DOI:
10.1039/C6RA05185E
(Paper)
RSC Adv., 2016,
6, 44224-44231
Impaction of precursor gas-induced catalyst change on morphology, growth kinetics and field emission property of carbon nanofibers
Received
27th February 2016
, Accepted 28th April 2016
First published on 29th April 2016
Abstract
Three types of carbon nanofiber (CNF): filamentous CNF, chain-like CNF and thick CNF were successfully synthesized from three hydrocarbon precursor gases of ethylene (C2H4), n-hexane (n-C6H14) and n-dodecane (n-C12H26) on iron catalyst at 1073 K. A detailed study implied that the nucleation of iron catalyst in the incubation period varied with the type of the precursor gas, which intensively affected the morphology, structure and growth kinetics of the CNFs. A thermodynamic basis of carbon diffusion in iron particle for the catalyst activity was derived. Various characterizations demonstrated that the typical filamentous CNFs (∼50 nm), developed from C2H4 with relatively large aspect ratios and highly ordered graphene layers, possessed the highest field emission enhancement factor (β). By contrast, the chain-like CNFs (∼80 nm), developed from n-C6H14 with non-sequence graphene layers, and the thick CNFs (∼500 nm), developed from n-C12H26 with numerous micropores and mesopores in the disordered graphene layers, possessed the lower values of β.
Introduction
Carbon nanofibers (CNFs) with specific electrical, mechanical and chemical properties1,2 have been applied in diverse areas, including electronics,3,4 catalyst supports,5 energy storage,6 composite materials7 and medical industry.8 Since all of these applications depend on the CNF properties (to a varying degree), controlling their size, morphology and structure during the synthesis process is extremely important.9,10 Similarly, various synthesis methods have been developed currently, including the plasma-enhanced chemical vapor deposition (PECVD)11,12 and the catalytic chemical vapor deposition (CCVD) methods.13 Catalytic chemical vapor deposition (CCVD) is a general method to produce the CNFs for its low cost and simple operation.
Researchers recently reported numerous CNFs with novel morphology and structure prepared by CCVD, and categorized them into several types based on the stacked orientation of the graphene layers. Herring-bone or fishbone-like CNFs with graphene layers angled to the fiber axis; tubular CNFs with graphene layers paralleling to the fiber axis, which is also defined as multi-walled carbon nanotubes (MCNTs); platelet CNFs with graphene layers being perpendicular to the fiber axis; spine-like nanofibers with graphene layers periodically growing, and the helical-type carbon nanotubes or nanofibers with twisted graphene layers.14–17 It is known that different types of carbon nanofibers can be deposited via control of the synthesis parameters such as reaction temperature, the types of catalyst and precursor gas.18–20 Thus a rational approach to prospective control requires a thorough understanding of the CNF formation mechanism by CCVD. The influences of temperature and catalyst on the CNF formation have been studied for decades,18,21 while researches on the impaction of precursor gas, especially the heavy hydrocarbons, on the CNF deposition were rarely reported. Since a comprehensive and systematical study is still deficient, a prospective design and synthesis of CNFs in industrial manufacture is hardly achieved.
In the present study, three groups of thermal decomposition experiments were conducted to investigate the impaction of hydrocarbon precursor gas on the formation of CNFs. Ethylene (C2H4) and two other precursor gases n-hexane (n-C6H14) and n-dodecane (n-C12H26) were selected based on our previous research.22 Three types of carbon nanofiber (filamentous CNF, chain-like CNF and thick CNF) were obtained by varying the precursor gas phase at 1073 K on iron film. It was found that the morphology, structure and grow kinetics of the CNFs dramatically depended on the size and evolution of the iron catalyst nucleated in the corresponding gas phase, which results in various field emission performances of the as-prepared CNFs, and also gives a further reveal of the CNF formation by CCVD. A thermodynamic basis of carbon diffusion in iron particle for the catalyst activity is derived. In addition, samples of the CNFs deposited at different stages were saved for the further characterization by various techniques to study their morphology and structure.
