DOI:
10.1039/C6RA06103F
(Paper)
RSC Adv., 2016,
6, 42911-42916
Poly(norbornyl-NDIs) as a potential cathode-active material in rechargeable charge storage devices†
Received
8th March 2016
, Accepted 21st April 2016
First published on 25th April 2016
Abstract
Two pendant-type naphthalene diimide (NDI) polymers bearing a polynorbornene backbone were prepared and their electrochemical properties explored. The NDI-based polymers displayed reversible redox responses in 1 M LiClO4 γ-butyrolactone electrolyte. Polymer 1, bearing a N-methyl group, exhibited remarkable charge-storage capabilities which demonstrated its potential usefulness as an organic cathode-active material in rechargeable devices with excellent performances such as large charge capacity, high rate capability, and charge–discharge cyclability.
Introduction
Naphthalene diimides (NDIs) have been widely investigated as air-stable n-type organic semiconductors with high electron-transporting capability based on their electron-accepting π-conjugated structure and high stacking propensity.1,2 There have been many reports on their potential applications in electronic and photo-electronic devices,3,4 such as organic light-emitting diodes,5 organic field effect transistors,6 and photovoltaic cells.7 For example, the NDI moiety works as an electron or a charge accepting unit leading to efficient charge separation capability in porphyrin–NDI dyads.8 In addition to studies of NDIs in a solid or solvent-free state, NDIs perform electron-accepting or electrochemical redox functionality in the presence of electrolytes or in wet devices as typically applied to sensors.9 We have previously reported that the electron deficient aromatic ring of NDI plays the role of an acceptor in photo-induced electron transfer and receptor of anions accompanied with fluorescence, absorption and intensity change in the detection of protons10 and fluoride ions.11 NDIs display reversible negative potential responses in electrolytes at comparable potentials to those of quinone and viologen derivatives.12 The latter has been well studied as an organic-based functional electrode to the application of redox catalysts and sensors.13 We have extensively studied the organic redox polymer-based rechargeable devices14 and recently reported that phthalimide and pyromellitic polyimides formed on carbon nanofibers work as an electrode-active material.15 The polyimides exhibited redox capacities around 100 mA h g−1 and showed good cycle stability; however, the practical charge capacity was not high because the amount of additive carbon as a current collector in the nanocomposites was large.
Li-ion battery technology is currently based on a mechanism where Li cations are intercalated into an anode and a cathode-active material during charging and discharging, respectively, with the Li cations shuttled between the cathode and the anode.16 Quinone derivatives such as anthraquinone have been examined as an organic cathode-active material in rechargeable Li batteries.17 However, their redox potentials are located at large negative potentials (2.2 V vs. Li/Li+ for anthraquinone), and the output voltage of the examined batteries is relatively low (i.e. <2.2 V). We anticipated that the application of NDI derivatives with a large positive redox potential (2.5 V vs. Li/Li+)18 and large charge capacity (e.g. 1,4,5,8-naphthalenetetracarboxdiimide: 201 mA h g−1) will display high output voltage and relatively high capacity as the cathode-active material of Li-ion batteries. In related research, Zhan et al. recently reported that a polyimide composed of repeating NDI moieties in the backbone worked as a cathode active material with a voltage of 2.47 V and a capacity of 202 mA h g−1 in the rechargeable Li batteries.19 However, the reported NDI backbone polymer lacked affinity with common electrolytes due to the planar backbone structure of the NDI polymer. This limited comparison of the above data as the unusual, highly volatile and flammable mixture of 1,3-dioxolane and dimethoxyethane solvent was required to undertake measurement. Recently monomeric NDI derivatives core-substituted with cyano, fluoro, and dimethylamine groups were tested as a cathode active material in Li-ion batteries in which the relationship of their redox potentials with the substituents on the naphthalene core were described. Unfortunately, the monomeric NDIs eluted out into the electrolyte and as a result the battery performance lacked durability.18
Immobilization of the redox active sites in the electrode, or on a current collector, is a crucial issue for the charge-storable electrode-active material. Ideally, the redox-active components are not to be eluted into the electrolyte as this causes self-discharge, but are instead to be swollen or solvated with electrolyte solutions for interpenetration of the counter ions in the electrode. A pendant-type introduction of redox-active groups within the polymers have been proved by us to provide redox active electrodes both with a high concentration of the redox sites, or a high capacity and a high-rate charging property using polymers bearing TEMPO (2,2,6,6-teramethylpiperidine-1-oxyl)20 and anthraquinone groups.21 Extending the redox molecules to NDIs, to form pendant-type polymeric analogues could also be an effective way to achieve swollen NDI polymers in spite of their typical planar and easily stacking properties. Technically, the choice of polymer then becomes important. Norbornene derivatives are known to polymerise via ROMP (ring-opening metathesis polymerization) in the presence of Grubbs catalyst, which is tolerant for broad functional groups. The bulky norbornene structure could be expected to improve the solubility of NDI for processing, by inhibiting molecular stacking. Furthermore, the polynorbornene backbone formed by ROMP contains a double bond, which could be crosslinked, if necessary, by UV irradiation with a photo-crosslinking reagent to form insoluble polymer layer.22
In this paper, we report the synthesis and properties of pendant-type NDI polymers, bearing a polynorbornene backbone. As the theoretical capacity of the repeating unit (139 mA h g−1) is relatively high in comparison with those of conventional cathode active materials, we anticipated that the norbornene-NDI polymers efficiently work as cathode active materials to possibly perform high-rate charging/discharging capability, relatively high output voltage and charge capacity, and high charging/discharging cyclability in charge storage devices including Li-ion batteries.
