DOI:
10.1039/C6RA06807C
(Paper)
RSC Adv., 2016,
6, 45968-45976
Rapid extraction of uranium ions from seawater using novel porous polymeric adsorbents†
Received
15th March 2016
, Accepted 3rd May 2016
First published on 4th May 2016
Abstract
Seawater contains uranium in surprisingly high quantities that can supply vast energy, if recovered economically. Attempts to design effective sorbents led to the identification of organic functional groups such as amidoximes. Here we report a porous polymer, a polymer of intrinsic microporosity (PIM) with permanent pores that feature amidoxime pendant groups, which is capable of removing more than 90% uranyl [U(VI)] from seawater collected from the Ulleung basin of the East Sea of the Republic of Korea. From this uptake, over 75% was collected in less than six hours, leading to highly feasible field applications. When the seawater was acidified by bubbling CO2 (pH = 5.4), the uptake increased dramatically. Regeneration studies showed full recovery of sorbents and no loss in capture capacity. Our results indicate that successful uranium recovery can be realized by scalable applications of porous polymeric networks and when low cost CO2 is co-administered, uptake can be significantly enhanced.
1. Introduction
Fossil fuel production has recently seen considerable increase, thanks to the new technologies in oil discovery and extraction. Considering the impact on the environment, in particular atmospheric heat transfers, burning carbon for energy continues to be a top environmental concern. Among the alternatives to fossil fuels, energy production from nuclear fission of radioactive elements remains the most mature and scalable. Uranium, the most popular nuclear fission fuel source, is commonly found in a wide variety of ores that are mined via conventional practices. The oceans, as an almost limitless resource, contain soluble species of many elements, including uranium. The runoff by the surface water continuously feeds the oceans with more, unless there is feedback by harvesting organisms. Over millions of years, uranium has accumulated, as its carbonate species, UO2(CO3)x, are highly water-soluble. The concentration of uranyl species is around 3 ppb and highly uniform throughout the oceans,1 resulting in estimates of four billion tonnes of uranium equivalent (∼800 times the proven land reserves).2 These estimates are not including the sedimentation of insoluble uranium minerals beneath the oceans.
Amidoximes,3,4 the oximes of amides, are known to be effective in reversible uranium uptake because of their selective coordination to uranyl ions.1,5 A range of amidoxime bearing linear polymer chains were prepared for this purpose,6,7 as well as a few cross-linked mesoporous examples.8 One of the first field demonstrations was by Tamada and co-workers in which the team installed amidoxime functionalities over inexpensive non-woven fibers by radiation induced graft polymerization of acrylonitriles.9 Rogers et al. later confirmed the coordination modes of uranium to amidoxime functionalities on ionic liquid model compounds.10 Bis-amidoximes (or imide oximes) are also found to improve uranyl binding.11,12 More recently, amidoxime-bearing polyethylene fibers are developed by an Oak Ridge National Laboratory (ORNL) team that showed up to 179 mg g−1 uptake at 6 ppm of uranium test samples, despite the slow adsorption kinetics.13 In a recent field application, ORNL sorbents led to an improved recovery of 3.3 mg U per g sorbent in 8 weeks of contact time and a $610 kg−1 U total cost.14
Remaining challenges using amidoxime-based sorbent for uranium capture would be to make the recovery of uranium from seawater more economically competitive. There are two characteristics, a desired uranium capture sorbent should bear: (1) high chemical specificity towards uranium from an oceanic soup of cations, (2) high porosity for effective diffusion and fast kinetic of treated water. In this regard, conventional porous materials such as activated carbons,15,16 graphene oxide,17 silica,18 and highly cross-linked polymers8 each provide a useful platform and continuously being exploited for the sorbent design. Proteins are also considered for uranyl specific peptide based capture19 as well as the dendrimers based on polyamidoamines (PAMAM) and polypropyleneimines (PPI).20 Among emerging new materials, porous network polymers offer both chemical functionality and enhanced diffusion because of their permanent intrinsic porosities.21 The main difference of porous polymers from other polymers is the structural certainty, in which porous networks are built in, not introduced by post modification procedures like cross-linking.22 Despite their favorable characteristics, no porous network polymer was investigated for uranium capture from seawater.
We have previously developed4 an amidoxime derivative of a polymer of intrinsic microporosity (PIM) structure, PIM-1 (ref. 23 and 24) and showed that amidoxime functionality was effective in carbon dioxide capture applications. The permanent porosity of a bent 1D chain played significant role in the mass transport of the flue gas systems.25 A third benefit of a PIM structure is their processability as they can be cast as membranes, while preserving their permanent porosities.24 The PIMs, despite their promise, were never utilized for uranium extraction from seawater. Herein, we present a rapid, recyclable uranium capture procedure from seawater by a nanoporous and processable polymer, amidoxime PIM-1. We show that having effective diffusivity through permanent pores provide rapid adsorption and desorption of uranium species in seawater. By carefully tuning the pH by bubbling CO2 in brine systems, we also conclude that the uptake capacities can be maximized.
