DOI:
10.1039/C6RA05716K
(Paper)
RSC Adv., 2016,
6, 45585-45594
Novel polyamide thin-film composite nanofiltration membrane modified with poly(amidoamine) and SiO2 gel
Received
3rd March 2016
, Accepted 21st April 2016
First published on 25th April 2016
Abstract
Recently, poly(amidoamine) (PAMAM) has emerged as a novel material due to its high density of functional groups, hyper-branched structure and hydrophilic nature. PAMAM has been used as a monomer during an interfacial polymerization process for the fabrication of nanofiltration membranes. Previous work has focused on the low generation of PAMAM (G0, G1 and G2), however, the high generation of PAMAM (G4 and G5) still lacks investigation. This work focuses on the preparation of nanofiltration membranes, which are made of PAMAM–NH2 G4 and PAMAM–NH2 G5. By optimizing the concentration of PIP and SiO2 gel in the aqueous solution, the pure water flux improved by 106% while separation properties are kept at the same level. XPS, EDS, SEM, AFM and contact angle were used to characterize the NF membrane properties. The PAMAM/PIP/SiO2 membrane prepared under the optimum conditions exhibited a pure water flux of 38.5 L m−2 h−1 and Na2SO4 rejection of 92.0% under 0.6 MPa. The PAMAM/PIP/SiO2 membrane’s robust long-time running performance showed its good potential in practical applications.
1. Introduction
Due to the serious global environmental problem, water safety has become a rising concern. To deal with this serious concern, many efforts have been made to reuse waste water.1 Nanofiltration (NF), owing to its unique nature between ultrafiltration and reverse osmosis, has generated great attention in academia and manufacturing recently. It has the following advantages: moderate MWCO (200–1000 Da), higher flux and lower operating pressure compared with reverse osmosis, high rejection of divalent ions (Mg2+, SO42−, Ca2+, Fe3+, and so on), low rejection of monovalent ions (Cl−, Na+, and so on) and low operating and maintenance costs.2–4 NF membranes have been applied in many areas:5,6 waste water treatment,7,8 separation of magnesium and lithium,9 the food industry,10 the pharmaceutical industry,11 the removal of sulfate from concentrated aqueous salt solutions,12 etc.
Interfacial polymerization is the main method of fabricating thin separation layers for NF membranes.13,14 This technique is a self-inhibiting process; the active thin film can be controlled in a range from sub-10 nm to 200 nm.15 In interfacial polymerization, monomer type will play a key role in NF membrane performance. Different monomers will endow the NF membrane with special properties. Tang et al.4 mixed a new monomer, 2,2′-bis(1-hydroxyl-1-trifluoromethyl-2,2,2-triflutoethyl)-4,4′-methylenedianiline (BHTTM), with PIP and successfully prepared a NF membrane that can tolerate active chlorine at 3000 ppm. Fang et al.16 employed a positively charged polymer, branched polyethylenimine, as a monomer, and successfully obtained a positively charged thin film membrane with a PWF of 17 LMH bar−1 and MgCl2 rejection of 96.7%. Ahmad Akbari et al.17 mixed 2,5-diaminobenzene sulfonic acid (2,5-DABSA) with piperazine, and prepared a novel sulfonated polyamide thin-film composite nanofiltration membrane which has a higher flux and better hydrophilic properties compared with a NF membrane prepared from pure piperazine.
Recently, poly(amidoamine) (PAMAM)18 has emerged as a novel material due to its high density of functional groups, hyper-branched structure and hydrophilic nature. PAMAM has been used as a monomer during the interfacial polymerization process for the fabrication of NF membranes. Jin et al.19 prepared a novel PA–SiO2 NF membrane with PAMAM (G0) as an aqueous phase solution; with the addition of nano-SiO2, the anti-fouling ability was enhanced. Lianchao et al.20 reported a novel NF membrane made via the interfacial polymerization of PAMAM (G0, G1, G2) with TMC, and this NF membrane was especially suitable for the separation of cationic solutes. Sum et al.21 employed PAMAM (G0) as a modifier in PIP aqueous solution, the NF membrane performance was improved greatly for the larger free volume of PAMAM. Mansourpanah et al.22 systematically investigated the effect of different generations and concentrations of PAMAM on NF membrane performance. However, the previous work has focused on the low generation of PAMAM (G0, G1, G2). The high generation of PAMAM (G4, G5) still lacks investigation.
In this study, we focus on the preparation of NF membranes made of PAMAM–NH2 G4 and PAMAM–NH2 G5. To improve NF membrane performance, PIP was added into an aqueous solution and the effect of PIP concentration in the aqueous solution was investigated. Moreover, to maximize membrane performance, SiO2 gel was added into the aqueous solution. XPS, EDS, SEM, AFM and water contact angle were used to characterize the NF membranes. Additionally, the preparation conditions were investigated thoroughly.
