DOI:
10.1039/C6RA06713A
(Paper)
RSC Adv., 2016,
6, 48189-48198
Synthesis of aminopropyltriethoxysilane grafted/tripolyphosphate intercalated ZnAl LDHs and their performance in the flame retardancy and smoke suppression of polyurethane elastomer†
Received
14th March 2016
, Accepted 9th May 2016
First published on 11th May 2016
Abstract
A novel flame retardant containing both phosphorus and silicon elements, composed of aminopropyltriethoxysilane grafted/tripolyphosphate intercalated ZnAl LDHs (A-LDHs), was synthesized by anion exchange and IHS (induced hydrolysis silylation) method, and characterized by XRD, FTIR, XPS, and TG. After being added into a PUE matrix, A-LDHs could exfoliate and disperse randomly in the matrix while the composites containing pristine LDHs (N-LDHs) still preserved the stacking lamellar structure. Moreover, T5, T10, T50 and Tmax of the composites containing A-LDHs were higher than those of PUE/LDHs composites containing N-LDHs, resulting from the good dispersion of A-LDHs. And A-LDHs performed better in the flame retardancy and smoke suppression of PUE than N-LDHs. Furthermore, the mechanism was investigated through the analysis of the condensed phase after cone calorimetry tests via LRS, FTIR and XPS. The findings showed that the improved flame retardancy and smoke suppression might originate from a more stable char layer due to the introduction of P and Si elements.
1. Introduction
Recently, more and more environmental legislation has restricted or banned the application of halogen-containing flame retardants in polymers since they give rise to toxic and dense smoke. Layered double hydroxides (LDHs) have received considerable attention as a rather new halogen free flame retardant. LDHs have a layered structure that can be represented by the chemical formula of [MII1−xMIIIx(OH)2]x+[(An−)x/n·mH2O], where M2+ is a divalent cation, M3+ is a trivalent cation, and An− denotes an inorganic or organic anion with negative charge n. Since both the composition of the metal layers and the gallery anions can be varied, there is enormous potential for preparing LDHs with a wide range of applications including use as flame retardants.1
S. Liu investigated the flame retardancy of MgAl LDHs in epoxy resin (ER), and the result showed that at a 1 wt% LDHs loading, the LOI value of ER was increased from 15.9 to 21.7, indicating good flame retardancy.2 C. Nyambo investigated the flame retardancy of MgAl LDHs in poly(methyl methacrylate) (PMMA) and polystyrene (PS), the best peak heat release rates (PHRR) reduction in PS was 56% observed at 10% LDHs loading, they noted that the flame retardant mechanism of LDHs involves both changes in the mass loss rate and some cooling effect due to the release of water.3 D. Y. Wang studied the flammability of fire retarding ethylene-propylene-diene terpolymer (EPDM)/layered double hydroxide (LDHs) nanocomposites, they thought that the flame retardant mechanism was mainly due to the reinforced char layers during combustion, leading to the low volatiles produced.4
It is well known that phosphorus-containing compounds as efficient halogen-free flame retardants are often used alone or combined with magnesium hydroxide or aluminium hydroxide, which are able to increase the conversion of organic matter to charred layers during burning. The charring of polymers can effectively reduce the generation of heat and hazardous materials during their combustion, such as flammable gases, toxic gases and smoke particles.5,6 L. P. Gao investigated the synergistic effect between phosphorus-containing flame retardant and organically-modified LDHs (OLDHs) on the thermal stability and fire behavior of polyisocyanurate–polyurethane foam (PIR foam). Their results showed that OLDHs had a synergistic effect with phosphorus-containing flame retardants on improving the flame retardancy of PIR foam. The reason is that OLDHs can promote the formation of a diethyl ethylphosphonate reinforced char layer, provide an effective barrier against heat and oxygen, release noncombustible gases and simultaneously effectively suppress smoke and gases during the combustion process.7 L. Ye found that LDHs intercalated by phosphate possessed enhanced thermal stability and flame retardant properties compared with ordinary carbonate-intercalated LDHs in the ethylene vinyl acetate (EVA) blends. The flame retarding mechanism of phosphate-intercalated LDHs can be ascribed to its catalysis degradation of the EVA resin, which promotes the formation of charred layers with the P–O–P and P–O–C complexes in the condensed phase.8 Y. G. Lee revealed that phosphorus could block active sites by forming either C–O–P or C–P–O bonds at graphene edges to inhibit carbon oxidation.9
However, the presence of hydroxyl groups makes LDHs hydrophilic, leading to a poor compatibility between LDHs and polymer matrix. And modification with suitable organic molecules renders LDHs highly appropriate for making polymer composites. Intercalation of anionic surfactants like sulphonates or carboxylates can make the LDHs interlayer hydrophobic as non-polar hydrocarbon chains fill the interlayer space.10 Silylation, also known as silane grafting, has been proven effective in enhancing the adhesion of polymers to LDHs.11 Q. Tao investigated the grafting of four silanes, trimethylchlorosilane (TMCS), dimethyldiethoxylsilane (DMDES), aminopropyltriethoxysilane (APTS) and tetraethoxysilane (TEOS), to LDHs via an induced hydrolysis silylation method (IHS). Their results indicated that APTS and TEOS could be grafted onto LDHs surfaces via condensation with hydroxyl groups of LDHs, while TMCS and DMDES could only be adsorbed on the LDHs surface with a small quantity. As far as APTS were concerned, –NH2 could be a key factor for the successful grafting of APTS.12 Y. Yuan found that [3-(methyl-acryloxy)propyl]trimethoxysilane modified LDHs showed fine dispersion and random orientation in acrylate resin, and their mechanical and thermal properties, as well as hardness were enhanced to different extents compared to pure polymer.13 Similarly, P. F. Lu found that vinyltriethoxysilane grafted LDHs could also be dispersed disorderly in unsaturated polyester with exfoliated structures.14
To our best knowledge, there have been no reports of the APTS grafted/tripolyphosphate intercalated ZnAl LDHs (A-LDHs) at present. Therefore, sodium tripolyphosphate (STPP) was used to prepare tripolyphosphate intercalated LDHs (S-LDHs) via anion exchange reaction followed by the grafting between the hydroxyl groups of S-LDHs with the ethoxy groups of APTS to synthesize A-LDHs in this study. Furthermore, A-LDHs was added into the polyurethane elastomer (PUE) to investigate the morphology, thermal stability and fire properties of PUE/LDHs composites. Particularly, the flame-retardant mechanism of A-LDHs was comprehensively studied using laser Raman spectroscopy (LRS), Fourier transform infrared (FTIR) and X-ray photoelectron spectroscopy (XPS).
