DOI:
10.1039/C6RA06223G
(Communication)
RSC Adv., 2016,
6, 53800-53803
Construction of highly sensitive surface-enhanced Raman scattering (SERS) nanosensor aimed for the testing of glucose in urine†
Received
9th March 2016
, Accepted 16th May 2016
First published on 18th May 2016
Abstract
A facile, highly sensitive “turn on” surface-enhanced Raman scattering (SERS) nanosensor for the detection of glucose has been developed based on the aggregation of 4-mercaptophenylboronic acid (4-MPBA)-decorated silver nanoparticles in specific bonding to glucose, which can be used for the clinical detection of glucose in urine.
The number of people with diabetes increases year by year all over the world, and this disease does serious harm to the human body due to various kinds of inevitable complications, which can cause different degrees of damage to skin, blood vessels, kidneys, nerves, and even the brain. The measurement of blood glucose is the key point in the diagnosis of diabetes. At present, many methods have been developed to measure blood glucose concentration, for example, fluorescence,1,2 colorimetry, surface plasmon resonance,3–5 and electrochemistry.6,7 The clinically relevant glucose range is 0–20.0 mM. Compared with blood glucose measurements, the appearance of glucose in urine is also an important indicator that is relevant to many diseases. Urine testing is more convenient for patients and the urine sample is much easier to obtain than a blood specimen. To measure glucose in urine, a test strip is commonly-used, on which a chromogenic agent shows different colors in response to the oxygen generated by decomposing hydrogen peroxide in the glucose oxidase/glucose catalysis reaction. However, a urine glucose test trip only gives a semi-quantitative result as blue: − (negative), green: + (positive, 0.3–0.5 wt%), yellow-green: ++ (positive, 0.5–1.0 wt%), orange: +++ (positive, 1–2 wt%) and brick red: ++++ (positive, >2 wt%). An accurate analytical method for glucose in urine with a lower detection limit is still in great need, and this would be of crucial significance in early diagnosis. Optical and spectral detection of glucose has shown certain advantages with respect to high efficiency and sensitivity, attracting much research efforts in recent years.8,9 Surface-enhanced Raman-scattering (SERS) spectroscopy, which is of high sensitivity and molecular specificity, has also been used for the analysis and detection of glucose.10–12 Van Duyne and co-workers13,14 have undertaken a systematic study on direct detecting glucose using SERS. They modified a silver surface with a layer of self-assembled 1-decanethiol to adsorb glucose to the Ag substrate's surface. Although this method implements the direct detection of glucose, the selectivity and the sensitivity of this direct-detection approach is limited, since the enrichment of glucose by 1-decanethiol lack specificity and glucose exhibits relatively weak Raman activity.15,16 In order to address these problems, Raman-label detection was introduced to the glucose assays, because these labelled molecules support much stronger SERS signals.17–19 Currently, there is a great deal of literature referring to the selective sensing of glucose over other saccharides, based on the formation of cyclic boronates via glucose.20–22 In these studies, phenylboronic acid derivatives with metal carbonyls or alkyne were usually used as SERS probes.23,24 In this experiment, we chose 4-MPBA, a simpler molecular structure, which is directly used as a probe without further modification. The utilization of 4-MPBA for the design of a glucose sensor is based on the consideration of three aspects. Firstly, the –SH group can form a strong metal–S bond to achieve the modification of metal nanoparticles (NPs) with a 4-MPBA monolayer, which simplifies the assembly process over the metal surface. Secondly, the 4-MPBA covering on the Ag NP surface is SERS-active and the vibration modes of the benzene ring are identifiable in SERS spectra.25 Thirdly, it can selectively bind glucose to form a cyclic boronate as a 4-MPBA/glucose combination with a ratio of 2
:
1. Owing to the above characteristics of 4-MPBA, Du et al. recently developed a glucose sandwich assay, using glucose as a target to bridge a 4-MPBA self-assembled smooth gold-coated slide and dual probe-decorated SERS tags (p-aminothiophenol (PATP) and 4-MPBA).26 Under laser irradiation, the in situ generated Raman reporter, 4,4′-dimercaptoazobenzene (DMAB), via two molecules of PATP on the Ag NP surface, provides intense characteristic SERS peaks and improves the sensitivity of the glucose detection. However, the SERS glucose sensor is still far from being suitable for practical purposes due to an excessively long sensing time of over 3 h. For most solid-supported glucose SERS sensing slides, an adequate incubation time for the 4-MPBA-functionalized wafers in glucose solution is needed to reach the binding equilibrium.