2. Experimental details
Materials
Tetrafluoroterephthalonitrile (>98%) and 5,5′,6,6′-tetrahydroxy-3,3,3′,3′-tetramethyl-1,1′-spirobisindane (>96%), for PIM-1 synthesis, were purchased from TCI, Japan. Anhydrous potassium carbonate (99.5%) was obtained from SAMCHUN, South Korea. Hydroxyl amine (50 wt% solution in water, 99%), CDCl3 (99.8%) and DMSO-d6 (99.96%) were from Sigma-Aldrich, USA. Uranyl nitrate hexahydrate (98–102%, Fluka) was used to prepare U stock solution containing 1 × 10−3 M U(VI) in 0.1 M HNO3. Tris buffer solution was prepared by mixing of 0.1 M citric acid (>99.0%, Sigma-Aldrich) with 0.1 M trisodium citrate (ACS grade, Sigma-Aldrich) and of 0.05 M tris(hydroxymethyl) aminomethane (99.8%, Sigma-Aldrich) with 0.05 M trizma hydrochloride (99%, Sigma-Aldrich), respectively. All the samples for adsorption test were prepared using de-ionized water (DIW, 18.2 MΩ cm). Synthetic seawater was obtained by dissolving 40 g of sea salt (Sigma-Aldrich) in 1 L of DIW.
Synthesis of amidoxime PIM-1
Amidoxime PIM-1 was produced in large scale according to procedure reported in our previous study.4 3.9 g of PIM-1 (ref. 26) was dissolved in 280 mL anhydrous tetrahydrofuran via slight sonication (∼1 min), and heated up to 65 °C under Ar atmosphere. About 40 mL of NH2OH aqueous solution was equipped in syringe pump, and then added dropwise into the above solution with injection speed of 1 mL min−1. The mixture was refluxed at 69 °C for 20 hours with vigorous stirring. After the reaction, the solution was cooled down to room temperature, and precipitated in 800 mL of ethanol. The obtained precipitates were filtered and thoroughly washed with 300 mL of ethanol four times. The white powder was finally dried at 110 °C at least 3 hours to generate amidoxime PIM-1. Yield (based on PIM-1): 93%.
Characterization of amidoxime PIM-1
1H NMR spectrum of amidoxime PIM-1 was obtained using DMSO-d6 as solvent on a Bruker DMX400 NMR spectrometer. FT-IR spectra were recorded on KBr disks using a Perkin-Elmer FT-IR spectrometer. Field-Emission Scanning Electron Microscope (FE-SEM) images of amidoxime PIM-1 before and after uranium capture were obtained by FEI Nova 230. Elemental analysis (CHNO) was acquired by a sFlash 2000 series of Thermo Scientific. Thermogravimetric analysis (TGA) was conducted with DTG-60A of Shimadzu by heating samples up to 800 °C at a rate of 10 °C min−1 under N2 atmosphere. Porosity of amidoxime PIM-1 was analyzed with Micromeritics Triflex accelerated surface area and porosimetry analyzer at 77 K after all the samples were pre-degassed at 110 °C for 5 h in vacuo. The specific surface area of the samples was calculated by Brunauer–Emmett–Teller (BET) method, and the pore size distribution was determined by NLDFT (Non-Local Density Functional Theory) approach.
Uranium sorption studies
Solutions containing 1 μM (≒238 ppb) of U were prepared by adding appropriate amounts of 1 × 10−3 M U stock into Tris buffer (DIW, pH 8) and synthetic sea water, respectively. The pH values of synthetic seawater were adjusted to the desired pH using negligible amounts of 1 M hydrochloric acid and 1 M sodium hydroxide solution. A desired amount of amidoxime PIM-1 was transferred to 24 mL PTFE vials and the reaction was initiated by adding 20 mL of the solutions containing 1 μM uranium. All samples were prepared in duplicate and the vials were tightly capped and mechanically shaken at 180 rpm. The sample solutions were periodically (10, 30, 360, 720, 1440 min) filtered by 0.2 μm nylon syringe filter (Whatman). Each filtrate was diluted with 2% nitric acid and total U concentration in the diluted samples was measured by Inductively Coupled Plasma-Mass Spectrometry (Agilent 7700S, ICP-MS). The sorption efficiency (%) was calculated as follows:
where C0 and Ce are the initial and equilibrium concentration of U in aqueous phase, respectively. To investigate the effect of competing metal ions, amidoxime PIM-1, treated with synthetic seawater for 1 day, was fully dissolved using 35% nitric acids for 2 h at 70 °C. The metal ions leached out from the amidoxime PIM-1 sorbents were quantified using ICP-MS following analytical procedure described above. The reactivity of amidoxime PIM-1 was also examined in actual seawater condition. Seawater samples were collected from the Ulleung basin, East Sea of Korea. Particulate impurities of seawater were filtered using 0.8 μm filter (Whatman). The initial uranium concentration in actual seawater was found to be about 1.5 ppb, and the U concentration was controlled to 3.3 ppb in sorption test. The U-laden seawater was purged with CO2 gases for 1 h to adjust pH condition (5–6) for optimum U sorption. Batch sorption experiment using actual seawater was conducted under identical procedure described above.