2. Experimental
2.1. Materials
Al(NO3)3·9H2O, Zn(NO3)3·6H2O and C2H5OH were purchased from Sinopharm Chemical Reagent Co., Ltd. (China). Sodium tripolyphosphate (STPP), aminopropyltriethoxysilane (APTS) were purchased from Aladdin Chemical Reagent Co., Ltd. (China). NaOH was purchased from Xilong Chemical Co., Ltd., (China). Toluene diisocyanate (TDI) was purchased from Mitsui Chemical Co., Ltd., (Japan). Polyester polyol was purchased from Shandong Dexin Chemical Co., Ltd., (China). 3,3′-Dichloro-4,4′-diaminodiphenylmethane (MOCA) was purchased from Jinan Haiwu Chemical Co., Ltd., China.
2.2. LDHs synthesis
Pristine LDHs (NO3–MgAl LDHs) was prepared by a conventional hydrothermal method. Typically, a suspension of Al(NO3)3·9H2O and Zn(NO3)2·6H2O with a total metal ion concentration of 0.3 M (Zn2+/Al3+ = 2
:
1), an aqueous solution of 1 M NaOH was added dropwise to the mother suspension with vigorous stirring under nitrogen until a pH of 6.5 was reached. Then the mixture solution with constant appointed pH was transferred to a 100 mL stainless-steel Teflon-lined autoclave with a fill factor of 80%. The autoclave was heated to 160 °C for 24 h. Finally, the autoclave was cooled down to room temperature naturally and the precipitate was centrifuged, washed with distilled water and dried at 60 °C, and the product was referred as N-LDHs.
P3O105− intercalated LDHs was prepared by anion exchange. Fresh slurry (200 mL, containing 1 g of solid) of N-LDHs was stirred for 30 min at room temperature. 1.2 g STPP was dissolved in 100 mL deionized water, and then added to the above slurry. The synthesis was carried out at room temperature and flushed with nitrogen for 4 h. Finally, the suspension was centrifuged, washed with ethanol and dried at 60 °C. The product was named S-LDHs.
The APTS grafted/P3O105− intercalated LDHs was prepared by an induced hydrolysis silylation method (IHS). 1 g S-LDHs was immersed in a solution of APTS in ethanol (3 g in 100 mL of ethanol) under stirring at 60 °C for 6 h, and then washed with ethanol in ultrasonic bath to remove unreacted APTS. Finally, the suspension was centrifuged, washed with ethanol and dried at 60 °C. The product was called A-LDHs.
2.3. Preparation of PUE/LDHs composites
Polyester polyol was heated to 110 °C, stirred and vacuumed for 2 h to remove trace water. After that, the vacuuming was stopped and the temperature was cooled down to 75 °C. Then TDI was added and reacted for 2 h to prepare prepolymer. With that, the prepolymer, MOCA and LDHs were mixed and stirred fully and the mixture was casted on a Teflon mold at 80 °C for 8 h and 120 °C for 4 h. Finally, PUE/LDHs composites were prepared. Table 1 shows the formulation of PUE/LDHs composites.
Table 1 Formulation of PUE/LDHs composites
Samples |
Prepolymer (g) |
MOCA (g) |
LDHs (g) |
LDHs contents (wt%) |
N-LDHs |
A-LDHs |
PUE0 |
45.31 |
4.69 |
0 |
0 |
0 |
PUE1 |
44.86 |
4.64 |
0.5 |
0 |
1 |
PUE2 |
43.96 |
4.54 |
1.5 |
0 |
3 |
PUE3 |
43.05 |
4.45 |
2.5 |
0 |
5 |
PUE4 |
42.14 |
4.36 |
3.5 |
0 |
7 |
PUE5 |
40.78 |
4.22 |
5 |
0 |
10 |
PUE6 |
44.86 |
4.64 |
0 |
0.5 |
1 |
PUE7 |
43.96 |
4.54 |
0 |
1.5 |
3 |
PUE8 |
43.05 |
4.45 |
0 |
2.5 |
5 |
PUE9 |
42.14 |
4.36 |
0 |
3.5 |
7 |
PUE10 |
40.78 |
4.22 |
0 |
5 |
10 |
2.4. Characterization techniques
X-ray diffraction (XRD) patterns were performed with a Bruker D8-Advance diffractometer (BRUKER, Germany), using Ni-filtered Cu Kα radiation (λ = 0.1542 nm). Fourier transform infrared (FTIR) spectra were recorded with a Nicolet 6700 FTIR spectrophotometer (Thermo Fisher Scientific, U.S.) by using a standard KBr pellet technique. X-ray Photoelectron Spectroscopy (XPS) was performed with an Escalab 250 spectrometer (Thermo Scientific Ltd., America), using Al Kα excitation radiation (hν = 1486.6 eV). Thermal gravimetric (TG) analysis was performed with a STA 409PC thermal analyzer (NETZSCH, Germany) with a constant heating rate of 10 °C min−1. The scanning electron microscopy (SEM) image of LDHs was taken using a JEOL JSM-7500F instrument (JEOL Co., Japan). The specimens were sputter-coated with a gold/palladium alloy ready for imaging. Transmission electron microscope (TEM) micrographs were obtained using a JEM-2100 instrument (JEOL Co., Japan) operating at an accelerating voltage of 200 kV. The samples were dispersed in deionized water and then dripped onto copper grids before observation. Cone calorimetry tests were performed on a JCZ-2 cone calorimeter (Jiangning, China) under an external heat flux of 50 kW m−2 irradiance with the sheet dimensions of 100 × 100 × 3 mm3 according to ISO5660-1 standard procedures. Smoke density tests were performed on a JSC-2 smoke density test machine (Jiangning, China) according to ISO 5659-2 standard procedures. Each specimen with dimensions of 75 × 75 × 2.5 mm3 was wrapped in aluminum foil and exposed horizontally to an external heat flux of 25 kW m−2 irradiance with the application of a pilot flame. Laser Raman spectroscopy (LRS) of residue was performed with a SPEX-1403 laser Raman spectrometer (SPEX Co., USA) at room temperature.