Experimental section
Synthesis experiment
The CNFs were synthesized on samples 1, 2 and 3 made of 20 μm thick iron films in a vertically placed quartz tube furnace. The feeding unit consists of a constant-flux pump, an evaporator and a mixer. The heating unit consists of five resistance elements uniformly arranged to maintain the reactor at the desired temperature. The diluent gas used in the present experiment was argon. Samples laid in an alumina crucible were hanged by nickel–chromium wires and connected to an electrobalance, placed at the middle of the quartz tube facing to the flow of the mixture gas of hydrocarbon/Ar.
To ensure the same carbon equivalent of the given precursors, sample 1 was deposited in C2H4/Ar (24%), sample 2 was deposited in n-C6H14/Ar (8%), and sample 3 was deposited in n-C12H26/Ar (4%) at 1073 K with a flow rate of 100 mL min−1. Samples were heated in flowing argon up to the specified temperature 1073 K at a ramp of 5 K min−1, and then replaced argon with the reaction mixture gas. Weight gains of samples were recorded by the electrobalance with a precision of 0.01 mg during the deposition process. Samples of CNFs deposited to different stages were saved for the further characterization. The pyrolysis products of the hydrocarbon precursors were collected at the outlet of the quartz tube and analyzed by gas chromatography.
Characterization
The morphology and structure of the CNFs deposited on the iron films were observed by a JSM-7500F emission scanning electron microscope (NI-SEM) with 25 kV accelerating voltage, and transmission electron microscope (TEM) was used to study the microscopic mechanisms involved in the catalytic growth of CNFs. High-resolution electron microscopy (HREM) images were used to study the structure of the CNFs and iron catalyst particle. TEM examination also provided the information on the size of the obtained catalysts. The random sampling investigation of the three samples was conducted in per unit area of 1 μ−2, 1 μ−2 and 100 μ−2, respectively. Raman spectrum analysis was carried out at room temperature using a Kaiser RXN dispersive Raman spectrometer equipped with a 633 nm (60 mW) laser for excitation. Nitrogen adsorption isotherm was conducted using an automatic adsorption system (ASAP 2020) at 77 K. Field emission measurements were performed on the as-prepared CNF carpet in 2 × 10−7 Torr in a diode type configuration. The distance between the CNF tips and the anode was 200 μm. The emission current was monitored with an electrometer (Keithley 2000). All the measurements were conducted at room temperature.
Results
The growth kinetics of the CNFs
Fig. 1 illustrates the weight gains of the three types of the CNFs versus time and the final morphologies in low-magnification (red, green and blue insets). It can be seen that a complete growth process of CNFs by CCVD generally goes through four stages as reported in literatures,23,24 which are defined as the incubation period, the rapid growth stage, the steady growth stage and the deactivation stage. It can be seen that all the three types of CNFs firstly undergo an incubation period with a hardly gained weight. After that, CNFs step into a rapid growth stage with an increasing rate. As the CNF nucleation terminates, massive CNFs get into the steady growth stage with a constant growth rate. As the growth process proceeds, catalyst in the deposition system gradually loses its activity leading to the cessation of the CNF growth. The growth kinetics curve of sample 1 (deposited from C2H4, red line) shows a higher yield of CNFs than sample 2 (deposited from n-C6H14, green line) and sample 3 (deposited from n-C12H26, blue line). It is noted that the CNFs obtained from heavy hydrocarbon (n-C12H26) lasted a much sluggish and longer growth period compared to those obtained from lighter hydrocarbons (C2H4 and n-C6H14).