Experimental
Materials
The photo-crosslinker, 2,6-bis(p-azidobenzal)-4-t-amylcyclohexanone, was obtained from Toyo Gosei Co. Vapor-grown carbon nanofibers (VGCF) and the binder, poly(vinylidene difluoride) (PVdF) resin (KF polymer), were purchased from Showa Denko Co. and Kureha Chemical Co., respectively. All starting materials, organic reagents, catalysts, electrolytes, and organic solvents were purchased from Tokyo Chemical Industry Co., Kanto Chemical Co., and Sigma-Aldrich Japan and were used without further purification. Compound 3 was synthesized according to literature procedures.23 Its spectroscopic characterization matched that of literature.
Synthesis of NDI monomers and polymers
Synthesis of N-(2-ethylhexyl)naphthalene-1,4,5,8-tetracarboxylic monoanhydride monoimide (4). Compound 4 was synthesized by modifying a previous report.24 1,4,5,8-Naphthalenetetracarboxylic dianhydride (2.00 g, 7.46 mmol) was suspended in DMF (34 mL), and 2-ethylhexylamine (1.11 mL, 6.78 mmol) was added. The reaction mixture was sonicated, then heated to 80 °C, and stirred overnight. To the resulting mixture was added 1 M HCl to pH 1 and stirred for 5 min, and then a large amount of water was added to precipitate the product. The precipitate was filtered off and the residue was washed with methanol (until filtrate was colourless). The residue was then dissolved in CHCl3 and washed with brine. The organic layer was dried over Na2SO4 and evaporated to yield 4 (1.74 g. 68%) as a yellowish solid. Melting point 167 °C. 1H NMR (DMSO-d6, 500 MHz) δ = 8.70 (ABq, J = 7.4 Hz, 4H), 4.00 (m, 2H), 1.86 (m, 1H), 1.29 (m, 8H), 0.87 (m, 6H); 13C NMR (DMSO-d6, 500 MHz): δ = 162.8, 159.7, 131.8, 130.5, 127.0, 126.2, 124.6, 123.8, 43.8, 37.2, 30.1, 28.1, 23.5, 22.4, 13.5, 10.5; mass spectum (−ive ESI): m/z 379.4 (M, calcd), 379.7 (found); anal. calcd for C22H21NO5: C, 69.6; H, 5.6; N, 3.7%. Found: C, 69.0; H, 5.2; N, 3.6%.
