which suggests the multilayer formation of dyes over the surface of the nanoparticles. In addition, the higher values of
n greater then unity further support the interaction of dye molecules with surface nanoparticles. The elemental mapping of dye-coated HAp impregnated CTAC–CeO
2 nanoparticles has also confirmed the surface adsorption of EBT dye over the surface of the nanoparticles (
Fig. 11). Single element mapping of Ce and O has shown the small sized CeO
2 nanoparticles; the appearance of Ca and P in agglomerated and large sized particles confirms the encapsulation of CeO
2 nanoparticles by HAp. The abundance of the pink color that is formed due to the collective effect of S (yellow) and N (purple) is proof of the surface adsorption of EBT dye on HAp–CTAC–CeO
2 nanoparticles. Similar results were obtained for other synthesized nanoparticles (results not shown here).
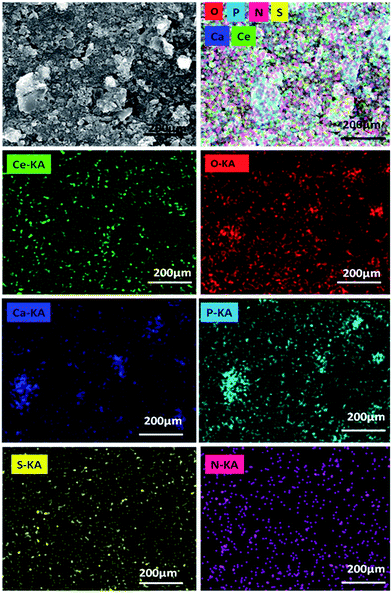 |
| Fig. 11 Elemental mapping of dye adsorbed CTAC functionalized CeO2 in the presence of HAp nanoparticles. | |
4.6. Adsorption kinetics
In order to examine the controlling mechanism of the adsorption processes, such as mass transfer and chemical reaction, pseudo first and second-order kinetics were used to test the experimental data. The pseudo first-order chemisorption kinetic rate equation is expressed as follows: |
 | (8) |
where Co and Ce are the initial and equilibrium concentrations of EBT in the solution and K1 is the rate constant (min−1). The values for rate constant K1 and regression coefficient R2 have been calculated from the plot of ln
Co/Ce vs. t (Fig. S11a†) and are tabulated in Table 1. The linear form of the pseudo second order kinetic model is expressed as follows: |
 | (9) |
where K2 (g mg−1 min−1) is the rate constant and qe is the amount of EBT adsorbed over the surface of the nanoparticles at equilibrium (Fig. S11b†). The experimental data indicate that the adsorption rate follows pseudo first-order kinetics for the removal of the dye, due to the higher fitting value of R2.
Table 1 Adsorption kinetics models of EBT dye on all nanoparticles
Nanoparticles |
Pseudo first order |
Pseudo second order |
K1 |
R2 |
K2 |
qe |
R2 |
HAp |
0.045 |
0.99 |
88.49 |
0.767 |
0.90 |
CeO2–Hap |
0.033 |
0.99 |
155.76 |
1.055 |
0.96 |
CTAB–CeO2–HAp |
0.189 |
0.96 |
89.85 |
1.119 |
0.98 |
CTAC–CeO2–HAp |
0.038 |
0.99 |
50.23 |
4.619 |
0.98 |
CPB–CeO2–HAp |
0.218 |
0.95 |
73.26 |
1.391 |
0.94 |
CPC–CeO2–HAp |
0.037 |
0.99 |
57.94 |
3.186 |
0.98 |
4.7. Applicability in real samples and salt effect
To check the applicability of the nanoparticles as an adsorbent in real samples, a variety of water samples was collected and the adsorptive behavior was tested by using them as solvents, instead of distilled water. Water samples from tap, well, tubewell, handpump and canal were used for this purpose. In each water sample, 50 ppm of dye was adsorbed using 50 ppm of nanoparticles and the percentage removal of dye was calculated (Fig. 12a). The results illustrated that EBT was successfully removed for all samples, but with slight reduction in removal efficiency. This could be attributed to interference caused by dissolved salts and low oxygen content in these water samples. This interference of salts was further tested from adsorption batches of dye containing 20 ppm of common salts present in water bodies, such as sodium chloride, nickel acetate, calcium carbonate, zinc sulphate and magnesium chloride (Fig. 12b). The results have shown that dissolved ions impart a small declining effect on the adsorption capacity of the nanoparticles. This decrease was associated with the competitive adsorption behavior of salts over the surface of nanoparticles. As a result, the dye molecules found less adsorption sites over the surface of the nanoparticles.