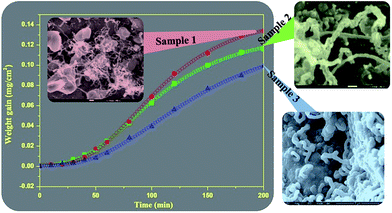 |
| Fig. 1 Weight gains of the three types of CNFs versus time, and the surface morphologies in low-magnification: sample 1 deposited from C2H4 (red), sample 2 deposited from n-C6H14 (green) and sample 3 deposited from n-C12H26 (blue). | |
Morphology and structure characterizations of the CNFs
The morphology evolution of the as-prepared CNFs is enlarged by NI-SEM in Fig. 2. Sample 1 possessed the filamentous carbon nanofibers with an average of 50 nm diameter. Firstly, the disc-shaped graphite was developed in the incubation period (Fig. 2a), and then the “tentacle” filaments grew out of the “octopus body” (Fig. 2b). The final morphology of octopus-like CNFs (Fig. 2c) reported by Jeong25 was obtained at 150 minutes. Sample 2 possessed in most case the chain-like CNFs (∼80 nm) precipitated from extruded iron particles (Fig. 2d), grew combinedly (Fig. 2e) and twisted in clusters ultimately (Fig. 2f). Sample 3 presented the thick CNFs (400–600 nm) with branches in some cases. Larger iron particles first nucleated near the cracked grain boundary (Fig. 2g). As the precipitation proceeded, the “branches” germinated from the “trunk” CNF (Fig. 2h), and blossomed in different directions (Fig. 2i).
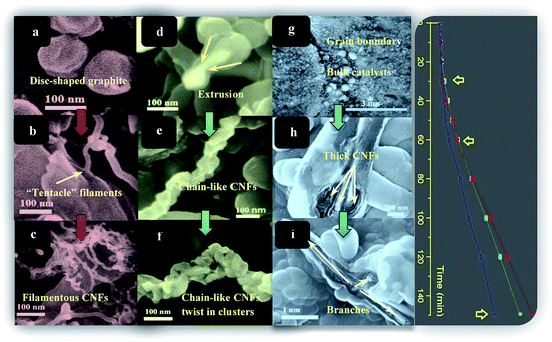 |
| Fig. 2 SEM images of the morphology evolution of the three obtained CNFs at various growth stage: filamentous CNFs at (a) 30 min, (b) 60 min and (c) 150 min; chain-like CNFs at (d) 30 min, (e) 60 min and (f) 150 min; thick CNFs at (g) 30 min, (h) 60 min and (i) 150 min. | |
Further structure characterization by HR-TEM of the as-prepared CNFs was conducted. Fig. 3a presents that the edge of iron catalyst for the filamentous CNFs perfectly paralleled to the graphene layers. The filamentous CNFs exhibited a high degree of graphitization, close to that of graphite, with the interlayer distance d002 ∼ 0.337 nm. The high degree of graphitization was also seen as the more intense arc fragments in the FFT pattern inset. Fig. 3b shows that the catalyst for the chain-like CNFs had a curvature surface which may lead the graphene planes stacked with slight mesopores. Fig. 3c illustrates that the catalyst for thick CNFs with irregular shape had a very rough surface where the graphene stacked in disordered, and that may result in numbers of micropores and mesopores. It also can be seen that the changes of the FFT patterns corresponding to the three iron catalysts indicate the phase change has occurred, probably due to the variations of temperature and carbon concentration in the catalyst particles.26
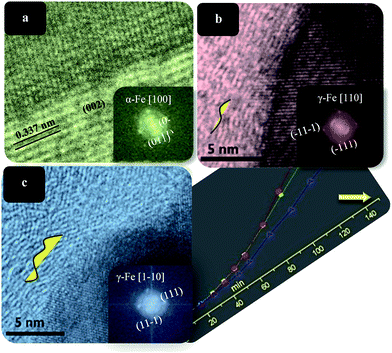 |
| Fig. 3 HR-TEM images of the CNFs obtained at 150 min and the corresponding inserted FFT patterns obtained by tilting them to low index zone axes: (a) [110] of α-Fe, filamentous CNFs; (b) [100] of γ-Fe, chain-like CNFs; (c) [1−10] of γ-Fe, thick CNFs. | |
Fig. 4 illustrates the Raman spectra of the three CNFs. It is can be seen that all the three CNFs showed two characteristic peaks which lie at about 1337 and 1590 cm−1 corresponded to the D band and G band of the graphite, respectively. It is known that the intensity ratio of the D and G bands (IG/ID) is a qualitative description of the crystallinity.24 The IG/ID ratios of the filamentous CNFs, chain-like CNFs, and thick CNFs are about 1, 0.85, and 0.6, respectively. These results indicate that the filamentous CNFs and chain-like CNFs possessing higher crystallinity than did the large thick CNFs. The results of Raman spectra agree with the HR-TEM observations.