To shorten the sensing time and achieve efficient detection of glucose, in this study, we designed a rapid and highly sensitive glucose nanosensor, based on the crosslinking of 4-MPBA-decorated Ag NPs, in response to glucose in urine. 4-MPBA, acting as a Lewis acid, can react with water, causing boron atoms to change from neutral sp2 to an anion form of sp3 (Scheme 1(a)). The 4-MPBA in the dissociated state can easily combine with vicinal diol to form a charged complex.27 The complex is thermodynamically stable. Glucose, as a polyhydroxy aldehyde, contains two pairs of adjacent hydroxyl groups, and each pair of adjacent hydroxyl groups can combine with a molecule of the tetrahedral 4-MPBA. Thus, they can produce a six-membered cyclic lactone compound, as shown in Scheme 1(c). Based on the recognition action between 4-MPBA and glucose, we can design this nanosensor, in which 4-MPBA molecules acting as probes are exactly laid in the nanogaps that generate an ultrahigh localized electronic field. Owing to the formation of “hot spots”, the sensitivity of this SERS method can be greatly improved.28
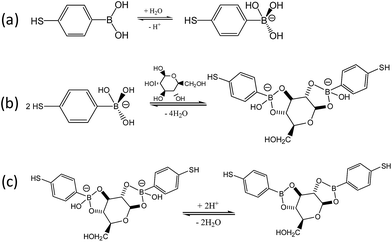 |
| Scheme 1 The process of two molecules of 4-MPBA binding one molecule of glucose. | |
Our designed glucose nanosensor is made from the 4-MPBA-decorated Ag NPs. A 49 nm Ag NP colloid was first synthesized using Lee's method, as described previously,29 and it has a plasmon band located at 421 nm. To prepare the 4-MPBA-functionalized Ag NP colloid, we added 10 mL of Ag NP colloid (7.2 × 10−12 mol L−1) in a 4-MPBA ethanol aqueous solution (2.0 μL, 1.0 mM) under stirring. After reacting overnight, we removed the unadsorbed 4-MPBA by centrifugation at 7000 rpm for 10 min, and the precipitates were redispersed in a phosphate buffer solution with a pH of 9.18 in a 60 °C water bath for half an hour. After 4-MPBA coating, the plasmon band has a 5 nm red shift (Fig. S1(a)†). The glucose-sensing process was carried out by adding different concentrations of glucose solutions (0.1 mL) to the above 4-MPBA-functionalized Ag NP colloids (0.9 mL). Scheme 2 shows the glucose sensing process. Owing to the 4-MPBA/glucose bonding ratio of 2
:
1, we can obtain a crosslinking aggregation of Ag NPs. The plasmon spectrum of the sensing solution shows the plasmon peak of Ag NPs moving to 431 nm with band broadening (Fig. S1(a)†), indicating the typical plasmon feature of Ag NP aggregates. A TEM image (Fig. S1(b)†) also proves the formation of large Ag NP aggregates. Under 532 nm laser irradiation, we measured the SERS spectra of the casting films of the 4-MPBA-modified Ag colloids before and after the addition of glucose, on a glass slide.