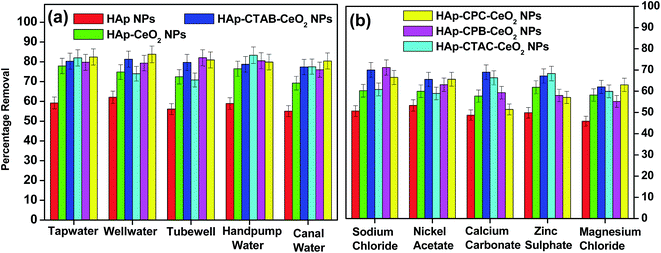 |
| Fig. 12 Effect on percentage dye removal by nanoparticles (a) in different real water samples and (b) in the presence of different salts. | |
5. Conclusions
CeO2 nanoparticles (both bare and surfactant stabilized) can penetrate cells and may interfere with cellular components, causing damage to cell division resulting in the inhibition of the growth of the organism. To summarize, we have shown the genotoxic and growth inhibitory effects of bare and surfactant stabilized CeO2 nanoparticles, which can be surpassed by using a biocompatible coating over the nanoparticles. The biocompatible coating leads to the enhancement of the size of the nanoparticles and alters the properties, such as making the nanoparticles non-toxic. The antiproliferative activity of around 0.00 to 7.7% PIG for HAp coated nanoparticles further enhanced the biocompatibility of as formed nanoparticles. The lower rate of chromosomal aberrations in the case of the HAp coating has clearly signified the biological importance of the as formed nanoparticles. The effect of coating on the surface properties of the nanoparticles was tested by adsorption experiments against EBT dye. The system was optimized with the help of different parameters, including the influence of pH, adsorbent dosage, initial dye concentration and time of contact. The findings show a slight decrease in dye adsorption efficiency by HAp coated nanoparticles, but a huge enhancement in the biocompatibility of the modified system. The prepared HAp/CeO2 nanohybrids can be exploited to act as competent low-cost adsorbents for removal and recovery of dye from the aqueous environment. This work validates and promotes the idea of using biocompatible and non-toxic nanomaterials for environmental remediation with high efficiency.
Acknowledgements
Savita Chaudhary is grateful to DST Inspire Faculty award [IFA-CH-17] and DST Purse grant II for financial assistance. Priyanka Sharma is grateful to DST India for providing a senior research Inspire fellowship [IF-140267].
References
- W. H. De Jong and P. J. A. Borm, Int. J. Nanomed., 2008, 3, 133–149 CrossRef CAS.
- S. Naahidi, M. Jafari, F. Edalat, K. Raymond, A. Khademhosseini and P. Chen, J. Controlled Release, 2013, 166, 182–194 CrossRef CAS PubMed.
- L. Yu, H. Wang, Y. Zhang, B. Zhang and J. Liu, Environ. Sci.: Nano, 2016, 3, 28–44 RSC.
- C. Walkey, S. Das, S. Seal, J. Erlichman, K. Heckman, L. Ghibelli, E. Traversa, J. F. McGinnis and W. T. Self, Environ. Sci.: Nano, 2015, 2, 33–53 RSC.
- M. Hajjari, M. Ardjmand and M. Tabatabaei, RSC Adv., 2014, 4, 14352–14356 RSC.