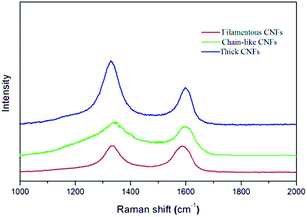 |
| Fig. 4 Raman spectra of filamentous CNFs (red curve), chain-like CNFs (green curve) and thick CNFs (blue curve). | |
The porous structure of the as-prepared CNFs was investigated by nitrogen adsorption–desorption measurements at 77 K. Fig. 5 shows that the filamentous CNFs represent the type-II adsorption–desorption isotherm which can be considered as mainly a non-mesoporous structure. While in terms of chain-like CNFs and thick CNFs, moderate N2 adsorbed at P/P0 < 0.1 and the continuous increase of N2 adsorption along with the enlarged hysteresis loops over the region of 0.1 < P/P0 < 0.9, especially for the thick CNFs, suggesting the presence of both micropores and mesopores in CNF.27 As a result, the filamentous CNFs shows a smaller BET surface area (163 m2 g−1) compared to those of chain-like CNFs (395 m2 g−1) and thick CNFs (421 m2 g−1), and the pore characteristics gives that the porosity of the filamentous CNFs (0.17 cm3 g−1) is also significantly lower than those of chain-like CNFs (0.26 cm3 g−1) and thick CNFs (0.41 cm3 g−1).
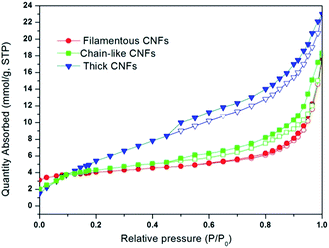 |
| Fig. 5 N2 adsorption–desorption isotherms of (1) filamentous CNFs, (2) chain-like CNFs and (3) thick CNFs. | |
Catalyst particle evolution
Catalyst particle generally plays a very leading role in the manipulation of CNFs with various morphologies and structures during the deposition process. Firstly, let us classify two types of metal catalyst in nano-scale. One is the supported nano-catalyst with diameter below 100 nm, which is mainly nucleated from the decomposition of metal carbides28 possess high catalytic activity to develop CNFs directly on supporter; the other one is defined as the bulk-catalyst, holding a diameter hundreds nanometers, which usually is developed by the cracking of the stress-concentrated areas near the grain boundaries saturated with carbon.25 Since the bulk catalyst possesses relatively low catalytic activity, it needs to nucleate or transform into nano-catalysts to develop CNFs.
Fig. 6 shows the TEM images of iron catalyst particles of the three obtained samples deposited for 30 minutes and 150 minutes, respectively. A bulk-catalyst of sample 1 first precipitated a layer of disc-shaped graphite in the nucleation period (Fig. 6a). The nano-catalysts nucleated from the bulk-catalyst developed “tentacle” filaments at the steady growth stage (Fig. 6b). Fig. 6c shows that the bulk-catalyst of sample 2 was extruded by the participated carbon in the initial stage, and then the chain-like CNFs were ultimately formed with an extruded nano-catalyst in each “chamber” (Fig. 6d). Fig. 6e shows a large size bulk-catalyst of sample 3 with irregular shape in the initial stage. Several nano-catalysts broken off from the bulk-catalyst (shown in the inset of Fig. 6e) were reshaped roundedly and trapped in the channel of the CNF (Fig. 6f). Since all the three types of CNFs grew via catalyst particle in the tip, the diameters of CNFs should intensively depend on the size of the corresponding nano-catalysts. The statistical results conducted by TEM shows that the average size of nano-catalysts of sample 3 (338 nm) is much larger than that of sample 1 (39 nm) and sample 2 (51 nm), and that allows sample 3 developing larger size CNFs as well.