2. Experimental
2.1 Materials
A polysulfone (PSF) ultrafiltration membrane (the MWCO and PWF are 50
000 Da and 125 LMH bar−1, respectively) supplied from the Water Treatment Technology Development Center (Hangzhou, China) was used as a support for the NF membrane. TMC (≥98%) was purchased from Qingdao Benzo Chemical Company (China). Dendritic polyamidoamine (PAMAM) was provided by Weihai CY Dendrimer Technology Co., Ltd. PIP (GR) was purchased from Sigma Aldrich. Silica sol (30% w/w, 8–15 nm) was supplied by Zhejiang Yuda Chemical Industry Company. All other reagents were of analytical grade and used without further purification.
Detailed information for PAMAM is shown in Table 1. The chemical structures of PAMAM and PIP are illustrated in Fig. 1.
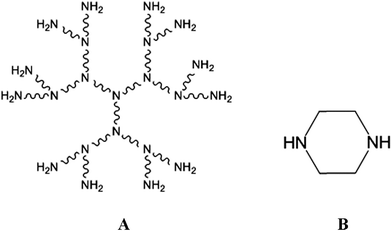 |
| Fig. 1 The chemical structures of PAMAM (G2) (A) and PIP (B). | |
2.2 Preparation of thin film composite membranes
The TFC membranes were fabricated though an interfacial polymerization process on a PSF supporting membrane with mixed amines in deionized water and TMC in hexane. First, the PSF membrane was immersed in the pure water for 5 hours to clean it up. Then, aqueous solutions of the amine monomer mixture were poured on the PSF membrane which was clamped with two Teflon chamber plates, and kept in contact for 5 minutes at room temperature. The excess solution was removed with an air knife to ensure that no droplets were on the membrane surface. Subsequently, the organic solution of TMC was poured on the PSF membrane for a pre-determined length of time (5 s, 10 s, 15 s and 30 s) to generate a thin polyamide layer on the supporting structure through interfacial polymerization. After the excess organic solution was removed, the membrane was put into an 80 °C oven for 5 minutes for further polymerization. Finally, the TFC membranes were stored in the pure water until they were tested.
2.3 Characterization of the TFC membranes
Three TFC membranes, PAMAM (0.5% w/v PAMAM G5, 0.15% w/v TMC, 15 s reaction time), PAMAM/PIP (0.4% w/v PAMAM G5 + 0.1% w/v PIP, 0.15% w/v TMC, 15 s reaction time), and PAMAM/PIP/SiO2 (0.4% w/v PAMAM G5 + 0.1% w/v PIP + 0.3% w/v SiO2, 0.15% w/v TMC, 15 s reaction time) were prepared for the characterization of the TFC membranes. All the membrane samples were rinsed with pure water several times and dried in a 40 °C oven for 24 hours.
2.3.1 XPS analysis. X-ray photoelectron spectroscopy analysis (XPS; VG Microlab II, UK) was used to determine the chemical composition of the membrane top layer.
2.3.2 EDS and SEM analysis. Energy Dispersive Spectrometry (EDS, JEOL JSM-6306LV) was used to determine the concentration of SiO2 in the membrane skin layer. The cross and top sectional morphologies of the NF membrane were observed using a scanning electron microscope (SEM, NOVA NANOSEM450) with a magnification of 50.0k×. The cross section of the NF membrane was obtained by fracturing the membrane in liquid nitrogen, and the membrane samples were sputtered with gold.
2.3.3 AFM analysis. Atomic force microscopy (Veeco, Nanoscope IIIa Multimode AFM) was employed to determine the topographic images and the roughness of the NF membrane top surface. The NF membranes were scanned with a range of 5 μm × 5 μm. The roughness of the NF membrane top surface was analyzed with NanoScope software, and the AFM figures were produced using Gwyddion software.
2.3.4 Dynamic water contact angle. To characterize the hydrophilicity of the NF membrane top surface, a contact angle meter (JC2000A, Shanghai Zhong Cheng Digital Equipment Co., Ltd, China) was utilized to measure the dynamic water contact angle at 25 °C. Each membrane sample was measured at 5 random locations to minimize experimental error.
2.4 Evaluation of the NF membrane
A cross-flow nanofiltration evaluation system described elsewhere23 was used to evaluate NF membrane performance. Briefly, the effective trans-membrane pressure was 0.6 MPa, and the ambient temperature was 25 °C. Three modules containing one plant membrane each were tested at the same time to ensure the repeatability of the experiment, and each membrane effective area was 75 cm2. The new modules were compacted with pure water under 0.6 MPa for 1 hour to reach a stable state, after that, pure water flux was measured as follows: |
 | (1) |
where J is the pure water flux (LMH), V is the volume of pure water (L), A is the effective membrane area (m2) and T is the permeate time (h).
Rejection properties were calculated using the following formula:
|
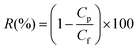 | (2) |
where
Cf is the content of the feed solution, while
Cp is the content of the permeate solution.
The content of the neutral organic solution was measured using a TOC analyzer (Shimadzu, Model TOCVPN Japan), and the content of salt solution was measured using a DDS-11A conductance meter (Shanghai Neici Instrument Company, China).