3. Results and discussion
3.1. Characterization of LDHs
3.1.1. XRD characterization. The XRD patterns of N-LDHs, S-LDHs and A-LDHs are shown in Fig. 1. It is generally known that the interlayer distance of 8.9 Å for nitrate ion intercalated LDHs is larger than that of carbonate-containing LDHs (7.6 Å), the N-LDHs pattern is a typical nitrate ion intercalated LDHs. The reflection peaks marked by ‘*’ may belong to the byproduct of ZnO. In the S-LDHs pattern, it's obvious that the (003) and (006) reflection peaks shift to the lower diffraction angle, and the expanded basal spacing indicates that the tripolyphosphate anions are indeed intercalated into the interlayer of LDHs by the anion exchange reaction. The merge of (110) and (113) reflection peaks is possibly due to a disturbance of the brucite-like layer structure, which can also be indicated by the decreasing intensity of (003) and (006) reflection peaks. Through grafting modification, the unchanged interlayer distance of A-LDHs indicates that the graft of APTS occurs mainly on the surface of LDHs.
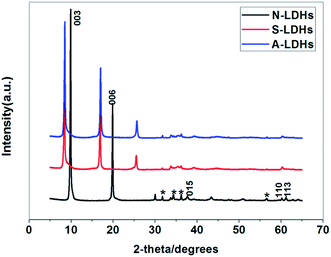 |
| Fig. 1 XRD patterns of LDHs. | |
3.1.2. FTIR characterization. The FTIR spectra of N-LDHs, S-LDHs and A-LDHs are shown in Fig. 2. In the N-LDHs spectrum, an intense broad band centered at 3450 cm−1 is assigned to O–H stretching vibration of water molecular and hydroxyl groups of brucite-like layers, and another adsorption band corresponding to water deformation is recorded around 1623 cm−1, and the intense adsorption band around 1389 cm−1 is ascribed to the symmetric stretching mode of the nitrate ions. In the spectrum of S-LDHs, the intensity of the band at 1389 cm−1 decreases significantly, indicating that the vast majority of NO3− is replaced by P3O105−.
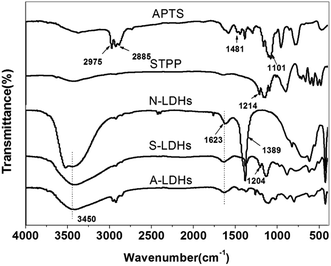 |
| Fig. 2 FTIR spectra of LDHs. | |
Compared with STPP, the band at 1214 cm−1 in the spectrum of STPP corresponds to the P
O stretching mode shifts to lower wave numbers (1204 cm−1) in S-LDHs, which indicates a decrease in the electron density of P
O bond due to the interactions with LDHs taking place through the lone electron pairs of the oxygen atoms in P3O105−. Therefore, it can be concluded that P3O105− interacts strongly with the hydroxyl groups of the LDHs through hydrogen bonding.15 Furthermore, the band characteristic of hydroxyl groups undergoes some broadening and becomes non-symmetrical indicating the existence of hydrogen bonding between the P3O105− and hydroxyl groups/water molecules.16 The above analysis shows that P3O105− has been successfully intercalated into the LDHs, and in addition to the electrostatic force, hydrogen bonds are also formed between the P3O105− and lamellar hydroxyl groups/interlayer water molecules. Compared with S-LDHs, the bands that appear at 2885–2975 cm−1 in A-LDHs are attributed to the C–H stretching band of aminopropyl groups of APTS, and the broadened band around 1137 cm−1 is attributed to the overlap of the Si–O band (1101 cm−1) and the P3O105− band (1137 cm−1).17 From these data, it can be concluded that APTS has been grafted onto the surface of A-LDHs.
3.1.3. XPS characterization. Fig. 3 is the XPS spectra of N-LDHs, S-LDHs and A-LDHs. In the survey spectra, the binding energy for Al 2p at 75.0 eV is associated to an aluminum hydroxide species Al(OH)n.18 The binding energy of Zn 3d, Zn 3s, Zn 2p3/2, Zn 2p1/2 asymmetric Auger lines (ZnL3M45M45) suggest the presence of Zn element in the LDHs. The Auger signals positioned at a higher energy peak (500 eV) is assigned to the bonding of Zn–OH.19 The N 1s binding energy (406 eV) in the XPS spectra of N-LDHs belongs to NO3−, and the disappearance of NO3− in S-LDHs implies the successful exchange of nitrate with P3O105−, which could be further confirmed by the appearance of P 2p and P 2s located at 133.5 eV and 190.3 eV, respectively.20 In the spectra of A-LDHs, the binding energy for Si 2p at 103.6 eV implies the successful graft of ATPS onto the surface of A-LDHs. In the Si 2p spectrum, the peaks at 102.9 eV, 103.7 eV and 104.5 eV are the binding energy of Si–C, Si–O–C and Si–O–M (M = Zn/Al).21,22 We can conclude that the chemical bond (Si–O–M) is formed by the reaction between Si–OH and the hydroxyl groups of LDHs layers, and ATPS is grafted on the LDHs surface through covalent bond.
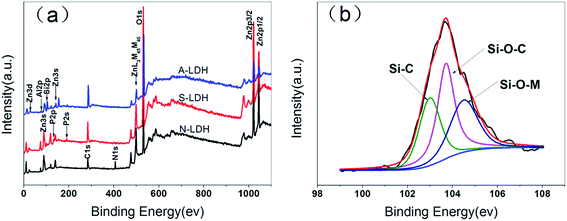 |
| Fig. 3 XPS spectra (a) LDHs and (b) Si 2p. | |
3.1.4. TG characterization. The TG curves of all LDHs are shown in ESI Fig. S1.† Generally, the hydrotalcite structure losses its water from the room temperature to around 200 °C, including the evaporation of adsorbed water (around 100 °C) and the elimination of the interlayer crystalline water (around 200 °C), the simultaneous dehydroxylation and deionization of the hydrotalcite framework occurs in 200–400 °C. When temperatures are further raised, LDHs are completely decomposed to form layered double oxides (LDO) (mixed metallic oxides).23 However, the non-volatile nature of the interlayer polyphosphate makes the thermal behavior more complicated. Obviously, the weight loss below 200 °C for LDHs is mainly assigned to water in LDHs. The weight loss for N-LDHs observed above 200 °C is ascribed to the dehydroxylation of metal hydroxide layer and deionization of NO3−. As for S-LDHs and A-LDHs, the TG curves are different from that of N-LDHs due to the volatilizable anion NO3− is replaced by the non-volatilizable anion P3O105−. Furthermore, the weight loss of A-LDHs is higher compared to that of S-LDHs, indicating the effective modification of S-LDHs by APTS.