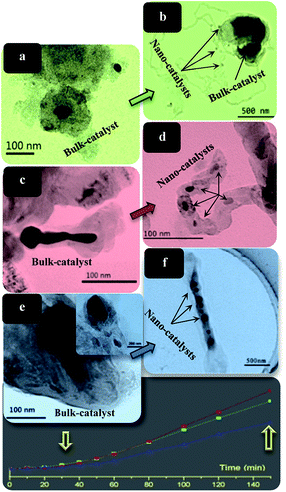 |
| Fig. 6 TEM images of the corresponding catalyst particles obtained at 30 min and 150 min, respectively: (a) bulk-catalyst, (b) nano-catalyst from filamentous CNFs; (c) bulk-catalyst, (d) nano-catalyst from chain-like CNFs; (e) bulk-catalyst, (f) nano-catalyst from thick CNFs. | |
Discussion
Impaction of hydrocarbon precursor cracking on the nucleation of iron catalyst
It is known that large hydrocarbon molecules firstly need to decompose into lighter ones before deposit CNFs through catalyst, and this reaction process becomes the rate-determining step in the incubation period since no precipitation of CNFs occurs. Most of the catalyst particles prepared at this time would be greatly affected during the precursor gas pyrolysis.
Fig. 7 shows the schematic diagram of the corresponding iron catalyst particles nucleated from C2H4, n-C6H14 and n-C12H26 precursor gases. In C2H4 gas phase (Fig. 7a), the filamentous CNFs grown out of bulk graphite possess the smallest iron nano-catalysts (∼40 nm), which is mainly nucleated via the decomposition of metal carbides.18,21 In n-C6H14 precursor gas (Fig. 7b), the iron particles saturated with carbon may have melted for the added heat released from more cracking of C–C bonds in n-C6H14. Thus, the large particle extruded into several nano-catalysts (∼50 nm) by surface tension and capillary forces from the precipitation of CNF develops the chain-like CNFs. However, in the precursor gas of n-C12H26 (Fig. 7c), the nucleation of iron catalyst particles becomes more multiple since the cracking of heavy hydrocarbon is a very complex process. We start with one possible reaction pathway of n-C12H26 cracking shown below:
|
C12H26 → 2C2H4 + C8H16 + H2 primary reaction
| (1) |
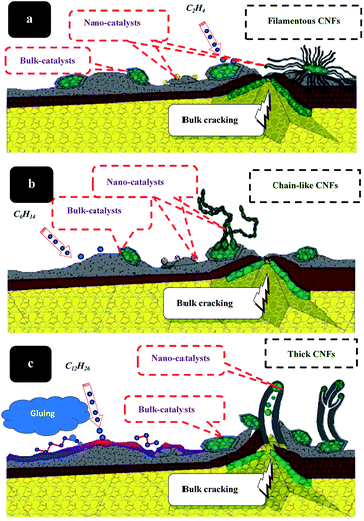 |
| Fig. 7 Schematic profile to describe the nano-catalyst and bulk-catalyst developed from the gas phase of (a) ethylene C2H4, (b) n-hexane n-C6H14 and (c) n-dodecane n-C12H26. | |
Larger molecular alkenes (e.g. C8H16) produced by the primary reaction proceed the secondary reaction eqn (2) and dehydrocyclize to arenes or aromatic compounds eqn (3) rather than cracking into small molecules, and degenerate into poly-aromatics and cokes in the end.29,30
|
C8H16 → C4H6 + C4H8 + H2 secondary reaction
| (2) |
|
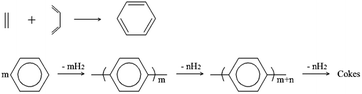 | (3) |
Poly-aromatics and cokes may stick to the metal surface like “glue”31,32 and block up the contact with absorbed C atoms. Thus the homogeneous nucleation of nano-catalysts is restrained, and the catalyst particles can only be generated from bulk fragmentation of the surface metal saturated with carbon near the grain boundaries, possessing sizes nearly ten times larger than that of filamentous CNFs, and that allows sample 3 developed thick CNFs with large diameters as well. Large bulk-catalyst saturated with carbon can easily be brittle and broken into fragments during the CNF growth, and these fragment particles remaining activities produced branch CNFs shown in Fig. 7c. We also notice that CNFs deposited from precursors smaller than n-C6H14 have little difference on the size, which may due to the diolefins and cycloolefins formation of these small alkanes are much less than the larger ones.