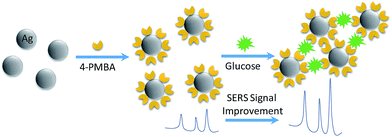 |
| Scheme 2 The glucose sensing mechanism based on a ‘turn-on’ SERS response. | |
The response time is an important parameter for glucose sensor, based on the consideration of practical purposes, and this has been evaluated. Fig. 1(a) shows the measured SERS spectra of 4-MPBA after a 20 mM glucose solution was added into the 4-MPBA-functionalized Ag NP colloid. The plotted SERS intensities at 1563 cm−1, along with the sensing times, is shown in Fig. 1(b).
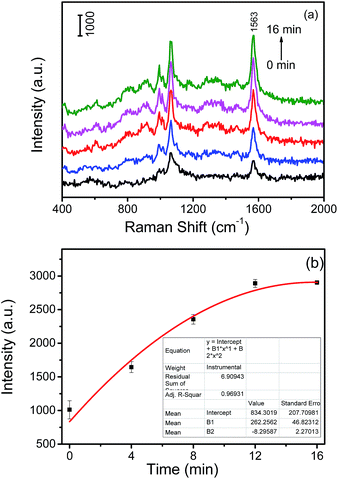 |
| Fig. 1 (a) Time-dependent SERS spectra of the 4-MPBA-functionalized Ag NPs for the response to glucose (20 mM). (b) The intensity at 1563 cm−1 of 4-MPBA versus the sensing time. | |
It was found that the SERS signal of 4-MPBA increases with time and reaches a platform after 12 min. So, our glucose sensor has a response time of 12 min, which is much shorter than that of other solid-supported SERS sensing chips, in which a longer sensing time of 3 h is needed for the binding equilibrium.23,24,26
This glucose sensor can achieve highly sensitive quantitative detection of glucose within the normal physiological range. SERS spectra of different concentrations of glucose solutions were measured using this sensor and are shown in Fig. 2(a). Fig. 2(b) shows a linear relationship between the glucose concentration and the changed intensity of the 1563 cm−1 peak of 4-MPBA by subtracting the blank one. The fitting equation is y = 359 + 70.8x, with a correlation coefficient of 0.990. In addition, the lowest detectable concentration is as low as 1.0 μM (signal to noise ratio = 3
:
1) (Fig. S2 in ESI†) and this SERS nanosensor shows great reproducibility (Fig. S4 in ESI†).
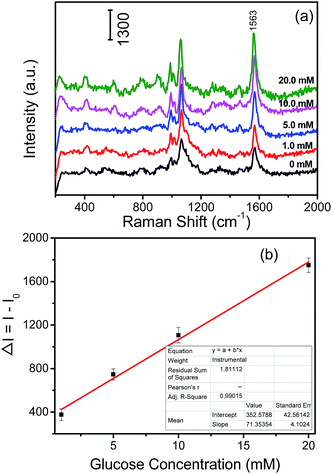 |
| Fig. 2 (a) SERS spectra for sensing different concentrations of glucose. (b) A linear relationship between the glucose concentration and the intensity of the 1563 cm−1 peak of 4-MPBA after subtracting the blank one. ΔI = I − I0, while I and I0 stand for the SERS intensity of 4-MPBA at 1563 cm−1, after and before adding different concentrations of glucose, respectively. | |
Fig. 3 shows the selectivity of the present sensor. We used other sugar solutions (50 mM), e.g. mannose, galactose, sucrose and fructose, comparing with 1.0 mM of glucose solution. The result shows that the changes in the SERS signal of 4-MPBA in response to other sugars are much less than that for glucose, though the concentration of glucose is 1/50 lower than other sugars, suggesting that our designed sensor has high selectivity.