3.1.5. SEM and TEM characterization. Fig. 4 shows the SEM and TEM images of LDHs. As can be seen, N-LDHs are an aggregate of typical lamellar structure attached with some small particles, as mentioned in the XRD analysis, and byproduct ZnO may appear in the synthesis process. Through ion exchange reaction, S-LDHs maintain the lamellar structure. S-LDHs and A-LDHs TEM images show a certain level of curves as marked parts by the rectangle in the images. This might be because that the LDHs lamella tends to thin during the intercalating and grafting reaction process and they get curled when touched by high energy electrical beams.24 According to A. V. Radha research, the anion exchange reactions of LDHs take place by the dissolution-reprecipitation mechanism rather than by the topotactic mechanism.25 In the SEM image of A-LDHs, the extent of lamella aggregation increases significantly. This phenomenon suggests that APTS is grafted on the surface of LDHs and exerts certain impacts on the aggregation morphology.
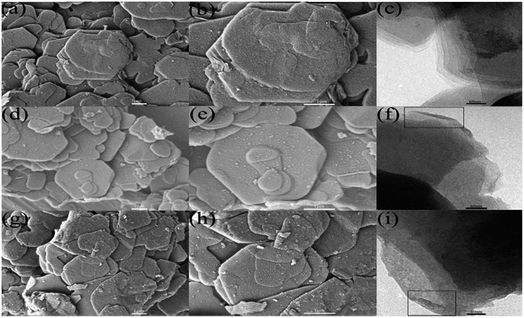 |
| Fig. 4 SEM and TEM of N-LDHs (a–c), S-LDHs (d–f) and A-LDHs (g–i). | |
3.2. Characterization of PUE/LDHs composites
3.2.1. Morphology of PUE/LDHs composites. XRD is an effective technique for observing the intercalation or exfoliation of LDHs structures. Fig. 5 shows the XRD patterns of the original LDHs and of the PUE composites with different LDHs loadings. As can be seen, all PUE composites show peaks located at 2θ = 20° that correspond to the crystallization of the soft segment of PUE.26 Obviously, PUE1 and PUE3 preserve the characteristic reflections of N-LDHs, diffraction peaks are observed at 2θ = 9.9° (003), 19.8° (006), and the intensity of diffraction peaks are increased with the increasing LDHs loadings, indicating that the N-LDHs are not intercalated by PUE macromolecular chains, and that no obvious intercalation or exfoliation occurs in the composites. However, the diffraction peaks located at 2θ = 8.4° (003) and 17.0° (006) attributed to A-LDHs characteristic reflections are absent in the PUE6 and PUE8 composites, indicating a collapse of the lamellar structure of stacked LDHs and thus a formation of disordered or exfoliated structure within the matrix. When the A-LDHs loadings increase to 5 wt%, a very weak peak is registered at around 2θ = 11.0° for PUE8 composites, which may be attributed to the γ-phase crystals of polymer.27 Probably, the –NH2 groups in the APTS strengthen the interaction between hydrotalcite-like compound layers and polymer chains, which favors the formation of the γ crystalline phase.28 In conclusion, the A-LDHs have been exfoliated and dispersed randomly in the matrix.
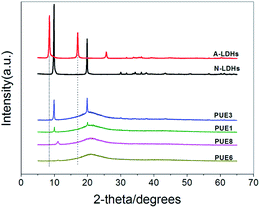 |
| Fig. 5 XRD patterns of LDHs and PUE/LDHs composites. | |
Although the XRD is indicative of a good dispersion of A-LDHs in PUE composites, more direct information can be obtained from SEM, as shown in Fig. 6. The A-LDHs (Fig. 6(b)) are dispersed as exfoliated thin layers while the N-LDHs in PUE1 are badly agglomerated in Fig. 6(a). As the loading rises up to 5 wt%, the cross section of PUE3 becomes rough. However, the PUE8 composite presents a relative smooth cross section, indicating good compatibility between the A-LDHs and PUE matrix. The results show that STPP and ATPS could improve the homogeneous dispersion of the LDHs in the PUE composites due to their better hydrophobicity.
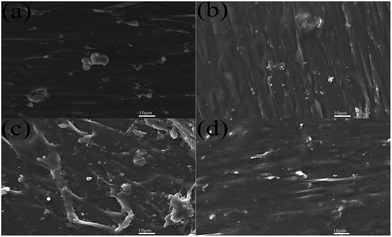 |
| Fig. 6 SEM of the cross section of (a) PUE1, (b) PUE6, (c) PUE3 and (d) PUE8. | |
3.2.2. Thermal stability of PUE/LDHs composites. Based on the situation which approached to realistic thermal decomposition, we investigated the thermal behavior of PUE and PUE/LDHs composites under air conditions, as shown in Fig. 7. The corresponding analysis data are listed in ESI Table S1.† Obviously, the initial decomposition temperature of the PUE/LDHs composites is lower than that of the neat PUE. This is because the LDHs as a weak alkaline can catalyze the degradation of amide groups in PUE, combining the weight loss of LDHs at low temperature.29 However, it's observed that the T5, T10, T50 and Tmax of PUE/LDHs composites containing A-LDHs are higher than those of PUE/LDHs composites containing N-LDHs. This is because the exfoliated A-LDHs layers dispersed disorderly in the PUE matrix produce good obstructive effect for the escape of degraded low molecules and thus remedy the negative catalytic degradation effect of LDHs on the PUE matrix.30 However, when the temperature is higher than 450 °C, the PUE/LDHs composites exhibit enhanced thermal stability due to the promoted char formation.31 This indicates that LDHs could promote the final char formation while catalyzing the initial decomposition of PUE/LDHs composites. Furthermore, A-LDHs added PUE/LDHs composites display more char than N-LDHs added PUE/LDHs composites when the LDHs loading is up to 10 wt%, which may result from the more stable char layer due to the existence of P, Si.