Effect of catalyst particle size on the growth of CNFs
The results in our study show that the size of catalyst particle not only governs the size of the CNF, but also determines the growth kinetics due to the various activities of catalyst particle. In the latter case, the activity of catalyst particles with different sizes was discussed below based on the diffusion of carbon in iron particle. The deposition process of CNFs by CCVD can be interpreted to the following course: C atoms cracking from precursor gas dissolve into the surface of catalyst particle, and then diffuse from the front to the rear, ultimately, as the end of the particle is saturated with carbon, the graphene layers precipitate there. Fig. 8 shows several possible situations based on the description of Snoeck,33 depending on the value of the carbon diffusivity in iron particle DC,Fe.
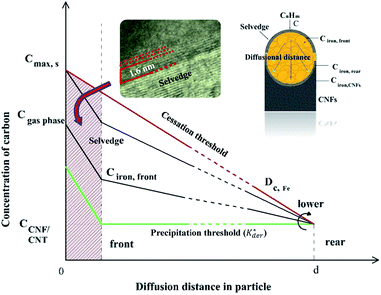 |
| Fig. 8 Schematic representation of the concentration profile of carbon dissolved in iron particle during the CNFs growth, depending on the value of the carbon diffusivity in iron particle DC,Fe. | |
At the front of the catalyst particle, a “selvedge” or “surface carbide” proposed by Alstrup34 with high concentration of carbon is created, and now was observed in the present study (TEM inset in Fig. 8) where the carbon concentration decreases from the surface concentration to the particle bulk concentration over a number of atomic layers35 (∼1.6 nm). Absorbed C atom cracked from precursor gas, dissolves in the iron catalyst particles, and diffuses down a certain concentration gradient in the particle. When the concentration of carbon and its chemical potential are uniform over the whole particle and equal to the solubility of CNF in iron (cCFe,f = cCFe,r = cCFe,CNF, μCgasphase = μCfront = μCrear = μCCNF, where cCi and μCi are the concentration and chemical potential of C in i, respectively) namely the “precipitation threshold” of CNF is satisfied (green line in Fig. 8). In this case, the gas phase (e.g. C2H4) is in equilibrium with the surface carbon resulting in:
|
C2H4 → 2Cs + 2H2 ⇌ 2CCNF + 2H2
| (4) |
where C
s and C
CNF are the surface carbon and CNF carbon, respectively. The “precipitation threshold” constant, or the equilibrium constant at CNF “precipitation threshold” should be an only temperature-determined constant
K*T, and equals to:
|
 | (5) |
where
Pi and
μ0i are the partial pressure and standard chemical potential of component i, and
rCNF is the rate of CNF formation.
At the “precipitation threshold” of CNF, DC,Fe can be very high, and the concentration of carbon in particle is almost uniform. Since the deposition of carbon is an endothermic reaction,36,37 the temperature at the rear face of the particle decreases, which results in the decline of DC,Fe as well. As the rate of CNF formation rCNF increases, the excess carbon from decomposition of precursor gas will be deposited at the exposed face of the particle, then the fraction of free particle surface available for adsorption and decomposition of hydrocarbon slowly decreases. Finally, the concentration of carbon in particle surface Cs reaches the maximum Cmax,s. In this case, the particle is completely encapsulated by thick carbon layer with losing its catalytic activity, and the growth of CNF ceases (red line in Fig. 8).
Fig. 9 schematically illustrates the growth “cessation threshold” of the three types of CNFs with totally different deposition kinetics and catalyst particles ranging from 50–500 nm. It is known that small catalyst particle nucleated from light precursors (C2H4 or CO et al.) possess a higher catalytic activity and surface energy to reshape the interface between the graphene and the precipitation plane, which leads to a high crystallinity and growth rate of CNF. The higher surface carbon concentration cmax,h of catalyst particles of chain-like CNFs may attribute to the additional heat released from n-C6H14 pyrolysis. Whereas this case is unlikely to happen during the decomposition process of n-C12H26 due to its low level cracking of C–C bonds.36 Long average diffusion path in the large catalyst particle of thick CNF leads to a relatively slow and long growth period at a certain temperature-determined DC,Fe.