Regeneration studies
The amidoxime PIM-1 after treatment was filtered using 0.2 μm nylon filter (Whatman), and the filtered sorbents were treated with 20 mL of 2 M sodium carbonate for 12 h to extract sorbed U.27 The sample was thoroughly washed with DIW 3 times and cycled to analyze regeneration/extraction efficiency of U ions.
3. Results and discussion
Characterization of amidoxime PIM-1
In order to obtain porous polymer that can sorb uranium via a binding of its amidoxime pendant groups, we first synthesized the polymer of intrinsic microporosity, PIM-1, a structurally entangled polymer with permanent pores from inefficient packing.24 Amidoxime PIM-1 was produced via post-modification of the nitrile groups in PIM-1 with hydroxylamine (Scheme 1), and the conversion of nitrile into amidoxime was confirmed by FTIR and 1H NMR spectroscopy (Fig. 1). The FTIR vibrations at 3480 and 3340 cm−1 are anti-symmetric and symmetric stretching mode of free NH2 group, respectively. A peak at 3175 cm−1 is due to O–H stretching vibration, and bands at 1656 and 915 cm−1 are assigned as C
N and N–O stretching vibrations of oxime functionality, respectively. The 1H NMR spectrum of amidoxime PIM-1 shows strong peaks at 5.81 and 9.44 ppm, indicating the presence of NH2 and OH groups in amidoxime moiety, respectively.
 |
| Scheme 1 Polymer of intrinsic microporosity, PIM-1 and its conversion to the amidoxime PIM-1, the uranium sorbent used in this study. | |
 |
| Fig. 1 (a) FTIR and (b) 1H NMR spectrum of amidoxime PIM-1. (c) N2 adsorption–desorption isotherm measured at 77 K. Inset displays the corresponding NLDFT pore size distribution. (d) SEM image of amidoxime PIM-1. | |
The porosity of amidoxime PIM-1 was confirmed by N2 physisorption measurement at 77 K. As shown in Fig. 1c, amidoxime PIM-1 is highly microporous and exhibits a type I sorption isotherm. The Brunauer–Emmett–Teller (BET) surface area of amidoxime PIM-1 was 531 m2 g−1 and total pore volume was 0.28 cm3 g−1. The NLDFT pore size distribution of amidoxime PIM-1 is narrow with average pore size of 2.5 nm (Fig. 1c inset). SEM image of amidoxime PIM-1 shows lumpy and porous morphology with micron sized grains. Elemental analysis of amidoxime PIM-1 (Table S1†) exhibits 4% increase in nitrogen content compared to the original PIM-1 structure, which well corresponds to its theoretical values. Thermogravimetric analysis of amidoxime PIM-1 (Fig. S1†) revealed high thermal stability up to 280 °C. However, around 300 °C, about 12% of mass loss was observed, which mainly results from the decomposition of amidoxime functional groups. FTIR spectra of amidoxime PIM-1 exhibit no change in functionality under wide pH windows (Fig. S2†), showing high chemical stability.
Amidoxime PIM-1 with high porosity and structural rigidity was subjected to uranium capture and recovery studies. We have investigated the effects of sorbent amount, contact time, pH, and counter ion for a rapid recovery of U from aqueous solutions. The results show that the U removal efficiency is exemplary even in a small dose amount of amidoxime PIM-1, and the rapid uptake (>75% in six hour) with full regeneration has great promise for field applications.
Uranyl uptake from deionized water
We monitored U sorption kinetics by different contents of amidoxime PIM-1 (0.01 g, 0.02 g, and 0.04 g) in 20 mL of aqueous U solution (pH 8), corresponding to solution volume to sorbent mass ratios (v
:
m) of 2000, 1000, and 500, respectively. As shown in Fig. 2a, U sorption rapidly occurred, even in the smallest dose of sorbent (i.e., highest ratio: 2000). Amidoxime PIM-1 removed 50% of aqueous U in 6 h. As the dose of sorbent increased by twice and four times, the sorption kinetics was accelerated. Greater than half of U was removed in aqueous solution in 30 min (first step) and then U was equilibrated at ∼83% in 6 h (second step). This shows that amidoxime PIM-1 could effectively uptake aqueous U at pH 8, average pH of seawater. The two kinetic steps with U uptake behavior by amidoxime PIM-1 can be explained using a pseudo-second order kinetic model as follows.