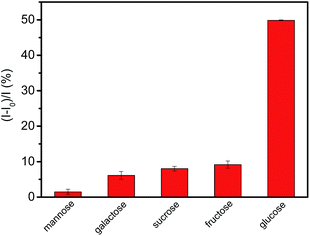 |
| Fig. 3 Comparison of the SERS intensity at 1563 cm−1 of 4-MPBA modified on Ag NPs in the presence of different saccharides, including mannose (50 mM), galactose (50 mM), sucrose (50 mM), fructose (50 mM) and glucose (1.0 mM). | |
To demonstrate the potential practicality of our sensor, we employed it to trace glucose in undiluted urine samples with an additional standard method. Humanity self-monitoring of urine was regarded as a convenient method of equity to blood glucose due to the advantages of painlessness and low cost. The results are listed in Table 1, from which the recovery rate of glucose ranges from 85–111%. Thus, the effective and accurate determination of glucose concentration in urine samples strongly suggests that this glucose nanosensor offers great potential in clinical diagnosis.
Table 1 Recovery rate of glucose in the urine samples. Each sample is measured five times
|
Real value (mM) |
Measured valued (mM) |
Recovery (%) |
1 |
0.2 |
0.1700 ± 0.0028 |
85.0 ± 1.4 |
2 |
0.4 |
0.3700 ± 0.0024 |
92.5 ± 0.6 |
3 |
0.8 |
0.8900 ± 0.0040 |
111.3 ± 0.5 |
4 |
1.0 |
1.050 ± 0.031 |
105.0 ± 3.1 |
5 |
5.0 |
4.95 ± 0.18 |
99.0 ± 3.6 |
6 |
20.0 |
19.96 ± 0.14 |
99.8 ± 0.7 |
In summary, owing to the plasmonic properties of noble metal nanoparticles in a particular spatial arrangement, which is constructed from Ag NPs aggregation system that has high SERS activity, we designed a new type of highly sensitive and selective glucose sensor in the aqueous phase. In conventional methods, additional aggregating agents are needed to induce silver nanoparticles to gather, which leads to the introduction of interfering ions. However, in the system we designed, we only use the properties of 4-MPBA itself to build a clustered system, which causes the formation of “hot spots” and an improvement in the SERS signal. 4-MPBA not only acted as a SERS labelling molecule, but it can also specifically recognize and capture glucose, realizing the quantitative determination of glucose in urine. The sensitivity is up to 1.0 μM. This prepared nanosensor has an easy handling procedure and a short response time (12 min). It is expected to become a new, efficient, simple, and economical sensor for the clinical detection of glucose in urine.
Acknowledgements
This work was supported by the National Instrumentation Program (NIP) of the Ministry of Science and Technology of China (Grant No. 2011YQ03012408), the National Natural Science Foundation of China (Grant No. 21373096, 21573087 and 21573092), and Science and Technology Development Program Funded Projects of Jilin province.
References
- Y. Yi, J. Deng, Y. Zhang, H. Li and S. Yao, Chem. Commun., 2013, 49, 612–614 RSC.
- A. Hu, Y. Liu, H. Deng, G. Hong, A. Liu, X. Lin and W. Chen, Biosens. Bioelectron., 2014, 61, 374–378 CrossRef CAS PubMed.
- X. Yang, Y. Yu and Z. Gao, ACS Nano, 2014, 8, 4902–4907 CrossRef CAS PubMed.
- C. Radhakumary and K. Sreenivasan, Anal. Chem., 2011, 83, 2829–2833 CrossRef CAS PubMed.
- H. He, X. Xu, H. Wu and Y. Jin, Adv. Mater., 2012, 24, 1736–1740 CrossRef CAS PubMed.
- S. Lee, B. S. Ringstrand, D. A. Stone and M. A. Firestone, ACS Appl. Mater. Interfaces, 2012, 4, 2311–2317 CAS.
- A. J. Bandodkar, W. Jia, C. Yardimci, X. Wang, J. Ramirez and J. Wang, Anal. Chem., 2015, 87, 394–398 CrossRef CAS PubMed.
- G. Qi, C. Fu, G. Chen, S. Xu and W. Xu, RSC Adv., 2015, 5, 49759–49764 RSC.