Synthesis of N-methyl-N′-(5′-norbornene-2′-methyl) naphthalene-1,4,5,8-tetracarboxylic diimide (5). Compound 3 (1.00 g, 3.56 mmol) was suspended in DMF (18 mL), and 5-norbornene-2-methylamine (548 μL, 4.27 mmol; endo/exo = 80/20) and trimethylamine (595 μL, 4.27 mmol) were added under a nitrogen atmosphere. The reaction mixture was heated to 90 °C and stirred overnight. To the resulting solution was added water (20 mL) and the precipitate was filtered. The residue was dissolved in CHCl3 and washed with 1 M HCl, water, and brine, successively. The organic layer was dried over Na2SO4 and evaporated to dryness. The crude product was purified by silica gel column chromatography using a CHCl3/C2H5OAc (9/1 in v/v) eluent to yield 5 (803 mg, 58%) as a greenish solid (endo/exo = 80/20). Melting point 274 °C. 1H NMR (CDCl3, 500 MHz) δ = 8.77 (ABq, J = 7.3 Hz, 4H, endo), 6.31 (dd, J = 5.9 Hz, 3.6 Hz, 0.8H, endo), 6.25 (dd, J = 5.9 Hz, 3.6 Hz, 0.8H, endo), 6.06 (dd, J = 5.9 Hz, 3.6 Hz, 0.2H, exo), 6.00 (dd, J = 5.9 Hz, 3.6 Hz, 0.2H, exo), 3.98 (m, 2H), 3.61 (s, 3H), 2.83 (s, 1H), 2.76 (s, 1H), 2.61 (m, 1H), 1.94 (m, 1H), 1.43 (m, 1H), 1.21 (m, 1H), 0.81 (m, 1H); 13C NMR (CDCl3, 500 MHz): δ = 163.1, 163.0, 137.7, 133.0, 131.0, 126.8, 126.6, 126.5, 49.6, 44.7, 44.6, 42.4, 38.0, 37.7, 30.6, 27.4; mass spectum (−ive ESI): m/z 386.4 (M, calcd), 387.1 (found); anal. calcd for C23H18N2O4: C, 71.5; H, 4.7; N, 7.3%. Found: C, 71.2; H, 4.2; N, 7.2%.
Synthesis of N-(2-ethylhexyl)-N′-(5′-norbornene-2′-methyl)naphthalene-1,4,5,8-tetracarboxylic diimide (6). Compound 6 was synthesized in a similar fashion to that of 5. The crude product was purified by silica gel column chromatography with a CHCl3 eluent to yield 6 (685 mg, 54%) as a pink solid (endo/exo = 80/20). Melting point 220 °C. 1H NMR (CDCl3, 500 MHz, ppm) δ = 8.76 (m, 4H), 6.31 (dd, J = 5.9 Hz, 3.6 Hz, 0.8H, endo), 6.25 (dd, J = 5.9 Hz, 3.6 Hz, 0.8H, endo), 6.06 (dd, J = 5.9 Hz, 3.6 Hz, 0.2H, exo), 6.00 (dd, J = 5.9 Hz, 3.6 Hz, 0.2H, exo), 4.15 to 3.98 (m, 4H), 2.83 (s, 1H), 2.76 (s, 1H), 2.61 (m, 1H), 1.94 (m, 2H), 1.47 to 1.30 (m, 10H), 1.23 (d, J = 8.6 Hz, 1H), 0.94 (t, J = 7.5 Hz, 3H), 0.88 (t, J = 7.2 Hz, 3H), 0.80 (m, 1H); mass spectum (−ive ESI): m/z 484.6 (M, calcd), 484.8 (found); anal. calcd for C30H32N2O4: C, 74.4; H, 6.7; N, 5.8%. Found: C, 74.2; H, 6.8; N, 5.8%.
Ring-opening metathesis polymerisation
A solution of Grubbs secondary catalyst (2.83 mg, 3.33 μmol) in CHCl3 (0.5 mL) was added to a solution of 5 (100 mg, 0.26 mmol) in CHCl3 (4.66 mL), and the mixture was stirred overnight at 60 °C under a nitrogen atmosphere. The resulting precipitate was filtered and washed with CHCl3 and hexane. The precipitated polymer powder was purified by reprecipitation from 1,1,1,3,3,3-hexafluoro-2-propanol (HFIP) into methanol to yield 1 (93 mg) as a pale powder. Mn = 7200, Mw/Mn = 1.7; 1H NMR (HFIP-d2, 500 MHz) δ = 8.69 (bm, 4H), 5.40 (bm, 2H), 3.59 (bm, 3H), 2.93 (bm, 4H), 1.74 (bm, 1H), 1.33 (bm, 3H), 0.91 (bm, 1H).