where qt [mg g−1] and qe [mg g−1] are the amounts of sorbed U(VI) at contact and equilibrium times, respectively; t is contact time [min]; k is rate constant [g mg−1 min−1]. As shown in Fig. 2b, the behavior of U sorption was nicely fitted by the kinetic model. The calculated rate constants were 3.5 × 10−2 (R2 = 0.991), 1.6 × 10−1 (R2 = 0.999), and 5.3 × 10−1 (R2 = 0.999) at the ratios of 2000, 1000, and 500, respectively. The rate constant increased by 15 times as the dose of amidoxime PIM-1 increased by 4 times. Pseudo-first order kinetic model was also applied to the data, showing significantly lower correlation coefficients (0.99735, 0.8588, and 0.4629 at the ratios of 2000, 1000, and 500, respectively). Consequently, the U sorption behavior in our study agrees well with the pseudo-second order kinetic model, which is suitable to describe the chemisorption process being the rate-controlling step. This good agreement implies that aqueous U uptake by amidoxime PIM-1 might be involved with the chemisorption by sharing or exchange of electrons of U with adsorbent.5,6,18,28 In addition, recent quantum calculation study have reported that η2 coordination with N–O bond between uranyl cation and amidoxime is the energetically most stable among the possible binding motifs. Uranyl cations are known as the dominant species in deionized water, therefore, it is more likely to suggest that U might be chemisorbed on the surface of amidoxime PIM-1 via η2 coordination with N–O bond.29
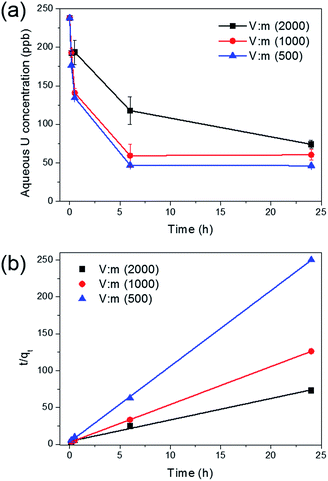 |
| Fig. 2 (a) Removal of aqueous U at different ratio between volume of solution (v) to mass of amidoxime PIM-1 sorbent (m), (b) and its sorption kinetics fitted with pseudo-second order kinetic model. | |
Uranyl uptake from synthetic seawater and pH effect
As the amidoxime PIM-1 showed high U uptake behavior in aqueous solution, we have investigated the sorption capacity of U by amidoxime PIM-1 in synthetic seawater at different pH conditions. Fig. 3a illustrates sorption kinetics of U (5 ppm) by amidoxime PIM-1 in synthetic seawater. At weak basic conditions (>pH 7), amidoxime PIM-1 was not able to remove aqueous UI, while a rapid sorption of U was observed at acidic conditions (pH 4 and 6). More enhanced sorption kinetics of aqueous U by the adsorbent was shown in the order of pH 6 > 4 > 7 = 8. Particularly, at pH 6, about 60% of U was removed in 1 h and reached equilibrium at 80% U removal in 6 h. At pH 4, on the other hand, sorption kinetic was much slower, i.e. aqueous U concentration was gradually decreased to 2 ppm in a week of interaction. The results show that pH 6 is an optimum condition for aqueous U uptake by amidoxime PIM-1 in synthetic seawater, which corresponds to the previous investigations.7,8,30 Zhang et al. have shown that weak acidic condition (pH 4–6) is the most favorable for U capture using amidoxime, and suggested that the change in amidoxime functional groups is responsible for different sorption kinetics of U at diverse pH conditions. Interestingly, however, as shown in Fig. S2,† FTIR spectra of amidoxime PIM-1 did not exhibit any change in functional groups under different pH conditions, thus, change in amidoxime functionality would not be the best explanation in this case. We, thus, assume that competing ions existing in synthetic seawater would affect U sorption behavior under the different pH conditions.
 |
| Fig. 3 (a) Effect of pH on U removal in synthetic seawater with dose of v : m (500). (b) Effect of competing metal ions attached on the amidoxime PIM-1 after being treated for 24 h at pH 6 and 8. | |
Fig. 3b exhibits metal ions adsorbed on the amidoxime PIM-1 during the U capture, where the metals were extracted from the sorbent, after being treated for synthetic seawater for 24 h, using 35% nitric acid solution as washing agent. Vanadium is known to be a major competing metal for U uptake from seawater resulting in decrease of U sorption capacity.31,32 In this study, dissolved V amounts from the adsorbent were similar as 1.827 and 1.865 ppb at pH 6 and 8, respectively. However, the amount of major metals, except V, leached out from the amidoxime PIM-1 surface was far different according to the pH conditions, indicating that U would be highly competing with metal species to bind on the surface of amidoxime PIM-1. The concentration of Ca2+, Zn2+, and Sr2+ on amidoxime PIM-1 was found to be 0.800, 0.840, and 0.076 ppm, respectively, at pH 8, while 0.127, 0.095, and 0.007 ppm, respectively, at pH 6. The content of metals at pH 8 was 6 to 10 times higher than that of at pH 6. This may be attributed to the change in surface charge of amidoxime PIM-1 depending on the pH of synthetic seawater. The cations such as Ca2+, Zn2+, and Sr2+ are known to be readily sorbed on the negatively charged surface.17,33 At higher pH, the fraction of negatively charged sites on amidoxime PIM-1 would be much more densely distributed than that of positively charged sites, therefore, electrostatic attractive force between the cations and the negatively charged sites could accelerate the competitive sorption process, finally resulting in less U uptake. Moreover, since protons can accelerate the dissociation of carbonate group from [UO2(CO3)3]4−, which is the rate-determining step for U uptake from seawater,34 low content of protons at neutral/basic condition may result in weak affinity of [UO2(CO3)3]4− toward surface functional groups of amidoxime PIM-1.