- G. Qi, K. Jia, C. Fu, S. Xu and W. Xu, J. Opt., 2015, 17, 114020 CrossRef.
- B. Sharma, R. R. Frontiera, A. Henry, E. Ringe and R. P. Van Duyne, Mater. Today, 2012, 15, 16–25 CrossRef CAS.
- W. Xie, P. Qiu and C. Mao, J. Mater. Chem., 2011, 21, 5190–5202 RSC.
- X. Sun and H. Li, Curr. Nanosci., 2016, 12(2), 175–183 CrossRef CAS.
- C. R. Yonzon, C. L. Haynes, X. Zhang, J. T. Walsh Jr and R. P. Van Duyne, Anal. Chem., 2004, 76, 78–85 CrossRef CAS PubMed.
- K. E. Shafer-Peltier, C. L. Haynes, M. R. Glucksberg and R. P. Van Duyne, J. Am. Chem. Soc., 2003, 125, 588–593 CrossRef CAS PubMed.
- J. Kaminsky, J. Kapitan, V. Baumruk, L. Bednarova and P. Bour, J. Phys. Chem. A, 2009, 113, 3594–3601 CrossRef CAS PubMed.
- A. Kaminska and R. Hołyst, J. Raman Spectrosc., 2012, 43, 959–962 CrossRef CAS.
- B. Küstner, M. Gellner, M. Schütz, F. Schöppler, A. Marx, P. Ströbel, P. Adam, C. Schmuck and S. Schlücker, Angew. Chem., Int. Ed., 2009, 48, 1950–1953 CrossRef PubMed.
- Z. Wang, S. Zong, W. Li, C. Wang, S. Xu, H. Chen and Y. Cui, J. Am. Chem. Soc., 2012, 134, 2993–3000 CrossRef CAS PubMed.
- Y. W. C. Cao, R. Jin and C. A. Mirkin, Science, 2002, 297, 1536–1540 CrossRef CAS PubMed.
- J. S. Hansena, J. B. Christensena, J. F. Petersena, T. Hoeg-Jensen and J. C. Norrild, Sens. Actuators, B, 2012, 161, 45–79 CrossRef.
- H. Eggert, J. Frederiksen, C. Morin and J. C. Norrild, J. Org. Chem., 1999, 64, 3846–3852 CrossRef CAS.
- W. Yang, H. He and D. G. Drueckhammer, Angew. Chem., Int. Ed., 2001, 40, 1714–1718 CrossRef CAS.
- K. V. Kong, Z. Lam, W. K. O. Lau, W. K. Leong and M. Olivo, J. Am. Chem. Soc., 2013, 135, 18028–18031 CrossRef CAS PubMed.
- K. V. Kong, C. J. H. Ho, T. Gong, W. K. O. Lau and M. Olivo, Biosens. Bioelectron., 2014, 56, 186–191 CrossRef CAS PubMed.
- J. Ye, Y. Chen and Z. Liu, Angew. Chem., Int. Ed., 2014, 53, 10386–10389 CrossRef CAS PubMed.
- X. Bi, X. Du, J. Jiang and X. Huang, Anal. Chem., 2015, 87, 2016–2021 CrossRef CAS PubMed.
- S. Hayik, K. L. Bhat and C. W. Bock, Struct. Chem., 2004, 15, 133 CrossRef CAS.
- H. Xu, E. J. Bjerneld, M. Käll and L. Börjesson, Phys. Rev. Lett., 1999, 83, 4357–4360 CrossRef CAS.
- P. C. Lee and D. Meisel, J. Phys. Chem. C, 1982, 86, 3391–3395 CrossRef CAS.
Footnote |
† Electronic supplementary information (ESI) available: Materials, plasmon spectra, TEM images, lowest detectable concentration, selectivity and reproducibility of SERS detection. See DOI: 10.1039/c6ra06223g |
|
This journal is © The Royal Society of Chemistry 2016 |