A solution of Grubbs secondary catalyst (2.83 mg, 3.33 mmol) in CHCl3 (0.5 mL) was added to a solution of 6 (100 mg, 0.21 mmol) in CHCl3 (3.62 mL), and the mixture was stirred overnight at 60 °C under a nitrogen atmosphere. The polymerization mixture was poured into hexane, and the precipitated polymer powder was purified by reprecipitation from chloroform into methanol to yield 2 (61 mg) as a brownish powder. Mn = 51
000, Mw/Mn = 3.1; 1H NMR (CDCl3, 500 MHz, ppm) δ = 8.57 (bm, 4H), 5.35 (bm, 2H), 4.05 (bm, 4H), 2.52 (bm, 2H), 1.85 (bm, 1H), 1.52 (bm, 2H), 1.29 (bm, 10H), 0.87 (bm, 7H).
Ring-opening metathesis polymerisation
To a solution of the NDI polymer (HFIP for 1 or CHCl3 for 2) were added a solution of the photo-crosslinker, and the mixed solutions were drop-cast on a glassy carbon substrate and polymerized by UV irradiation (Ushio Inc. USH-250D, 40 mJ cm−2). The layer thickness was estimated by using a contact stylus profiler (KLA Tencor P-15).
Photo-crosslinked polymer/carbon composite electrodes were prepared as follows: a NDI polymer (10 mg) and a cross-linker (0.1 mg) were mixed with VGCF and a binder powder in the presence of NMP as a solvent. The resulting black clay was spread onto an aluminum sheet or a glassy carbon substrate and dried in vacuo. Photo-crosslinking was then carried out by UV irradiation (5 J cm−2) for 250 s (20 mW cm−2).
Electrochemical measurements
The electrochemical measurements were performed under nitrogen and 0.1 M tetrabutylammonium hexafluorophosphate in CH2Cl2 as the electrolyte. A potentiostat system (BAS Inc. ALS760E) was used for the cyclic voltammetry, chronopotentiometry and chronoamperometry. A platinum disk and coiled platinum wire were used as the working and counter electrode, respectively, and the working potential was measured vs. a Ag/AgCl reference electrode. Cyclic voltammograms of the polymer electrodes were measured in γ-butyrolactone (GBL) solution in the presence of 1 M lithium perchlorate (LiClO4) as the supporting electrolyte.
Cell fabrication
Coin type test cells were fabricated by sandwiching the electrolyte layer of 1 M LiClO4 in GBL with a lithium metal anode and the photo-crosslinked polymer cathode, using a separator film (Celgard #3501 from Hohsen Co.) in a glove box under an atmosphere of dry argon. The cyclability performance of the fabricated cell was tested by repeated charge–discharge galvanostatic cycles. The cut-off potentials were 1.0–3.5 V vs. Li/Li+.
Measurements
1H and 13C NMR spectra were recorded on a JEOL ECX-500 spectrometer with chemical shifts downfield from tetramethylsilane as the internal standard. UV-vis spectra were measured by an UV-670 UV-vis spectrophotometer. Surfaces and a cross section of the polymers were observed by scanning electron microscopy (SEM). Samples were scribed on the glass substrate side and split in liquid nitrogen to observe the cross-section SEM-images (HITACHI High-Tech S-3000N). Mass spectra were obtained using a JMS-GCMATE II or Bruker Daltonics Autoflex. Molecular weight measurements were calculated using gel permeation chromatography using a TOSOH HLC-8220 instrument with CHCl3 as the eluent. Calibration was achieved against polystyrene standards.
Results and discussion
Ring-opening metathesis polymerization of the NDI-norbornene gave the corresponding NDI polymers with high molecular weight (Scheme 1), and the NDI groups in monomers were tolerant for the Grubbs catalyst. The methyl-substituted NDI polymer 1 was found to be sparingly soluble in most common solvents so measurements were performed in HFIP. Polymer 2 bearing the large 2-ethylhexyl side chains was very soluble in CHCl3 and common solvents. The solutions yielded homogeneous and tough polymer layers on every substrate tested by a drop-casting or a spin-coating (i.e. glass, ITO, glassy carbon). A different aggregation property was observed by SEM (Fig. 1). The polymer solutions showed two absorption peaks around 380 and 360 nm to be ascribed to the NDI moieties. On the other hand in the solid layers, red shift of the peaks was observed for 1 but not for 2 (inset of Fig. 2), probably because the bulky alkyl chains prevent aggregation of the NDIs.