Fig. 4a shows sorption kinetics of U(VI) by amidoxime PIM-1 in synthetic seawater at pH 6. Rapid sorption of U was observed at the early stage, and 90% of U uptake was achieved in 12 h. With the dose of v
:
m (2000), 50% of U was removed in 30 min, and the concentration was equilibrated at >95% after 12 h. With the higher dose of amidoxime PIM-1 (v
:
m = 1000–250), more than 95% of U was adsorbed in 30 min, and the plateau was maintained for 24 h. The results revealed that amidoxime PIM-1 is a highly effective sorbent in terms of fast kinetic and high capacity for U uptake. The fast sorption kinetic was also analyzed by pseudo-second order kinetic model (Fig. 4b). The sorption data were well fitted by the kinetic model even under synthetic seawater conditions indicating chemisorption process of U on the amidoxime PIM-1 surface. The rate constants were calculated as 5.5 × 10−2, 5.6 × 10−1, 7.9 × 10−1, and 32 × 10−1 g mg−1 min−1 for 2000, 1000, 500, and 250 of v
:
m conditions, respectively. The corresponding correlation coefficients (R2) were 0.998, 0.999, 0.999, and 0.999. With the dose more than 1000 of v
:
m, U was almost completely removed in 30 min. The results showed that even the small quantity of amidoxime PIM-1 dose can rapidly uptake U in synthetic seawater.
 |
| Fig. 4 (a) Aqueous U removal by amidoxime PIM-1 with different volume to mass ratio sorbent at pH 5 in synthetic seawater. (b) Sorption kinetics of amidoxime PIM-1 interpreted by pseudo-second order kinetic model. | |
Table 1 summarizes the U uptake kinetic constant (k) and distribution coefficient (Kd [g L−1] = Uadsorbent [mg g−1]/Uequilibrium [mg L−1]) of amidoxime PIM-1 and its comparison with recent studies. Obtained k and Kd were 7.9 × 10−1 and 8.2 in this study. The sorption kinetics (k) was 15 to 1500 times faster compared to the reported data and the Kd was 1.4 to 27 times higher than the other adsorbents, except nonwoven fabric. Moreover, among these materials only amidoxime PIM-1 have been tested in synthetic seawater environment, proving that the developed adsorbent in this study can rapidly and effectively uptake U from actual seawater.
Table 1 Comparison of the reported amidoxime based adsorbents for uranium uptake
Functional group |
Adsorbent type |
Cinitial |
Uranium solution |
pH |
k [g mg−1 min−1] |
Kd [L g−1] |
Ref. |
v : m = 500 (40 mg adsorbent/20 mL solution). |
Amidoxime |
Porous polymer |
238 ppb |
Synthetic seawater |
6 |
7.9 × 10−1 |
8.2 |
This studya |
Amidoxime |
Nonwoven fabric |
50 ppb |
Deionized water |
7.5 |
1.2 × 10−3 |
39.8 |
Liu et al. (2012)41 |
Amidoxime |
Polyvinyl alcohol fiber |
976 ppm |
Deionized water |
4 |
9.2 × 10−4 |
8.2 |
Chi et al. (2013)42 |
Amidoxime |
Na-montmorillonite |
47.6 ppm |
NaNO3 |
4 |
2.1 × 10−2 |
6.0 |
Li et al. (2015)43 |
Amidoxime |
Nonwoven fabric |
7.1 ppm |
NaCl, NaHCO3 |
8 |
5.7 × 10−4 |
11.4 |
Zeng et al. (2015)44 |
Amidoxime |
Wool fibers |
100 ppm |
Deionized water |
5 |
2.4 × 10−3 |
0.3 |
Yin et al. (2016)45 |
Amidoxime |
Fe3O4·SiO2 |
23.8 ppm |
NaClO4 |
5 |
1.7 × 10−3 |
0.6 |
Zhao et al. (2014)46 |
Amidoxime |
CNT |
100 ppm |
Deionized water |
4.5 |
1.5 × 10−2 |
0.9 |
Wang et al. (2014)47 |
Amidoxime |
Fe3O4·graphene |
47.6 ppm |
NaClO4 |
5 |
5.0 × 10−4 |
2.4 |
Zhao et al. (2013)30 |
Carboxylate |
TiO2–cellulose |
25 ppm |
Deionized water |
6 |
4.7 × 10−2 |
0.3 |
Anirudhan et al. (2010)48 |
Vinylphosphonic acid |
Polyvinyl alcohol fiber |
98.6 ppm |
Deionized water |
4 |
1.1 × 10−2 |
3.3 |
Chi et al. (2013)49 |
Uranyl uptake from actual seawater and regeneration of sorbents
The high uranyl capture of amidoxime groups at pH of 6 prompted us to offer ways to achieve the best recovery. Among acidification options, carbon dioxide bubbling serves the optimal sustainability for two reasons: (1) CO2 dissolves in water to make pH 5–6 at equilibrium with 300 ppm atmospheric concentration or more,35 and (2) power generation produces vast amounts of CO2 that has no immediate use, making it essentially a very low cost chemical feedstock.36 In a typical operation, a constant stream of CO2 feed would be passed through a fluidized bed system of oceanic water (or brines from desalination plants) to maintain a pH of around 5–6. Excess CO2 bubbles away and spent sorbents are collected for regeneration to be prepared for the next cycle.