Table 1 Detailed information for PAMAM G4 and PAMAM G5
Generation |
M.W. |
Molecular formula |
Surface group |
Number of end groups |
4 |
14 215 |
C622H1248N250O124 |
–NH2 |
64 |
5 |
28 826 |
C1262H2528N506O252 |
–NH2 |
128 |
2.5 Antifouling performance test
Antifouling performance testing was carried out with a BSA solution (1 g L−1) under 0.6 MPa at 25 °C. First, the membrane module was operated with pure water for 40 minutes and the flux was recorded every 10 minutes. Then, the pure water was replaced with BSA solution and the flux was recorded every 10 minutes during 40 minutes of continuous running. Finally, the BSA solution was replaced with pure water, and the flux recorded over the following 40 minutes. The flux recovery ratio (FRR) of the NF membrane was calculated according to the following equation: |
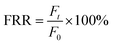 | (3) |
where F0 is the flux of the initial time and Ft is the flux of time t.
3. Results and discussion
3.1 Chemical composition
X-ray photoelectron spectroscopy analysis identifies the chemical composition of the TFC membranes in the range of 3–10 nm. Detailed information of the chemical composition is given in Fig. 2 and Table 2. The results show that change in the chemical composition of different samples is very limited, which is very similar to the results reported in ref. 21, and the observations reveal that the main composition of the three samples is PAMAM. However, no obvious presence of Si in the PAMAM/PIP/SiO2NF membrane can be seen from the XPS results. This is possibly due to the concentration of SiO2 in the polyamide layer being very low, so no Si is detected (Table 3).
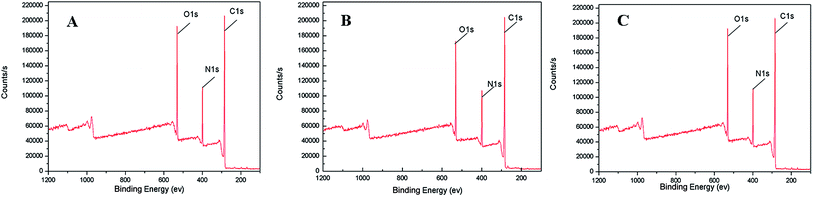 |
| Fig. 2 XPS spectra of (A) PAMAM NF membrane, (B) PAMAM/PIP NF membrane, and (C) PAMAM/PIP/SiO2 NF membrane. | |
Table 2 Elemental composition of the NF membranes analyzed using XPS
Sample |
Atom percent (%) |
Si |
C1s |
N1s |
O1s |
PAMAM |
68.22 |
15.07 |
16.71 |
0 |
PAMAM/PIP |
69.78 |
14.18 |
16.04 |
0 |
PAMAM/PIP/SiO2 |
68.00 |
14.52 |
17.48 |
0 |
Table 3 Elemental content of the PAMAM/PIP/SiO2 NF membrane top surface analyzed using EDS
Element |
wt% |
Atom% |
C |
58.63 |
65.7 |
N |
15.89 |
15.27 |
O |
19.7 |
16.57 |
Si |
0.51 |
0.24 |
S |
5.28 |
2.22 |
To prove the existence of silica in the membranes, EDS results are provided. Both of the membrane top surface and cross-section were scanned to record the EDS spectra shown in Fig. 3 and 4. The EDS spectra present a Si peak at 1.76 keV. The Si content of the top surface and the cross-section is 0.51 wt/% and 0.56 wt/%, respectively. This result proves the existence of SiO2 nanoparticles.
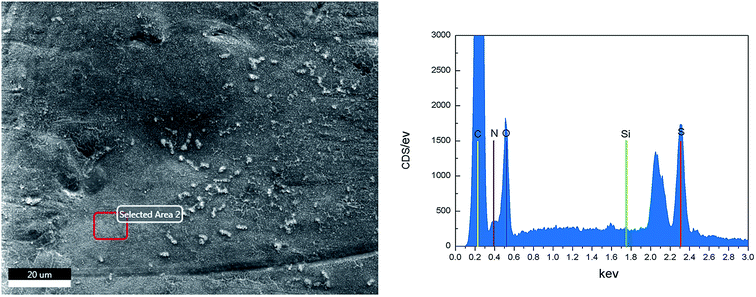 |
| Fig. 3 EDS spectrum of the PAMAM/PIP/SiO2 NF membrane top surface. | |
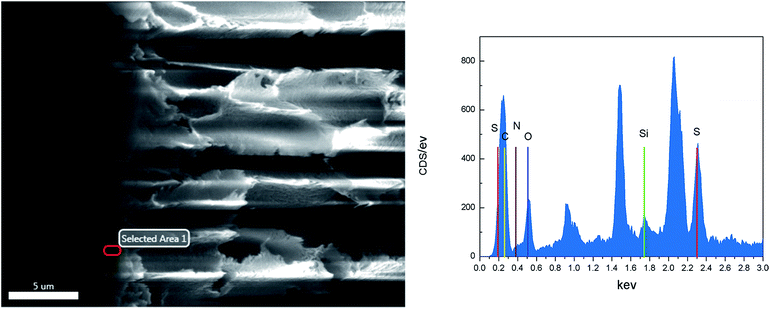 |
| Fig. 4 EDS spectrum of the PAMAM/PIP/SiO2 NF membrane cross-section. | |
3.2 Morphology
Fig. 5 shows the morphologies of the top surface and cross section of the PAMAM NF membrane, PAMAM/PIP NF membrane and PAMAM/PIP/SiO2 NF membrane. It clearly shows that the PAMAM NF membrane exhibits a nodular structure. This structure can be ascribed to the huge monomer weight of PAMAM, so the PAMAM–TMC matrix is big, similar to the 2D structural module reported in ref. 21. On the other hand, with the addition of PIP, some areas of the NF membrane surface exhibit a bigger nodular structure, while in the other areas, the nodular structure becomes smaller. The change can be ascribed to the small molecular weight of PIP, meaning that PIP can easily permeate the polyamide layer and aggregate on the membrane surface, while in the other areas, given the small size of the PIP–TMC matrix, it can fill the gap between the PAMAM–TMC matrix. With the addition of SiO2, the diffusion of PIP was changed by the negative charge of SiO2, because of the electrostatic interaction of PIP and SiO2. So the NF membrane changes a lot with the addition of SiO2, and the aggregation of PIP on the membrane surface reduces greatly (Table 4).