- C. M. Rico, S. C. Lee, R. Rubenecia, A. Mukherjee, J. Hong, J. R. Peralta-Videa and J. L. Gardea-Torresdey, J. Agric. Food Chem., 2014, 62, 9669–9675 CrossRef CAS PubMed.
- F. Pagliari, C. Mandoli, G. Forte, E. Magnani, S. Pagliari, G. Nardone, S. Licoccia, M. Minieri, P. D. Nardo and E. Traversa, ACS Nano, 2012, 6, 3767–3775 CrossRef CAS PubMed.
- A. Asati, S. Santra, C. Kaittanis and J. M. Perez, ACS Nano, 2010, 4, 5321–5331 CrossRef CAS PubMed.
- F. Piccino, F. Gottschalk, S. Seeger and B. Nowack, J. Nanopart. Res., 2012, 14, 1109 CrossRef.
- K. A. Amin, M. S. Hassan, S. T. Awadel and K. S. Hashem, Int. J. Nanomed., 2011, 6, 143–149 CrossRef CAS PubMed.
- A. Rocca, S. Moscato, F. Ronca, S. Nitti, V. Mattoli, M. Giorgi and G. Ciofani, Nanomed. Nanotech. Biol. Med., 2015, 11, 1725–1734 CrossRef CAS PubMed.
- V. Selvaraj, N. Nepal, S. Rogers, N. D. P. K. Manne, R. K. Arvapalli, K. M. Rice, S. Asano, E. Fankenhanel, J. Y. Ma, T. Shokuhfar, M. Maheshwari and E. R. Blough, Data in Brief, 2015, 4, 105–115 CrossRef PubMed.
- D. A. Pelletier, A. K. Suresh, G. A. Holton, C. K. McKeown, W. Wang, B. Gu, N. P. Mortensen, D. P. Allison, D. C. Joy and M. R. Allison, Appl. Environ. Microbiol., 2010, 76, 7981–7989 CrossRef CAS PubMed.
- P. Rosenkranz, M. F. Cruz, E. Conde, M. R. Fernandez, J. Flores, M. Fernandez and J. Navas, Toxicol. In Vitro, 2012, 26, 888–896 CrossRef CAS PubMed.
- Z. Zhang, X. He, H. Zhang, Y. Ma, P. Zhang, Y. Ding and Y. Zhao, Metallomics, 2011, 3, 816–822 RSC.
- R. Rajan, K. Chandran, S. L. Harper, S.-I. Yun and P. T. Kalaichelvan, Ind. Crops Prod., 2015, 70, 356–373 CrossRef CAS.
- K. Cheng, Y. Zhu, W. Weng, J. Lin and H. Wang, Thin Solid Films, 2015, 584, 9–12 CrossRef CAS.
- F. Tang, C. Wang, X. Wang and L. Li, Colloids
Surf., A, 2015, 485, 49–54 CrossRef CAS.
- E. S. Urquiza, F. A. Cardona, E. H. Herman, P. F. Pacheco-García, R. G. Rodríguez, J. L. Coffer, M. E. Mendoza-Alvarez, J. F. Vélez-Ruiz and M. A. Méndez-Rojas, J. Colloid Interface Sci., 2015, 460, 339–348 CrossRef PubMed.
- T. Ishihara, T. Maeda, H. Sakamoto, N. Takasaki, M. Shigyo, T. Ishida, H. Kiwada, Y. Mizushima and T. Mizushima, Biomacromolecules, 2010, 11, 2700–2706 CrossRef CAS PubMed.
- M. Muthiah, I. K. Park and C.-S. Cho, Biotechnol. Adv., 2013, 31, 1224–1236 CrossRef CAS PubMed.
- L. S. Acosta-Torres, L. M. López-Marín, R. E. Núñez-Anita, G. H. Padrón and V. M. Castaño, J. Nanomater., 2011, 8, 941561 Search PubMed.