The U sorption under actual seawater condition and concomitant sorbent regeneration were conducted with the dose of v
:
m (500) to minimize experimental error caused from unavoidable mass loss of the sorbents during regeneration test. The pH of actual seawater was titrated by passing CO2 gas feed for 1 h, and pH of the seawater was successfully maintained to be 5.4 throughout the reaction (Fig. 5a). Fig. 5b represents U uptake by amidoxime PIM-1 at pH 6 in Ulleung basin seawater controlled to have 3.3 ppb of U. Aqueous U in the seawater titrated by CO2 purging was rapidly and completely removed in 12 h by amidoxime PIM-1 with removal capacity of 96.4%. The obtained kinetic constant and Kd were 11 × 10−1 (g mg−1 min−1) and 16.7 (L g−1), respectively (Fig. 5c). The uptake kinetics were better in chemical acidification with 1 M HCl (Fig. 5b), indicating the CO2 purging method for pH control also makes slightly more stable uranyl carbonate species during the uptake from seawater. But the total removal capacities become identical after 24 hours, indicating that the equilibrium for speciation shifted for the capture once more uranyls are captured. The uptake efficiency of amidoxime PIM-1 is comparable with other U adsorbents in terms of a rapid kinetic and sorption efficiency with respect to dose amount. Manos et al. reported that layered metal sulfide (v
:
m of 100) captured 76.3–84.2% U in actual seawater condition (3.8 ppb of initial U concentration).27 The amidoxime PIM-1, in this study, showed more than 95% uptake in 6 h with one-fifth amount of sorbent needed for the uptake of U by the layer metal sulfide.
 |
| Fig. 5 (a) pH monitoring of actual seawater titrated by purging CO2 gases (b) U sorption behavior of amidoxime PIM-1 in actual seawater (Ulleung basin, Republic of Korea). The pH of seawater was adjusted to be about 5.5 using either by chemical treatment of 1 M HCl or gaseous CO2 bubbling. (c) Sorption kinetics of amidoxime PIM-1 interpreted by pseudo-second order kinetic model, and (d) corresponding sorbent regeneration and U extraction test. | |
Regeneration of amidoxime PIM-1 would enhance its economic feasibility for field application.37 Fig. 5d displays three cycles of U adsorption and extraction percentage under actual seawater condition. After the adsorption of uranium, the amidoxime PIM-1 sorbent was treated with 2 M Na2CO3 for the sorbent regeneration and U recovery. The U removal was found to be intact with high removal from the first to third cycles (95.4, 93.1, and 91.9%). The U extraction also shows that more than 92% of U can be recovered throughout three cycles. The results demonstrate that amidoxime PIM-1 can be simply regenerated and reused for U uptake from actual seawater.
Towards a membrane application
Most of U uptake studies reported so far are based on batch system, which may not be feasible for industrial application since large stream of U-laden aqueous medium (such as those found in desalination brines) would prefer continuous system. Unlike common porous polymers, amidoxime PIM-1 is soluble in DMF, DMSO, DMAc,4,38 and it can be easily fabricated on membrane by the solution casting method, proving its good processibility for large-scale applications. Moreover, sequential U sorption tests showed that amidoxime PIM-1 can efficiently uptake U from actual seawater with mild acidic condition (pH 6), thus, amidoxime PIM-1 membrane would be suitable for ex situ U capture process. The membrane of amidoxime PIM-1 (Fig. 6a) shows high flexibility, and SEM image of the film exhibits that amidoxime PIM-1 is uniformly packed throughout the surface with the small lumpy polymer granules (Fig. 6b). Future study would go along with a practical optimization of amidoxime PIM-1 membrane for actual field application, i.e. membrane operation parametric study (feed concentration, trans-membrane pressure etc.), mechanical strength test, and alkaline treatment.39,40
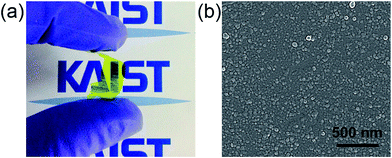 |
| Fig. 6 (a) A photograph of flexible film of amidoxime PIM-1. Amidoxime PIM-1 was dissolved in DMF and cast on Petri dish, then kept under Ar atmosphere for 1 day. The dish was further heated in the oven at 150 °C for 3 h to give amidoxime PIM-1 thin film. (b) SEM image of the corresponding amidoxime PIM-1 film. | |
4. Conclusion
Uranium capture from seawater can unlock the unlimited energy resource that is not dependent of fossil fuels. Though it has its own environmental impact, nuclear energy can be far more controllable, if monitored correctly. Sorbents that can effectively capture uranium from seawater have to have chemical affinity and suitable porosity for fast turnovers. Porous polymers that have permanent intrinsic pores are capable of providing both, an opportunity that is left unexploited. We have shown that a nanoporous polymer, PIM-1 with amidoxime pendant groups can recover uranium from actual seawater in high capacities (>90%) and fast (>50% within the hour). Regenerated sorbent can be used multiple times without deterioration. This study marks one of the first attempts to bring designed porous network polymers for the effective capture of uranium in seawater, and certainly will pave the way for breakthrough discoveries of tapping into a vast energy source.