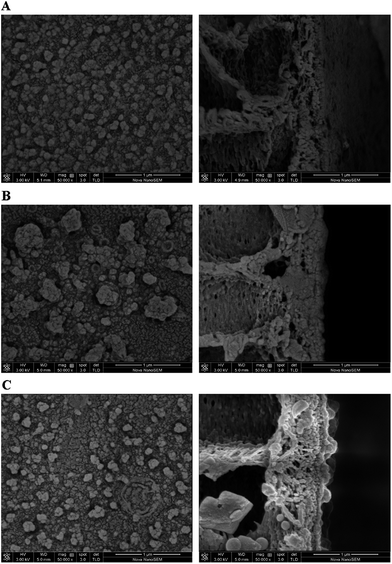 |
| Fig. 5 SEM images of the surface and cross-sectional morphology of the NF membranes. (A) PAMAM NF membrane, (B) PAMAM/PIP NF membrane, and (C) PAMAM/PIP/SiO2 NF membrane. | |
Table 4 Elemental content of the PAMAM/PIP/SiO2 NF membranes cross-section analyzed using EDS
Element |
wt% |
Atom% |
C |
64.25 |
73.93 |
N |
5.44 |
5.36 |
O |
17.58 |
15.19 |
Si |
0.56 |
0.27 |
S |
12.18 |
5.25 |
The AFM results are given in Fig. 6 and Table 5 with an area of 5 μm × 5 μm. As mentioned before, the PIP–TMC matrix will fill the gap between the PAMAM–TMC matrix, so the bumps in Fig. 5B become smaller and the roughness of the PAMAM/PIP NF membrane is less than the PAMAM NF membrane. Also, the addition of SiO2 increases NF membrane roughness, which is consistent with the work reported in ref. 24.
 |
| Fig. 6 AFM images of the NF membranes. (A) PAMAM NF membrane, (B) PAMAM/PIP NF membrane, and (C) PAMAM/PIP/SiO2 NF membrane. | |
Table 5 The roughness of the NF membranes
Membranes |
Ra (nm) |
Rms (nm) |
PAMAM |
38.3 |
47.1 |
PAMAM/PIP |
20.8 |
25.8 |
PAMAM/PIP/SiO2 |
29.8 |
39.4 |
3.3 Hydrophilicity
The dynamic water contact angle results for the NF membranes are shown in Fig. 7. It is shown that all the membranes have good hydrophilicity (below 40°), which is mainly ascribed to the high density of end functional groups of PAMAM. Obviously, with the addition of SiO2 gel, the water contact angle is reduced greatly, this is because the SiO2 gel is rich in hydroxyl groups, which are very hydrophilic.
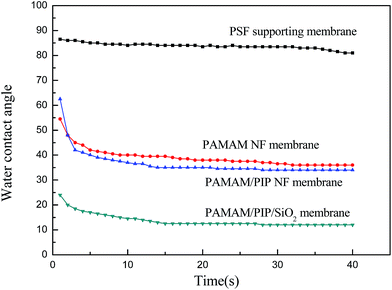 |
| Fig. 7 Dynamic water contact angles of the NF membranes. | |
3.4 Effect of preparation parameters on NF membrane performance
3.4.1 PAMAM concentration. Monomer concentration is the key influential factor for NF membrane formation, and will affect the NF membrane performance greatly. The concentration of PAMAM in aqueous solution was investigated to optimize NF membrane performance. In Fig. 8, the effect of PAMAM concentration on NF membrane PWF and salt rejection was studied under the following conditions: with a pre-determined concentration of PAMAM G5 in aqueous solution, 0.15% w/v TMC in organic solution, a reaction time of 15 s and curing conditions of 80 °C for 5 minutes. In Fig. 8, when the concentration of PAMAM increases from 0.3% w/v to 0.5% w/v, the water flux decreases a little from 18.9 LMH to18.7 LMH, while the salt rejection increases greatly from 84.4% to 92.4%. This was because at high PAMAM concentrations, the degree of polymerization of the polyamide layer is increased, so salt rejection is increased while water flux is decreased. With a further increase of PAMAM, the salt rejection stays at a stable level, and the PWF become lower. So, the optimal PAMAM concentration is 0.5% w/v.