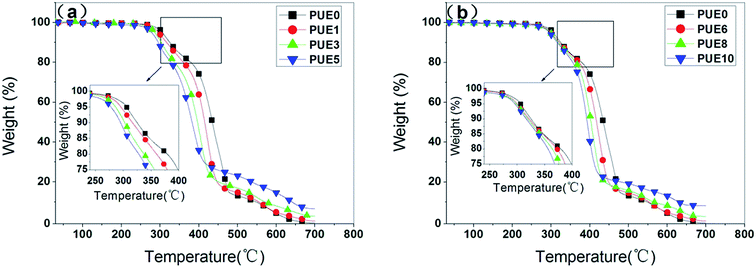 |
| Fig. 7 TG curves of PUE/LDHs composites. | |
3.2.3. Flame retardancy of PUE/LDHs composites. The flame retardancy of PUE/LDHs composites was investigated by cone calorimetry test. From Fig. 8, we could get the following information:
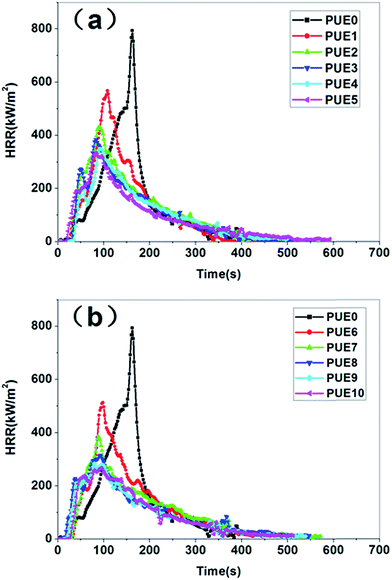 |
| Fig. 8 HRR curves of PUE/LDHs composites. | |
(1) The additions of LDHs shorten the time to the peak heat release rate (PHRR) for two main reasons. Primarily, the LDHs could catalyze the degradation of amide group of PUE in advance as a weak alkaline.29 Secondly, the addition of LDHs to PUE is expected to increase the viscosity of the neat polymer reducing heat exchange between the sample surface exposed to the radiant source and the bulk of the sample, which would result in a rapid increase in the surface temperature and accelerate the pyrolysis significantly.32
(2) However, the PHRR of PUE composites decreases significantly. Compared with PUE0 (793 kW m−2), when 1 wt% is added into PUE, the PHRR of PUE1 and PUE6 are 567 and 521 kW m−2, decreased by 28.5% and 35.4%, respectively. It can be observed that the PHRR decreases with increasing LDHs loadings. When up to 5 wt% LDHs loadings, the PHRR of PUE3 and PUE8 drop to 384 and 316 kW m−2, decreased by 51.6% and 60.2%, respectively. Nevertheless, the PHRR decreases slightly with more LDHs loadings. The remarkably reduced PHRR for the PUE/LDHs composites could be explained in two aspects. On the one hand, LDHs undergoes decomposition through an endothermic reaction, which acts as a heat sink leading to a reduced temperature of the combustion system. At the same time, the released vapour derived from the LDHs decomposition could dilute the oxygen concentration around the material and hinder the full combustion of the material.33 On the other hand, the char layer covering the material surface formed by the catalyzing charring effect of LDHs slows down the heat and mass transfer between gas and condensed phases and prevents the underlying material from further combustion. And the mixed metal oxide formed by decomposition of LDHs compacts and stabilizes the char layer to exert a better barrier effect.34
(3) Compared with N-LDHs, A-LDHs could reduce the PHRR of PUE/LDHs composites more effectively. In addition to the above reasons, the flame retardant elements P and Si migrate to the char surface and play the role of char enhancer, which would further improve the stability of the char layer.35
The flame retardancy of LDHs for PUE/LDHs could also be evaluated by the total heat release (THR), as shown in ESI Fig. S2.† Compared with PUE0, 62.7 MJ m−2, the THR of both PUE1 and PUE6 are reduced to 60 MJ m−2. To further increase the LDHs loadings, A-LDHs shows better flame retardancy. When the LDHs loading is up to 7 wt%, the THR of PUE4 is 51.7 MJ m−2, decreased by 17.5% while the same A-LDHs loading causes the THR of PUE9 to fall to 48.5 MJ m−2, a decrease by 22.6%.
3.2.4. Smoke suppression of PUE/LDHs composites. The smoke density curves and data for neat PUE and PUE/LDHs composites are shown in Fig. 9 and ESI Table S2.† For PUE0, the maximum smoke density (Ds,max) and smoke density at 10 min (Ds,10 min) are 421 and 391, respectively. With the addition of LDHs, both the Ds,max and Ds,10 min of PUE/LDHs composites are decreased. When 5 wt% N-LDHs is added, the Ds,max and Ds,10 min of PUE3 are 315 and 274, decreased by 25.2% and 29.9%, respectively. However, the same loading of A-LDHs reduces the Ds,max and Ds,10 min of PUE8 to 204 and 178, decreased by 51.5% and 54.5%, respectively. This is mainly due to the barrier effect not only plays a role in flame retardancy as discussed in the cone calorimetry test, but also has the function of smoke suppression. The compacted char layer could inhibit the release of smoke during the combustion process, and it also explains the better smoke suppression effect of A-LDHs from the barrier aspect. It's observed that 7 wt% N-LDHs is required for PUE4 to reduce the Ds,max and Ds,10 min to 220 and 196, while only 3 wt% A-LDHs could achieve a similar smoke suppression effect for PUE7. This indicates that A-LDHs exerts a better smoke suppression effect as P, Si enhance the char layer.