- F. Hui and J. Lédion, Eur. J. Water Qual., 2002, 1, 1–27 Search PubMed.
- V. Sreeja, K. N. Jayaprabha and P. A. Joy, Appl. Nanosci., 2015, 5, 435–441 CrossRef CAS.
- A. Bhatnagar, E. Kumar and M. Sillanpää, Chem. Eng. J., 2010, 163, 317 CrossRef CAS.
- W. Li, D. Liu, J. Wu, C. Kim and J. D. Fortner, Environ. Sci. Technol., 2014, 48, 11892–11900 CrossRef CAS PubMed.
- M. Li, X. Xiao, R. Liu, C. Chen and L. Huang, J. Mater. Sci.: Mater. Med., 2008, 19, 797–803 CrossRef CAS PubMed.
- T. Moudakir, K. Djessas and G. Masse, J. Cryst. Growth, 2004, 270, 517–523 CrossRef CAS.
- R. Kumar, N. M. Sundaram and V. Rajendran, Int. J. Eng. Sci. Tech., 2010, 2, 2437–2444 Search PubMed.
- C. Piccirillo, C. W. Dunnill, R. C. Pullar, D. M. Tobaldi, J. A. Labrincha, I. P. Parkin, M. M. Pintado and P. M. L. Castro, J. Mater. Chem. A, 2013, 1, 6452–6461 RSC.
- M. Zhu, H. X. Wang, J. Y. Liu, H. L. He, X. G. Hua, Q. J. He, L. X. Zhang, X. J. Ye and J. L. A. Shi, Biomaterials, 2011, 32, 1986–1995 CrossRef CAS PubMed.
-
(a) S. Chaudhary, P. Sharma, R. Kumar and S. K. Mehta, Ceram. Int., 2015, 41, 10995–11003 CrossRef CAS;
(b) S. Chaudhary, A. Sood and S. K. Mehta, J. Nanosci. Nanotechnol., 2014, 14, 6824–6834 CrossRef CAS PubMed.
- M. Kumari, A. Mukherjee and N. Chandrasekaran, Sci. Total Environ., 2009, 407, 5243–5246 CrossRef CAS PubMed.
- N. I. M. Nayak and P. Lakshmi, J. Pharmacol. Pharmacother., 2014, 5, 56–58 CrossRef PubMed.
- S. Pakrashi, N. Jain, S. Dalai, J. Jayakumar and P. T. Chandrasekaran, PLoS One, 2014, 9, e87789 CrossRef PubMed.
- S. K. Mehta, S. Chaudhary, S. Kumar and S. Singh, J. Nanopart. Res., 2010, 5, 1697–1709 CrossRef.
- S. K. Mehta, S. Chaudhary and K. K. Bhasin, J. Nanopart. Res., 2009, 11, 1759–1766 CrossRef CAS.
- D. Jamwal, G. Kaur, P. Raizada, P. Singh, D. Pathak and P. Thakur, J. Phys. Chem. C, 2015, 119, 5062–5073 CrossRef CAS.
- C. Walkey, S. Das, S. Seal, J. Erlichman, K. Heckman, L. Ghibelli, E. Traversa, J. F. McGinnisf and W. T. Self, Environ. Sci.: Nano, 2015, 2, 33–53 RSC.
- A. P. Bijelić, M. Mojović, S. Stamenković, M. Jovanović, V. Selaković, P. Andjus and G. Bačić, Free Radicals Biol. Med., 2016, 96, 313–322 CrossRef PubMed.
- T. Pirmohamed, J. M. Dowding, S. Singh, B. Wasserman, E. Heckert, A. S. Karakoti, J. E. King, S. Seal and W. T. Self, Chem. Commun., 2010, 46, 2736–2738 RSC.
Footnote |
† Electronic supplementary information (ESI) available. See DOI: 10.1039/c6ra06933a |
|
This journal is © The Royal Society of Chemistry 2016 |
Click here to see how this site uses Cookies. View our privacy policy here.