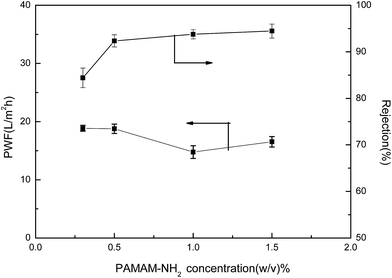 |
| Fig. 8 Effect of PAMAM concentration on NF membrane performance (0.6 MPa, 2000 ppm Na2SO4). | |
3.4.2 TMC concentration. The effect of TMC concentration on NF membrane performance is presented in Fig. 9. Similar to before, the interfacial polymerization conditions are as follows: 0.5% w/v PAMAM in aqueous solution, a certain concentration of TMC in organic solution, a reaction time of 15 s and curing conditions of 80 °C for 5 minutes. When the concentration of TMC increased from 0.05% w/v to 0.15% w/v, the salt rejection increased sharply from 58.0% to 92.4%. As mentioned before, with an increase of TMC concentration, the polymerization degree increased, so the salt rejection increased while the water flux decreased. With an increase of TMC concentration, the membrane performance becomes poor. So 0.15% w/v is the optimal concentration of TMC.
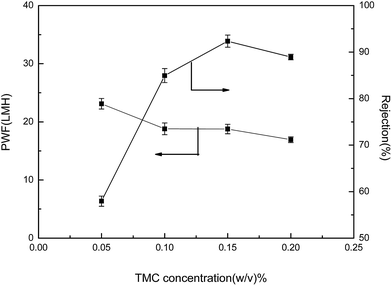 |
| Fig. 9 Effect of TMC concentration on NF membrane performance (0.6 MPa, 2000 ppm Na2SO4). | |
3.4.3 Reaction time. Reaction time also plays an important role in NF membrane fabrication. Reaction times ranging from 5 s to 30 s were investigated, and the results for the effect of reaction time are shown in Fig. 10. From the results, it is clearly seen that when the reaction time is prolonged from 5 s to 15 s, the best NF membrane with PWF of 18.7 LMH and salt rejection to 92.4% was obtained, and when the time is extended to 30 s, a decline in salt rejection was observed.
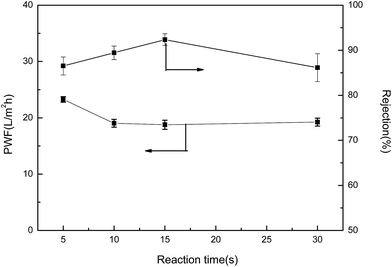 |
| Fig. 10 Effect of reaction time on NF membrane performance (0.6 MPa, 2000 ppm Na2SO4). | |
It is well known that interfacial polymerization is self-limited. With an increase of the reaction time, the thickness of the polyamide layer is improved, which will hinder the diffusion of monomers from the aqueous solution to the organic solution. So in the following experiments, 15 s was selected as the reaction time.
3.4.4 Different generation of PAMAM. For hyper-branched polymers, generation also affects NF membrane performance. In ref. 22, the effect of low generation PAMAM (G0, G1 and G2) on NF membrane performance was studied systematically. However, high generation PAMAM still lacks investigation. In this section, two high generations of PAMAM (G4 and G5) are studied. The experimental conditions are as follows: 0.5% w/v PAMAM in aqueous solution, 0.15% w/v TMC in organic solution, a reaction time of 15 s and curing conditions of 80 °C for 5 minutes. Membrane performance is shown in Table 6. For the lower molecular weight of PAMAM–NH2 G4, molecular vibration is lower when compared with G5, so the G4 NF membrane possesses the higher PWF. But the PAMAM–NH2 G5 has a more compact structure and more functional groups, so the salt rejection of PAMAM–NH2 G5 is higher than PAMAM–NH2 G4. So, PAMAM–NH2 G5 is more suitable to be an aqueous phase monomer.