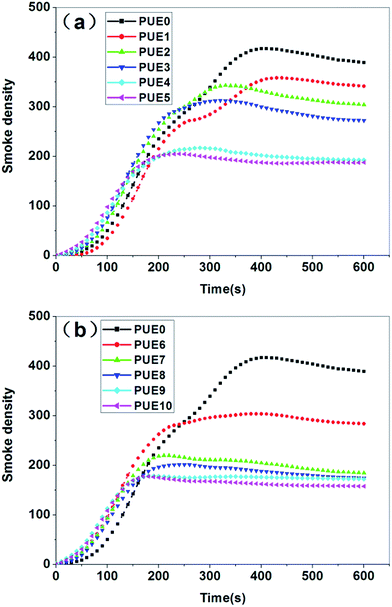 |
| Fig. 9 Smoke density curves of PUE/LDHs composites. | |
3.3. Char residue analysis
3.3.1. LRS analysis. Laser Raman spectra (LRS) are usually used to characterize the graphitic structure of materials, and to evaluate the order degree of carbon materials. Fig. 10 presents the LRS of the char residue for PUE0, PUE3 and PUE8 after cone calorimetry test. The curve-fitting software Origin is used for every spectrum to divide the curves into two Gaussian bands, and the two visible bands provide positive evidence for the formation of polyaromatic species or so-called graphitic structures.36 The band around 1590 cm−1 (G band) corresponds to an E2g mode of hexagonal graphite and is related to the vibration of sp2-bonded carbon atoms in a graphite layer, whereas the peak at approximate 1370 cm−1 (D band) is attributed to the vibrations of carbon atoms with dangling bonds in the plane terminations of disordered graphite or glassy carbons. The graphitization degree of the char can be estimated from the ratio of the intensities of the G and D bands (IG/ID). The higher IG/ID value means a higher degree of graphitization of char residue, which corresponds to relatively better flame-retardant performance.37 It should be noted that the IG/ID ratio follows the sequence PUE0 (0.223) < PUE3 (0.259) < PUE8 (0.267), indicating that LDHs could transfer amorphous char into graphitic structure during the material combustion, which enhances the strength of the char layer and compacts them to perform a better barrier effect. Furthermore, the IG/ID ratio for PUE8 is higher than that of PUE3, implying that the flame-retardant performance of A-LDHs is more efficient than N-LDHs, and the result is consistent with the cone calorimetry test experiment.
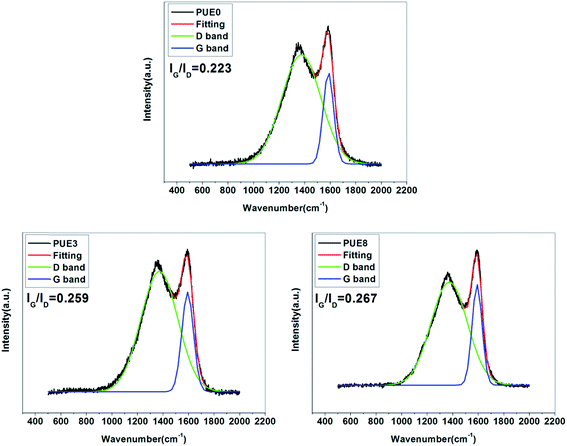 |
| Fig. 10 Laser Raman spectra of the char residue for PUE0, PUE3 and PUE8. | |
3.3.2. FTIR analysis. In order to further understand the reasons for the better flame-retardant performance of A-LDHs, the chemical components of the char layer of PUE3 and PUE8 after cone calorimetry test are measured by FTIR, as shown in Fig. 11. It's obvious that both char layers show an intensive peak at 656 cm−1 attributed to M–O or O–M–O (M = Zn, Al), and three extra absorption peaks are shown in the char layer of PUE8. The absorption peak at 1052 cm−1 means that Si–O structures remain in the char. The peak at 1000 cm−1 is attributed to the appearance of compounds such as polyphosphate or SiO2. In the meantime, an absorbance peak at 948 cm−1 which represents Si–O–phenyl or P–O–phenyl stretching vibrations is detected. We speculate that P and Si may participate in the incarbonization process and these interactions could enhance the thermal stability of the products in the condensed phase during the combustion.38 The superior thermal stability of the char could explain the better flame-retardant performance of A-LDHs.
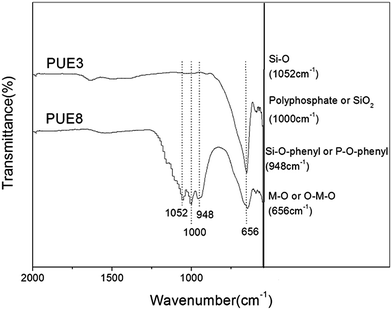 |
| Fig. 11 FTIR of the char residue for PUE3 and PUE8. | |
3.3.3. XPS analysis. The char from the PUE0, PUE8 char layer surface (PUE8-surface) and inner (PUE8-inner) is further investigated by XPS, whose results are shown in Fig. 12. The C 1s spectra feature three peaks at 284.7 eV, 286.4 eV and 288.9 eV that are attributed to the C–H and C–C in aliphatic and aromatic species, C–O in ether and/or hydroxyl group and C
O, respectively.39 The thermal oxidative resistance can be investigated through calculating Cox/Ca, where Cox and Ca represent oxidized carbons (C–O, C
O) and aliphatic and aromatic carbons (C–C, C–H), respectively. The lower the Cox/Ca value, the better the thermal oxidative resistance of the char layers formed during the combustion process of the material.40 This is mainly because the lower Cox/Ca value indicates more content of the polyaromatic species in the char layer. More polyaromatic species mean a denser morphology, a better barrier effect and higher flame retardance. The polyaromatic cross-linked networks are the foundations that make materials extinguish in the fire.41 The C 1s related data are listed in Table 2, the Cox/Ca value of the PUE0 is the highest showing the lowest thermal oxidative resistance. The Cox/Ca value of the PUE8-inner is the lowest, only 0.14, which can be explained by the compacted char layer formed in the surface that prevents the internal material from contacting the ambient oxygen, reducing the oxidation degree of the internal material greatly. And the relatively high Cox/Ca value for PUE8-surface is due mainly to oxygen atoms that are combined with P and Si atoms to form a stable char layer.42 In the O 1s spectrum, peaks at 533 eV, 531.7 eV, 530.9 eV and 529.8 eV are attributed to the C–O–C, Si–O–Si, M–O–M and O
P groups.33 It is observed that the char layer of PUE0 contain many C–O–C groups, and O
P group is mainly appears in the PUE8-surface layer, which might be because in the combustion process, most of the P element migrate to the surface to facilitate the formation of a stable char layer. In the P 2p spectrum of PUE8-surface, the P 2p spectrum can be separated into two peaks at 133.4 eV and 134.6 eV attributed to –P(
O)–O–C– and –P(
O)–O–Si–, respectively.43 This suggests that the migrated P elements in the surface not only form –P(
O)–O–C– structure, but –P(
O)–O–Si– as well, a more stable linkages structure.