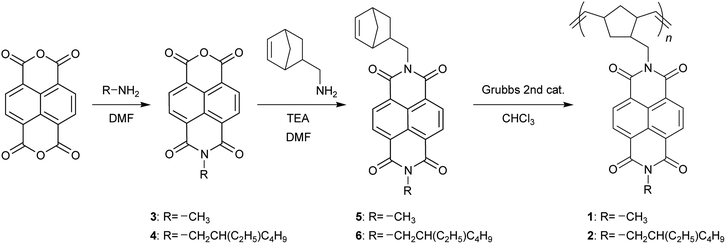 |
| Scheme 1 Synthetic route used to prepare compounds 1 and 2. | |
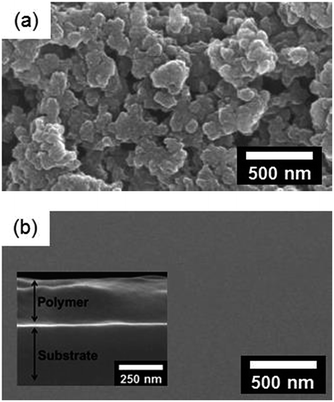 |
| Fig. 1 SEM images of the drop-cast 1 (a) and 2 (b) layers on glass substrates. Inset: cross-section SEM image of 2. | |
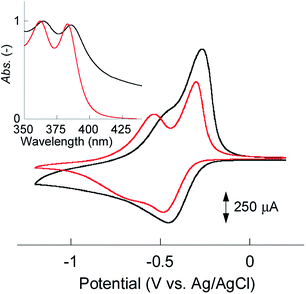 |
| Fig. 2 Cyclic voltammograms of 1 (black) and 2 (red) layers in 1 M LiClO4 using GBL electrolyte at a scan rate of 25 mV s−1. Inset: UV-vis spectra of solid films of 1 and 2 on a glass substrate. | |
The fact that the polynorbornene backbone has a double bond between the cyclopentane residues, allows reaction with a photo-crosslinker by UV irradiation to form new substrate-bound polymers in the reduced state. The polymer layers were obtained by drop-casting of a HFIP solution of 1, or a CHCl3 solution of 2, with 1 wt% photo-crosslinker on a glassy carbon substrate (active surface area = 2.5 cm2), which was then dried under ambient condition, and photo-crosslinked by UV irradiation. As expected, the crosslinked polymers were swollen with, but insoluble in GBL, one of the most common electrolytes in Li-ion batteries, during the redox process (Scheme 2). The previous papers18,19 suggested that an enolate structure was formed on the reduced state of NDI polymers. Li cations could associate with the enolate anion for charge compensation. Charges propagated throughout the polymers occur by a self-electron exchange reaction accompanied by the diffusion of Li cations. Both of the polymer layers were ca. 100 nm thick and displayed reversible redox responses without current decrease during the repeating scans (Fig. 2). Cross-linked polymer 1 displayed two oxidation peaks at −0.45 and −0.27 V, and one broad reduction wave at −0.45 V; on the other hand, cross-linked polymer 2 presented two oxidation and reduction waves (Fig. 2). This is consistent with the findings of the UV study and could be explained by the interactions or stacking of the NDI moieties in 1.
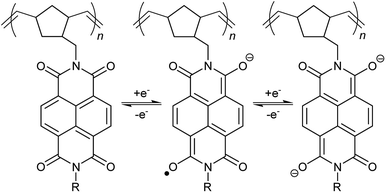 |
| Scheme 2 Redox reactions of the norbornene-NDI polymer. | |
Diffusion coefficients D in 1 and 2 were estimated from chronoamperograms of the polymer layers using the Cottrell equation in the potential range of 0 to −1.2 V vs. Ag/AgCl (Fig. 3). The D values of the NDI polymers were on the order of 10−10 cm2 s−1, almost comparable with those of our previously reported radical polymers, 1.2 × 10−10 cm2 s−1 for poly(2,2,6,6-tetramethylpiperidinyloxy-4-yl acrylamide) (PTAm) in the aqueous electrolyte25 and 5.3 × 10−10 cm2 s−1 for poly[2,3-bis(2′,2′,6′,6′-tetramethylpiperidinyl-N-oxyl-4′-oxycarbonyl)-5-norbornene] (PTNB) in the acetonitrile electrolyte which are used as an electrode-active material in rechargeable charge storage devices.26 The larger De of 1 compared with 2 could be considered that the methyl group substituted NDI moiety in 1 was partially stacked to cause a more efficient self-electron exchange and charge-transport process.