Table 6 Performance of the NF membranes prepared from PAMAM–NH2 G4 and PAMAM–NH2 G5
Membrane |
PWF (LMH) |
Solute rejection (%) |
Glucose |
Sucrose |
Raffinose |
Na2SO4 |
MgSO4 |
NaCl |
PAMAM–NH2 G4 |
20.5 ± 1.1 |
89.3 ± 1.3 |
95.9 ± 0.8 |
96.4 ± 1.1 |
87.2 ± 2.1 |
89.3 ± 1.0 |
39.6 ± 0.9 |
PAMAM–NH2 G5 |
18.7 ± 0.9 |
88.6 ± 1.5 |
94.7 ± 1.2 |
96.8 ± 0.7 |
92.3 ± 1.7 |
92.6 ± 1.3 |
40.9 ± 1.1 |
3.4.5 PAMAM/PIP ratio. The total concentration of amine is kept at 0.5% w/v, and the effect of PAMAM/PIP ratio is illustrated in Fig. 11. It can be seen that when the amount of PIP is increased from 0 to 20% (w/w), the PWF improves from 18.7 LMH to 32.1 LMH, while the salt rejection is kept at the same level. According to the discussion in Section 3.2, the addition of PIP will decrease the roughness of the membrane, which will reduce the effective membrane area. To figure out the reason for the improvement in the PWF, the separation properties were studied thoroughly, and the results are listed in Table 7. The rejection for NaCl decreases a lot from 40.9% to 31.9%, and the rejection for glucose also decreases from 88.6% to 84.4%. From these results, it is speculated that with the addition of PIP, the amount of nanopores of under 0.36 nm in size increases a lot. So a relatively loose polyamide layer was obtained for the amount of PIP in the range of 5–10% w/w.
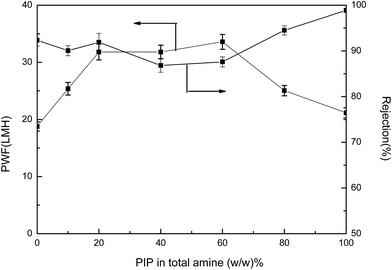 |
| Fig. 11 Effect of PAMAM/PIP mixing ratio on NF membrane performance (0.6 MPa, 2000 ppm Na2SO4). | |
Table 7 The performance of the NF membranes prepared from PAMAM and PAMAM/PIP
Membrane |
PWF (LMH) |
Solute rejection (%) |
Glucose |
Sucrose |
Raffinose |
Na2SO4 |
MgSO4 |
NaCl |
PAMAM–NH2 |
18.7 ± 0.9 |
88.6 ± 1.5 |
94.7 ± 1.2 |
96.8 ± 0.7 |
92.3 ± 1.7 |
92.6 ± 1.3 |
40.9 ± 1.1 |
PAMAM–NH2/PIP |
32.1 ± 1.4 |
84.4 ± 0.8 |
96.0 ± 0.7 |
97.0 ± 0.5 |
91.5 ± 1.1 |
92.8 ± 1.0 |
31.9 ± 1.2 |
Nevertheless, when the addition of PIP kept on increasing from 10% to 60%, the rejection decreased. This is mainly ascribed to the competing effect between PIP and PAMAM, which will reduce the polymerization degree. When the amount of PIP is over 80%, rejection increases while the PWF decreases. This is because when PIP is the major component, the NF membrane exhibits the properties of PIP, the thickness of the NF membrane increases and the rejection increases. Thus, when the amount of PIP reaches 20%, the NF membrane performance is optimized.
3.4.6 SiO2 concentration. To maximize the membrane performance, SiO2 gel is added into the aqueous solution which contains 0.1% w/v PIP + 0.4% w/v PAMAM, then reacted with 0.15% w/v TMC organic solution for 15 seconds, and finally heat treated in a 80 °C oven for 5 minutes. The results are shown in Fig. 12, when the SiO2 increases from 0% w/v to 0.1% w/v, the flux decreases and the salt rejection increases. The phenomenon can be explained as follows: the size of SiO2 is very close to that of the UF membrane pores, and SiO2 blocks some membrane pores, so the flux is reduced. When the amount of SiO2 reaches 0.3% w/v, for the increase of membrane roughness (which leads to the membrane effective contact area improving) and hydrophilicity, just as has been discussed in Section 3.2 and 3.3, so the flux improves to 38.5 L m−2 h−1 while salt rejection is still kept at a high level (92%). When the SiO2 keeps increasing, for the agglomeration of SiO2 particles, the flux decreases. So, the optimal amount of SiO2 is 0.3% w/v.
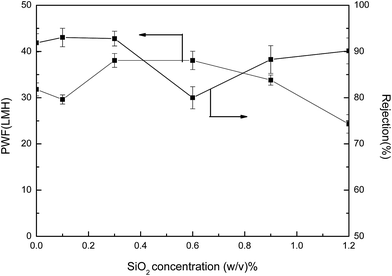 |
| Fig. 12 Effect of SiO2 concentration on NF membrane performance (0.6 MPa, 2000 ppm Na2SO4). | |
3.5 Rejection properties of the PAMAM–NH2/PIP/SiO2 NF membrane
Various solutions (glucose, sucrose, raffinose, Na2SO4, MgSO4, NaCl) were used to identify the optimal NF membrane. The optimized NF membrane was prepared under the following conditions: 0.1% w/v PIP + 0.4% w/v PAMAM + 0.3% w/v SiO2 in aqueous solution, 0.15% TMC in organic solution, a reaction time of 15 seconds, and heat treatment conditions of 80 °C for 5 minutes.