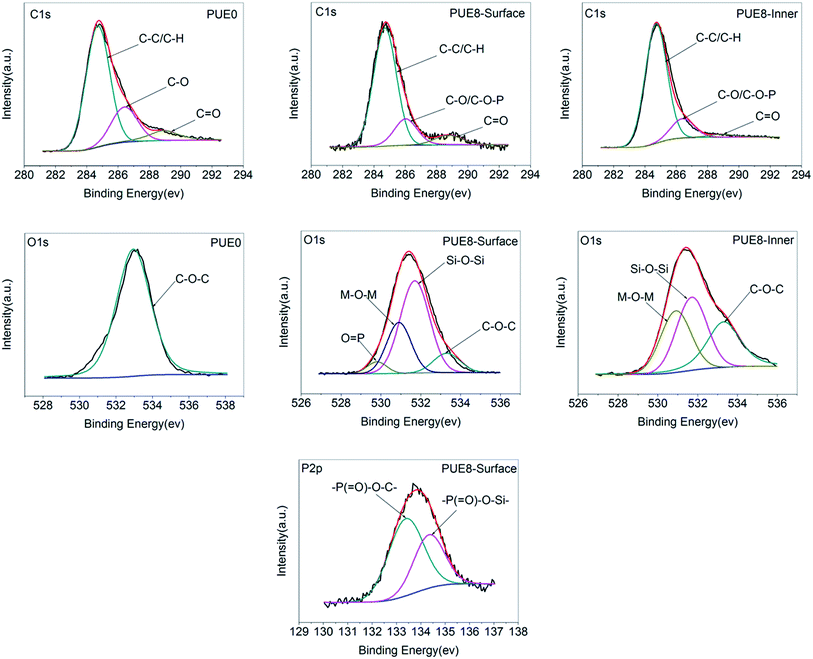 |
| Fig. 12 XPS spectra of the char residue. | |
Table 2 Results of C 1s XPS of char residue of PUE/LDHs composites
Element |
PUE0 |
PUE8-inner |
PUE8-surface |
C–C (284.7 eV) |
0.69 |
0.88 |
0.74 |
C–O (286.4 eV) |
0.22 |
0.10 |
0.17 |
C O (288.9 eV) |
0.09 |
0.02 |
0.09 |
Cox/Ca |
0.44 |
0.14 |
0.35 |
4. Conclusions
In this work, the APTS grafted/tripolyphosphate intercalated ZnAl LDHs (A-LDHs) has been synthesized through the anion exchange and IHS method. We have revealed that in A-LDHs, the P3O105− exists in the interlayer of LDHs through electrostatic force and hydrogen bonding, and APTS is grafted on the LDHs surface through covalent bond (Si–O–M) formed by the condensation reaction between Si–OH and the hydroxyl groups of LDHs layers. The modified LDHs is added into the PUE matrix, the XRD and SEM analysis show that A-LDHs could exfoliate and disperse randomly in the matrix in comparison with the unmodified LDHs. Compared with N-LDHs, A-LDHs added composites exert better performance in thermal stability, flame retardancy and smoke suppression. Both the T5, T10, T50 and Tmax of PUE/LDHs composites containing A-LDHs are higher than those of PUE/LDHs composites containing N-LDHs. When 1 wt% LDHs is added into PUE, the PHRR of PUE1 (N-LDHs) and PUE6 (A-LDHs) are decreased by 28.5% and 35.4%, respectively, compared with that of neat PUE. The Ds,max of PUE3 containing 5 wt% N-LDHs decreases to 315 while PUE8 containing the same loading of A-LDHs decreases the Ds,max to 204. Furthermore, the LRS, FTIR and XPS confirm that composites containing A-LDHs produce a more stable char layer due to the introduction of P and Si elements, which may stabilize the char layer by the formation of stable structures to promote the quality of the char layer, and consequently provide a shielding effect from the heat source, oxygen, and so on, and finally the good thermal stability, flame retardancy and smoke suppression.
Acknowledgements
The authors thank the Research Fund for the Doctoral Program of Anhui Jianzhu University (2014) and National Key Technology R&D Program (2013BAJ01B05) for their financial support.
Notes and references
- Y. Q. Shu, P. G. Yin, J. F. Wang, B. L. Liang, H. Wang and L. Guo, Ind. Eng. Chem. Res., 2014, 53, 3820–3826 CrossRef CAS.
- S. Liu, H. Q. Yan, Z. P. Fang, Z. H. Guo and H. Wang, RSC Adv., 2014, 4, 18652–18659 RSC.
- C. Nyambo, P. Songtipya, E. Manias, M. M. J. Gasco and C. A. Wilkie, J. Mater. Chem., 2008, 18, 4827–4838 RSC.
- D. Y. Wang, A. Das, A. Leuteritz, R. N. Mahaling, D. Jehnichen, U. Wagenknecht and G. Heinrich, RSC Adv., 2012, 2, 3927–3933 RSC.
- M. Z. Dong, X. Y. Gu and S. Zhang, Ind. Eng. Chem. Res., 2014, 53, 8062–8068 CrossRef CAS.
- X. Y. Shan, L. Song, W. Y. Xing, Y. Hu and S. M. Lo, Ind. Eng. Chem. Res., 2012, 51, 13037–13045 CrossRef CAS.
- L. P. Gao, G. Y. Zheng, Y. H. Zhou, L. H. Hu, G. D. Feng and M. Zhang, Polym. Degrad. Stab., 2014, 101, 92–101 CrossRef CAS.
- L. Ye and B. J. Qu, Polym. Degrad. Stab., 2008, 93, 918–924 CrossRef CAS.
- Y. J. Lee and L. R. Radovic, Carbon, 2003, 41, 1987–1997 CrossRef CAS.