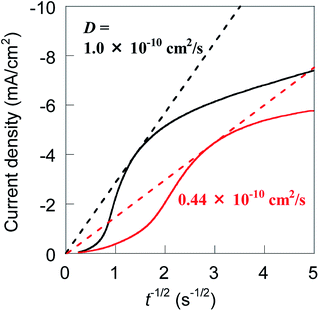 |
| Fig. 3 Cottrell plots of the redox responses of the 1 (black) and 2 (red) layers in 1 M LiClO4 GBL electrolyte. | |
The rechargeable/charge storage performance was tested using the coin-cell fabricated with the NDI polymers as the cathode, lithium foil as the anode, with a microporous membrane as a separator, and 1 M LiClO4 GBL electrolyte. Cyclic voltammograms of the two coin-cells displayed reversible redox responses around E1/2 = 2.5 V vs. Li/Li+ (inset of Fig. 5), which coincided with the potentials of 1 and 2 vs. Li/Li+. Charging–discharging curves of the cells exhibited a plateau voltage at the E1/2 and their coulombic efficiencies (the ratio of discharging vs. charging capacity) were >99%. Their power performance was examined by measuring the discharging curves with various current densities or C rates (1C rate is defined as the charge–discharge current which takes 1 h to charge and discharge the entire theoretical capacity). The discharging capacity for the cell containing crosslinked 1 was 138 mA h g−1 at 5C (the rate to fully charge within 12 min) (Fig. 4), suggesting that almost all of the NDI moiety contributed to charge storage. The discharging capacity maintained at 70% at 20C (the charging for 3 min). This high-rate charging capability could be ascribed to its high diffusion coefficient, D. The cells also exhibited excellent cyclability (Fig. 5). The initial capacity was maintained (over 90%) even after 1000 charging–discharging cycles at 10C (Fig. S1†).
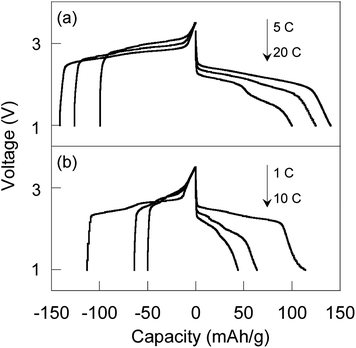 |
| Fig. 4 Charging and discharging curves of the coin-cells fabricated with crosslinked 1 (a) and 2 (b) cathode. | |
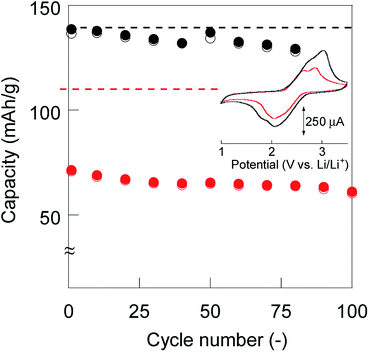 |
| Fig. 5 Cycle performance of the coin-cells fabricated with 1 (black) and 2 (red) cathode. Dashed lines show theoretical capacities. Inset: cyclic voltammograms of crosslinked 1 and 2 composite cathodes in the coin-cells. | |
Conclusions
The high charge capacity, rate capability, and cyclability of the test cell showed a potential for the pendant-type NDI polymers to be applicable as a cathode active material in a rechargeable Li battery. While large alkyl chains assist with processability, it appears they hinder performance of pendant systems. This, together with the pendant approach, are useful design concepts for new generation cathode-active materials. The cell performance, such as the output voltage and the cyclability, could be further enhanced by NDI core-substitution, perhaps with electron-withdrawing groups, and more extensive crosslinking of the norbornene backbone. Furthermore the pendant-type NDI polymers have a potential to be examined as a cathode-active material in a heavy metal-free Li-ion batteries.
Acknowledgements
This work was partially supported by a Grant-in-Aid for Scientific Research (No. 24225003) from MEXT, Japan, and the Australian Research Council through the Discovery program (DP130101861). The collaboration is the direct result of the Top Global University Project from MEXT, Japan. Y. S. acknowledges the Leading Graduate Program in Science and Engineering, Waseda University from MEXT, Japan.
Notes and references
- H. E. Katz, A. J. Lovinger, J. Johnson, C. Kloc, T. Siegrist, W. Li, Y.-Y. Lin and A. Dodabalapur, Nature, 2000, 404, 478–481 CrossRef CAS PubMed.