The results are shown in Fig. 13, it is clearly shown that salt rejection is in the order of Na2SO4 > MgSO4 > NaCl, which indicates that the novel NF membrane is negatively charged. From the rejection results to neutral solution, the MWCO of the NF is between 200 and 300.
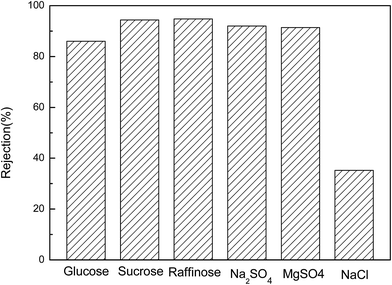 |
| Fig. 13 The separation properties of the PAMAM–NH2/PIP/SiO2 NF membrane. | |
Furthermore, Table 8 compares the PAMAM–NH2/PIP/SiO2 NF membrane with some other PAMAM NF membranes which have been reported in the literature. It shows that the PAMAM–NH2/PIP/SiO2 NF membrane has a relatively good performance.
Table 8 List of comparisons of the NF membrane performance that was prepared from PAMAM
Monomers and additives |
PWF (LMH bar−1) |
MWCO (Da) |
Salt rejection (%) |
Salt concentration (ppm) |
Operation pressure (bar) |
Reference |
Na2SO4 |
MgSO4 |
NaCl |
PAMAM(G0) + PIP + TEA |
9.24 |
— |
— |
99.0 |
— |
1000 |
7 |
21 |
PAMAM(G0, G1, G2) + PIP + TEA |
3 |
140–170 |
— |
— |
80 |
1000 |
7 |
22 |
PAMAM(G0, G1, G2) |
6.25 |
300–350 |
38.2 |
67.6 |
36.2 |
1000 |
6 |
20 |
PAMAM(G0, G1) |
1.59 |
>600 |
88.6 |
86.4 |
48.1 |
500 |
8 |
25 |
PAMAM(G0) + SiO2 |
2.41 |
— |
78 |
— |
— |
— |
6 |
26 |
PAMAM(G4, G5) + PIP + SiO2 gel |
6.41 |
200–300 |
92 |
91.4 |
35.2 |
2000 |
6 |
Current work |
3.6 Stability of the PAMAM–NH2/PIP/SiO2 NF membrane in the long-time running
The stability of the NF membrane in long term testing plays an important role in practical applications. Fig. 14 shows the salt rejection and flux change during 4 days testing. The results show that the NF membrane possesses robust stability. The PAMAM–NH2/PIP/SiO2 NF membrane possess Na2SO4 rejection of about 92% and a permeate flux of about 34 LMH.
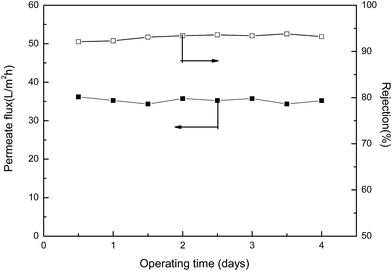 |
| Fig. 14 Long term testing of the PAMAM/PIP/SiO2 NF membranes (0.6 MPa, 2000 ppm Na2SO4). | |
3.7 Antifouling properties of the NF membranes over long term running
NF membrane antifouling properties were tested using BSA solution at 0.6 MPa at 25 °C. Fig. 15 shows that all of the three NF membranes had good antifouling performance. The differences in their flux recovery ratios are very small. This is because the compositions of their surfaces are almost the same as the XPS results show. This means that the compositions of all NF membrane surfaces are PAMAM, as PAMAM has the highest density of functional groups, a hyper-branched structure and a hydrophilic nature. Its rich –NH–CO– groups will form hydrogen bonds with water, which reduces BSA absorption on the membrane surface. All of the NF membranes exhibited excellent antifouling properties which are attributed to PAMAM.
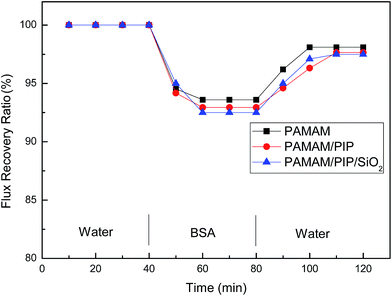 |
| Fig. 15 Time dependent flux recovery ratio of the NF membranes in antifouling evaluation (0.6 MPa, 25 °C). | |
4. Conclusions
A novel PAMAM/PIP/SiO2 NF membrane was successfully prepared though interfacial polymerization. The NF membrane was characterized using XPS, EDS, SEM, AFM and contact angles. The PAMAM/PIP/SiO2 NF membrane almost doubled its flux when compared with the PAMAM membrane, the PWF improved from 18.7 LMH to 38.5 LMH while the salt rejection stayed at the same level. The salt rejection is in the order of Na2SO4 > MgSO4 > NaCl, which indicates that the novel NF membrane is negatively charged. Antifouling testing proves that the NF membranes have good antifouling properties. The optimum preparation conditions are 0.1% w/v PIP + 0.4% w/v PAMAM + 0.3% w/v SiO2 in aqueous solution, 0.15% TMC in organic solution, a reaction time of 15 seconds, and heat treatment conditions of 80 °C for 5 minutes.