- H. Y. Zeng, P. H. Zhu, S. Xu, M. C. Liao, Z. Q. Zhang, X. J. Liu and J. Z. Du, Ind. Eng. Chem. Res., 2014, 53, 18380–18389 CrossRef CAS.
- Q. Tao, J. Yuan, R. L. Frost, H. P. He, P. Yuan and J. X. Zhu, Appl. Clay Sci., 2009, 45, 262–269 CrossRef CAS.
- Q. Tao, H. P. He, T. Li, R. L. Frost, D. Zhang and Z. S. He, J. Solid State Chem., 2014, 213, 176–181 CrossRef CAS.
- Y. Yuan and W. F. Shi, Mater. Res. Bull., 2011, 46, 124–129 CrossRef CAS.
- P. F. Lu, Polym.-Plast. Technol. Eng., 2010, 49, 1450–1457 CrossRef CAS.
- M. A. B. Muñoz, L. V. F. Gonzalez, M. E. P. Bernal, R. J. R. Casero and V. S. Escribano, J. Inclusion Phenom., 1984, 1, 411–417 CrossRef.
- M. Badreddine, M. Khaldi, A. Legrouri, A. Barroug, M. Chaouch and A. D. Roy, Mater. Chem. Phys., 1998, 52, 235–239 CrossRef CAS.
- J. L. Hu, M. Y. Gan, L. Ma, Z. T. Li, J. Yan and J. Zhang, Surf. Coat. Technol., 2014, 240, 55–62 CrossRef CAS.
- K. A. D. F. Castro, A. Bail, P. B. Groszewicz, G. S. Machado, W. H. Schreiner, F. Wypych and S. Nakagaki, Appl. Catal., A, 2010, 386, 51–59 CrossRef CAS.
- G. M. Damián, F. Tzompantzi, A. Mantilla, R. P. Hernández and A. H. Gordillo, Appl. Clay Sci., 2016, 121–122, 127–136 CrossRef.
- K. H. Goh, T. T. Lim, A. Banas and Z. L. Dong, J. Hazard. Mater., 2010, 179, 818–827 CrossRef CAS PubMed.
- Y. Qian, P. Wei, P. K. Jiang, J. W. Hao and J. X. Du, Composites, Part B, 2013, 45, 1541–1547 CrossRef CAS.
- H. Y. Li, R. G. Wang, H. L. Hu and W. B. Liu, Appl. Surf. Sci., 2008, 255, 1894–1900 CrossRef CAS.
- Y. Yuan and W. F. Shi, Appl. Clay Sci., 2012, 67–68, 83–90 CrossRef CAS.
- Y. Q. Sun, Y. M. Zhou, Z. Q. Wang and X. Y. Ye, Appl. Clay Sci., 2009, 255, 4497–4502 CAS.
- A. V. Radha, P. V. Kamath and C. Shivakumara, Solid State Sci., 2005, 7, 1180–1187 CrossRef CAS.
- K. M. Zia, M. Barikani, I. A. Bhatti, M. Zuber and M. Barmar, Carbohydr. Polym., 2009, 77, 54–58 CrossRef CAS.
- H. D. Peng, W. C. Tjiu, L. Shen, S. Huang, C. B. He and T. X. Liu, Compos. Sci. Technol., 2009, 69, 991–996 CrossRef CAS.
- M. Herrero, P. Benito, F. M. Labajos, V. Rives, Y. D. Zhu, G. C. Allen and J. M. Adams, J. Solid State Chem., 2010, 183, 1645–1651 CrossRef CAS.
- S. D. Guo, C. Zhang, H. D. Peng, W. Z. Wang and T. X. Liu, Compos. Sci. Technol., 2011, 71, 791–796 CrossRef CAS.
- T. Nogueira, R. Botan, F. Wypych and L. Lona, Composites, Part A, 2011, 42, 1025–1030 CrossRef.
- K. L. Xu, G. M. Chen and J. Q. Shen, Appl. Clay Sci., 2013, 75–76, 114–119 CrossRef CAS.
- C. Nyambo, E. Kandare, D. Y. Wang and C. A. Wilkie, Polym. Degrad. Stab., 2008, 93, 1656–1663 CrossRef CAS.
- C. M. Becker, A. D. Gabbardo, F. Wypych and S. C. Amico, Composites, Part A, 2011, 42, 196–202 CrossRef.
- Q. L. He, T. T. Yuan, S. Y. Wei and Z. H. Guo, J. Mater. Chem. A, 2013, 1, 13064–13075 CAS.
- X. D. Qian, L. Song, Y. Bihe, B. Yu, Y. Q. Shi, Y. Hu and R. K. K. Yuen, Mater. Chem. Phys., 2014, 143, 1243–1252 CrossRef CAS.
- B. Wang, K. Q. Zhou, B. B. Wang, Z. Gui and Y. Hu, Ind. Eng. Chem. Res., 2014, 53, 12355–12362 CrossRef CAS.
- Z. Q. Shen, L. Chen, L. Lin, C. L. Deng, J. Zhao and Y. D. Wang, Ind. Eng. Chem. Res., 2013, 52, 8454–8463 CrossRef CAS.
- W. C. Zhang, X. M. Li, X. Y. Guo and R. J. Yang, Polym. Degrad. Stab., 2010, 95, 2541–2546 CrossRef CAS.
- S. Bourbigot, M. L. Bras, R. Delobel and L. Gengembre, Appl. Surf. Sci., 1997, 120, 15–29 CrossRef CAS.
- X. Wang, L. Song, H. Y. Yang, W. Y. Xing, B. Kandola and Y. Hu, J. Mater. Chem., 2012, 22, 22037–22043 RSC.
- H. B. Zhao, B. W. Liu, X. L. Wang, L. Chen, X. L. Wang and Y. Z. Wang, Polymer, 2014, 55, 2394–2403 CrossRef CAS.
- W. Zhao, J. Liu, H. Peng, J. Y. Liao and X. J. Wang, Polym. Degrad. Stab., 2015, 118, 120–129 CrossRef CAS.
- W. C. Zhang, X. M. Li, H. B. Fan and R. J. Yang, Polym. Degrad. Stab., 2012, 97, 2241–2248 CrossRef CAS.
Footnote |
† Electronic supplementary information (ESI) available. See DOI: 10.1039/c6ra06713a |
|
This journal is © The Royal Society of Chemistry 2016 |