- B. A. Jones, A. Facchetti, M. R. Wasielewski and T. J. Marks, J. Am. Chem. Soc., 2007, 129, 15259–15278 CrossRef CAS PubMed.
- S. V. Bhosale, C. H. Jania and S. J. Langford, Chem. Soc. Rev., 2008, 37, 331–342 RSC.
- S.-L. Suraru and F. Würthner, Angew. Chem., Int. Ed., 2014, 53, 7428–7448 CrossRef CAS PubMed.
- L. J. Rozanski, E. Castaldelli, F. L. M. Sam, C. A. Mills, G. J.-F. Demets and S. R. P. Silva, J. Mater. Chem. C, 2013, 1, 3347–3352 RSC.
- N. B. Kolhe, A. Z. Ashar, K. S. Narayan and S. K. Asha, Macromolecules, 2014, 47, 2296–2305 CrossRef CAS.
- T. Earmme, Y.-J. Hwang, N. M. Murari, S. Subramaniyan and S. A. Jenekhe, J. Am. Chem. Soc., 2013, 135, 14960–14963 CrossRef CAS PubMed.
- B. Robotham, K. A. Lastman, S. J. Langford and K. P. Ghiggino, J. Photochem. Photobiol., A, 2013, 251, 167–174 CrossRef CAS.
- S. Guha, F. S. Goodson, L. J. Corson and S. Saha, J. Am. Chem. Soc., 2012, 134, 13679–13691 CrossRef CAS PubMed.
- H. F. Higginbotham, R. P. Cox, S. Sandanayake, B. A. Graystone, S. J. Langford and T. D. M. Bell, Chem. Commun., 2013, 49, 5061–5063 RSC.
- D. Buckland, S. V. Bhosale and S. J. Langford, Tetrahedron Lett., 2011, 52, 1990–1992 CrossRef CAS.
- W. Choi, D. Harada, K. Oyaizu and H. Nishide, J. Am. Chem. Soc., 2011, 133, 19839–19843 CrossRef CAS PubMed.
- R. Gangopadhyay and A. De, Chem. Mater., 2000, 12, 608–622 CrossRef CAS.
- H. Nishide and K. Oyaizu, Science, 2008, 319, 737–738 CrossRef CAS PubMed; K. Oyaizu and H. Nishide, Adv. Mater., 2009, 21, 2339–2344 CrossRef.
- K. Oyaizu, A. Hatemata, W. Choi and H. Nishide, J. Mater. Chem., 2010, 20, 5404–5410 RSC.
- J.-M. Tarascon and M. Armand, Nature, 2001, 414, 359–367 CrossRef CAS PubMed.
- Z. Song, H. Zhan and Y. Zhou, Chem. Commun., 2009, 448–450 RSC.
- G. S. Vadehra, R. P. Maloney, M. A. Garcia-Garibay and B. Dunn, Chem. Mater., 2014, 26, 7151–7157 CrossRef CAS.
- Z. Song, H. Zhan and Y. Zhou, Angew. Chem., Int. Ed., 2010, 49, 8444–8448 CrossRef CAS PubMed.
- H. Nishide, S. Iwasa, Y.-J. Pu, T. Suga, K. Nakahara and M. Satoh, Electrochim. Acta, 2004, 50, 827–831 CrossRef CAS.
- T. Kawai, K. Oyaizu and H. Nishide, Macromolecules, 2015, 48, 2429–2434 CrossRef CAS.
- T. Suga, H. Konishi and H. Nishide, Chem. Commun., 2007, 1730–1732 RSC.
- B. A. Ikkanda, S. A. Samuel and B. L. Iverson, J. Org. Chem., 2014, 79, 2029–2037 CrossRef CAS PubMed.
- R. T. Hayes, M. R. Wasielewski and D. Gosztola, J. Am. Chem. Soc., 2000, 122, 5563–5567 CrossRef CAS.
- N. Sano, W. Tomita, S. Hara, C.-M. Min, J.-S. Lee, K. Oyaizu and H. Nishide, ACS Appl. Mater. Interfaces, 2013, 5, 1355–1361 CAS.
- K. Oyaizu, Y. Ando, H. Konishi and H. Nishide, J. Am. Chem. Soc., 2008, 130, 14459–14461 CrossRef CAS PubMed.
Footnote |
† Electronic supplementary information (ESI) available. See DOI: 10.1039/c6ra06103f |
|
This journal is © The Royal Society of Chemistry 2016 |