Acknowledgements
This work was mainly supported by the IWT funding (NRF-2012-C1AAA001-M1A2A2026588). C. T. Y. also acknowledges Korea CCS R&D Centre, Basic Science Research Program through the National Research Foundation of Korea (NRF), ICT & Future Planning (2013R1A1A1012998), and KAIST EEWS Initiative. J. B. thanks to Global Ph.D. fellowship (2013H1A2A1033423).
References
- R. V. Davies, J. Kennedy, R. W. McIlroy, R. Spence and K. M. Hill, Nature, 1964, 203, 1110–1115 CrossRef.
- I. Tabushi, Y. Kobuke and T. Nishiya, Nature, 1979, 280, 665–666 CrossRef CAS.
- S. Zulfiqar, F. Karadas, J. Park, E. Deniz, G. D. Stucky, Y. Jung, M. Atilhan and C. T. Yavuz, Energy Environ. Sci., 2011, 4, 4528–4531 CAS.
- H. A. Patel and C. T. Yavuz, Chem. Commun., 2012, 48, 9989–9991 RSC.
- J. Kim, C. Tsouris, R. T. Mayes, Y. Oyola, T. Saito, C. J. Janke, S. Dai, E. Schneider and D. Sachde, Sep. Sci. Technol., 2013, 48, 367–387 CrossRef CAS.
- S. Das, A. K. Pandey, A. A. Athawale and V. K. Manchanda, J. Phys. Chem. B, 2009, 113, 6328–6335 CrossRef CAS PubMed.
- A. Y. Zhang, T. Asakura and G. Uchiyama, React. Funct. Polym., 2003, 57, 67–76 CrossRef CAS.
- Y. Yue, R. T. Mayes, J. Kim, P. F. Fulvio, X.-G. Sun, C. Tsouris, J. Chen, S. Brown and S. Dai, Angew. Chem., Int. Ed., 2013, 52, 13458–13462 CrossRef CAS PubMed.
- N. Seko, A. Katakai, S. Hasegawa, M. Tamada, N. Kasai, H. Takeda, T. Sugo and K. Saito, Nucl. Technol., 2003, 144, 274–278 CAS.
- P. S. Barber, S. P. Kelley and R. D. Rogers, RSC Adv., 2012, 2, 8526–8530 RSC.
- G. Tian, S. J. Teat, Z. Zhang and L. Rao, Dalton Trans., 2012, 41, 11579–11586 RSC.
- G. Tian, S. J. Teat and L. Rao, Dalton Trans., 2013, 42, 5690–5696 RSC.
- T. Saito, S. Brown, S. Chatterjee, J. Kim, C. Tsouris, R. T. Mayes, L. J. Kuo, G. Gill, Y. Oyola, C. J. Janke and S. Dai, J. Mater. Chem. A, 2014, 2, 14674–14681 CAS.
- J. Kim, C. Tsouris, Y. Oyola, C. J. Janke, R. T. Mayes, S. Dai, G. Gill, L. J. Kuo, J. Wood, K. Y. Choe, E. Schneider and H. Lindner, Ind. Eng. Chem. Res., 2014, 53, 6076–6083 CrossRef CAS.
- G. Tian, J. X. Geng, Y. D. Jin, C. L. Wang, S. Q. Li, Z. Chen, H. Wang, Y. S. Zhao and S. J. Li, J. Hazard. Mater., 2011, 190, 442–450 CrossRef CAS PubMed.
- Y. F. Yue, X. G. Sun, R. T. Mayes, J. Kim, P. F. Fulvio, Z. A. Qiao, S. Brown, C. Tsouris, Y. Oyola and S. Dai, Sci. China: Chem., 2013, 56, 1510–1515 CrossRef CAS.
- H. Chen, D. D. Shao, J. X. Li and X. K. Wang, Chem. Eng. J., 2014, 254, 623–634 CrossRef CAS.
- Y. G. Zhao, J. X. Li, S. W. Zhang and X. K. Wang, RSC Adv., 2014, 4, 32710–32717 RSC.
- L. Zhou, M. Bosscher, C. S. Zhang, S. Ozcubukcu, L. Zhang, W. Zhang, C. J. Li, J. Z. Liu, M. P. Jensen, L. H. Lai and C. He, Nat. Chem., 2014, 6, 236–241 CrossRef CAS PubMed.
- M. S. Diallo, W. Arasho, J. H. Johnson and W. A. Goddard Iii, Environ. Sci. Technol., 2008, 42, 1572–1579 CrossRef CAS PubMed.
- N. B. McKeown and P. M. Budd, Chem. Soc. Rev., 2006, 35, 675–683 RSC.
- H. A. Patel, F. Karadas, J. Byun, J. Park, E. Deniz, A. Canlier, Y. Jung, M. Atilhan and C. T. Yavuz, Adv. Funct. Mater., 2013, 23, 2270–2276 CrossRef CAS.
- P. M. Budd, B. S. Ghanem, S. Makhseed, N. B. McKeown, K. J. Msayib and C. E. Tattershall, Chem. Commun., 2004, 230–231, 10.1039/b311764b.
- M. Carta, R. Malpass-Evans, M. Croad, Y. Rogan, J. C. Jansen, P. Bernardo, F. Bazzarelli and N. B. McKeown, Science, 2013, 339, 303–307 CrossRef CAS PubMed.