Acknowledgements
The authors are thankful for the financial support received from 2013 Special Project of the Development and Industrialization of New Materials of National Development and Reform Commission in China (GX1301), the National Science and Technology Support Project of China (2014BAB07B01 and 2015BAB09B01), project of National Energy Administration of China (2011-1635 and 2013-117) and the Key Technology R&D Program of Jiangsu Committee of Science and Technology in China (BE2013031).
References
- M. Shannon, P. Bohn, M. Elimelech, J. Georgiadis, B. Mariñas and A. Mayes, Nature, 2008, 452, 301–310 CrossRef CAS PubMed.
- D. Hu, Z.-L. Xu, Y.-M. Wei and Y.-F. Liu, Desalination, 2014, 336, 179–186 CrossRef CAS.
- D. Hu, Z.-L. Xu and C. Chen, Desalination, 2012, 301, 75–81 CrossRef CAS.
- Y.-J. Tang, Z.-L. Xu, S.-M. Xue, Y.-M. Wei and H. Yang, J. Membr. Sci., 2016, 498, 374–384 CrossRef CAS.
- A. W. Mohammad, Y. H. Teow, W. L. Ang, Y. T. Chung, D. L. Oatley-Radcliffe and N. Hilal, Desalination, 2015, 356, 226–254 CrossRef CAS.
- D. Zhou, L. Zhu, Y. Fu, M. Zhu and L. Xue, Desalination, 2015, 376, 109–116 CrossRef CAS.
- X. Wei, X. Kong, S. Wang, H. Xiang, J. Wang and J. Chen, Ind. Eng. Chem. Res., 2013, 52, 17583–17590 CrossRef CAS.
- B. A. M. Al-Rashdi, D. J. Johnson and N. Hilal, Desalination, 2013, 315, 2–17 CrossRef CAS.
- S.-Y. Sun, L.-J. Cai, X.-Y. Nie, X. Song and J.-G. Yu, Journal of Water Process Engineering, 2015, 7, 210–217 CrossRef.
- I. Cortés-Giraldo, C. Megías, M. Alaiz, J. Girón-Calle and J. Vioque, Food Chem., 2016, 192, 114–118 CrossRef PubMed.
- S. T. Morthensen, J. Luo, A. S. Meyer, H. Jørgensen and M. Pinelo, J. Membr. Sci., 2015, 492, 107–115 CrossRef CAS.
- L. Meihong, Y. Sanchuan, Z. Yong and G. Congjie, J. Membr. Sci., 2008, 310, 289–295 CrossRef.
- J.-Q. Liu, Z.-L. Xu, X.-H. Li, Y. Zhang, Y. Zhou, Z.-X. Wang and X.-J. Wang, Sep. Purif. Technol., 2007, 58, 53–60 CrossRef CAS.
- W. Fang, L. Shi and R. Wang, J. Membr. Sci., 2014, 468, 52–61 CrossRef CAS.
- S. Karan, Z. Jiang and A. Livingston, Science, 2015, 348, 1347–1351 CrossRef CAS PubMed.
- W. Fang, L. Shi and R. Wang, J. Membr. Sci., 2013, 430, 129–139 CrossRef CAS.
- A. Akbari, E. Aliyarizadeh, S. M. Mojallali Rostami and M. Homayoonfal, Desalination, 2016, 377, 11–22 CrossRef CAS.
- H. Wang, C. Wu, Z. Wei, C. Li and Q. Liu, RSC Adv., 2016, 6, 4673–4682 RSC.
- L. M. Jin, S. L. Yu, W. X. Shi, X. S. Yi, N. Sun, Y. L. Ge and C. Ma, Polymer, 2012, 53, 5295–5303 CrossRef CAS.
- L. Lianchao, W. Baoguo, T. Huimin, C. Tianlu and X. Jiping, J. Membr. Sci., 2006, 269, 84–93 CrossRef.
- J. Y. Sum, A. L. Ahmad and B. S. Ooi, J. Membr. Sci., 2014, 466, 183–191 CrossRef CAS.
- Y. Mansourpanah and Z. Jafari, React. Funct. Polym., 2015, 93, 178–189 CrossRef CAS.
- G.-E. Chen, Y.-J. Liu, Z.-L. Xu, Y.-J. Tang, H.-H. Huang and L. Sun, RSC Adv., 2015, 5, 40742–40752 RSC.
- D. Hu, Z.-L. Xu and Y.-M. Wei, Sep. Purif. Technol., 2013, 110, 31–38 CrossRef CAS.
- X. X. Xu, C. L. Zhou, B. R. Zeng, H. P. Xia, W. G. Lan and X. M. He, Sep. Purif. Technol., 2012, 96, 229–236 CrossRef CAS.
- L. Jin, W. Shi, S. Yu, X. Yi, N. Sun, C. Ma and Y. Liu, Desalination, 2012, 298, 34–41 CrossRef CAS.
|
This journal is © The Royal Society of Chemistry 2016 |