- R. Swaidan, B. S. Ghanem, E. Litwiller and I. Pinnau, J. Membr. Sci., 2014, 457, 95–102 CrossRef CAS.
- P. M. Budd, E. S. Elabas, B. S. Ghanem, S. Makhseed, N. B. McKeown, K. J. Msayib, C. E. Tattershall and D. Wang, Adv. Mater., 2004, 16, 456–459 CrossRef CAS.
- M. J. Manos and M. G. Kanatzidis, J. Am. Chem. Soc., 2012, 134, 16441–16446 CrossRef CAS PubMed.
- Y. S. Ho and G. McKay, Process Biochem., 1999, 34, 451–465 CrossRef CAS.
- S. Vukovic, L. A. Watson, S. O. Kang, R. Custelcean and B. P. Hay, Inorg. Chem., 2012, 51, 3855–3859 CrossRef CAS PubMed.
- Y. G. Zhao, J. X. Li, S. W. Zhang, H. Chen and D. D. Shao, RSC Adv., 2013, 3, 18952–18959 RSC.
- P. A. Kavakli, N. Seko, M. Tamada and O. Guven, Sep. Sci. Technol., 2004, 39, 1631–1643 CrossRef.
- T. Suzuki, K. Saito, T. Sugo, H. Ogura and K. Oguma, Anal. Sci., 2000, 16, 429–432 CrossRef CAS.
- T. S. Anirudhan and M. Ramachandran, Ind. Eng. Chem. Res., 2008, 47, 6175–6184 CrossRef CAS.
- C. Z. Wang, J. H. Lan, Q. Y. Wu, Q. Luo, Y. L. Zhao, X. K. Wang, Z. F. Chai and W. Q. Shi, Inorg. Chem., 2014, 53, 9466–9476 CrossRef CAS PubMed.
- E. O. Mclean, in Methods of Soil Analysis. Part 2. Chemical and Microbiological Properties, ed. A. L. Page, American Society of Agronomy, Soil Science Society of America, Madison, WI, 1982, pp. 199–224 Search PubMed.
- Z.-Z. Yang, L.-N. He, J. Gao, A.-H. Liu and B. Yu, Energy Environ. Sci., 2012, 5, 6602–6639 CAS.
- H.-B. Pan, W. Liao, C. M. Wai, Y. Oyola, C. J. Janke, G. Tian and L. Rao, Dalton Trans., 2014, 43, 10713–10718 RSC.
- N. Y. Du, G. P. Robertson, J. S. Song, I. Pinnau and M. D. Guiver, Macromolecules, 2009, 42, 6038–6043 CrossRef CAS.
- H. B. Pan, L. J. Kuo, J. Wood, J. Strivens, G. A. Gill, C. J. Janke and C. M. Wai, RSC Adv., 2015, 5, 100715–100721 RSC.
- C. T. S. Das, C. Zhang, J. Kim, S. Brown, Y. Oyola, C. J. Janke, R. T. Mayes, L. J. Kuo, J. R. Wood, G. A. Gill and S. Dai, Ind. Eng. Chem. Res., 2016, 55, 4294–4302 CrossRef.
- X. Y. Liu, H. Z. Liu, H. J. Ma, C. Q. Cao, M. Yu, Z. Q. Wang, B. Deng, M. Wang and J. Y. Li, Ind. Eng. Chem. Res., 2012, 51, 15089–15095 CrossRef CAS.
- F. T. Chi, S. Hu, J. Xiong and X. L. Wang, Sci. China: Chem., 2013, 56, 1495–1503 CrossRef CAS.
- W. P. Li, X. Y. Han, X. Y. Wang, Y. Q. Wang, W. X. Wang, H. Xu, T. S. Tan, W. S. Wu and H. X. Zhang, Chem. Eng. J., 2015, 279, 735–746 CrossRef CAS.
- Z. H. Zeng, Y. Q. Wei, L. Shen and D. B. Hua, Ind. Eng. Chem. Res., 2015, 54, 8699–8705 CrossRef CAS.
- Z. L. Yin, J. Xiong, M. Chen, S. Hu and H. M. Cheng, J. Radioanal. Nucl. Chem., 2016, 307, 1471–1479 CrossRef CAS.
- Y. G. Zhao, J. X. Li, L. P. Zhao, S. W. Zhang, Y. S. Huang, X. L. Wu and X. K. Wang, Chem. Eng. J., 2014, 235, 275–283 CrossRef CAS.
- Y. Wang, Z. X. Gu, J. J. Yang, J. L. Liao, Y. Y. Yang, N. Liu and J. Tang, Appl. Surf. Sci., 2014, 320, 10–20 CrossRef CAS.
- T. S. Anirudhan and S. S. Sreekumari, Colloids Surf., A, 2010, 361, 180–186 CrossRef CAS.
- F. T. Chi, X. L. Wang, J. Xiong and S. Hu, J. Radioanal. Nucl. Chem., 2013, 296, 1331–1340 CrossRef CAS.
Footnotes |
† Electronic supplementary information (ESI) available. See DOI: 10.1039/c6ra06807c |
‡ Equal contribution. |
|
This journal is © The Royal Society of Chemistry 2016 |