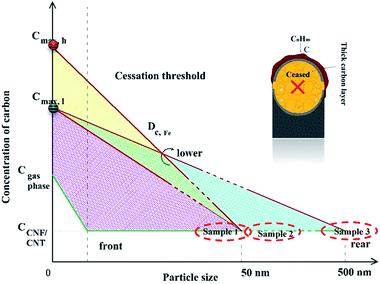 |
| Fig. 9 Schematic concentration profile of carbon dissolved in catalyst particle of the three samples corresponding to the “Cessation threshold” value of DC,Fe. | |
Field emission characteristics of the as-prepared CNFs
Carbon nanofibers with various morphology and structure surely possess diverse electrochemical properties. Fig. 10 presents the current density–electric field (J–E) characteristics curves and Fowler–Nordheim (F–N) plots of the three types of CNFs. The turn-on field were defined when I = 10 μA cm−2. Fig. 10a indicates that the turn on fields of the filamentous CNFs, chain-like CNFs, and thick CNFs are 3.6, 3.8 and 5 V μm−1, respectively. It is known that the emission current depends on the geometrical shape of the emitters. As can been seen, the turn-on field of thick CNFs is considerably high than the filamentous CNFs and chain-like CNFs, which attributes to the extremely low aspect ratio and the round tip of the thick CNFs. The roughness surface and disordered graphene multi-layers in the thick CNFs also affected the field emission performance. It is noticeable that the turn-on field does not vary much between the filamentous CNFs and chain-like CNFs, whereas, the filamentous CNFs had a higher maximum current density (9 mA cm−2 at 6.5 V μm−1) than that of the chain-like CNFs (7.2 mA cm−2 at 7 V μm−1). This can be explained by the different alignments of graphene layers with respect to the fiber axis.38 Filamentous CNFs possessing a high aspect ratio and small tip radius of curvature have been regarded as effective field emitters.39 However, the graphene layers of chain-like CNFs are not all parallel to the fiber axis for which could be curved at the junction, suggesting that the electrons cannot easily pass through the graphene layers to the emission sites. Thus, we obtained a relatively smaller emission current density from the chain-like CNFs than the filamentous CNFs.
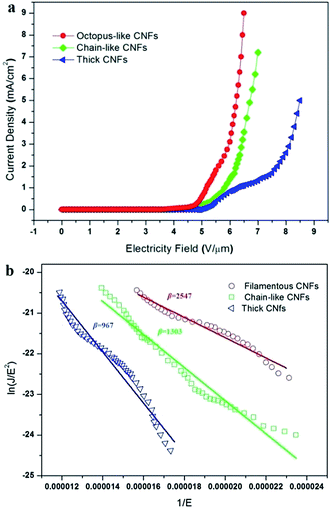 |
| Fig. 10 Field emission characteristic of the three types of CNFs: (a) J–E curves and (b) F–N plots. | |
The field emission enhancement factor (β) is generally used to characterize the field emitter efficiency, and can be calculated by the Fowler–Nordheim (F–N) equation,40,41
where
J,
E,
β, and
φ are emission current density, applied electric field, field enhancement factor, and work function,
40 respectively. The field-enhancement factor
β of the F–N equation can be calculated from the slope of the ln(
J/
E2)
vs. (1/
E) plot (
Fig. 10b) corresponding to the three as-prepared samples. The filamentous CNFs have the highest
β value of 2547, which is higher than that of the reported CNFs prepared at lower temperature.
39 Although the chain-like CNFs and thick CNFs possess the lower values of
β (1303 and 967), the use in catalyst support of these CNFs with microporous/mesoporous structures is prospective, also a better field emission performance can be obtained by a design modification.
Conclusions
Three types of CNF (filamentous CNF, chain-like CNF, and thick CNF) were successfully prepared from three hydrocarbon precursor gases: ethylene (C2H4), n-hexane (n-C6H14) and n-dodecane (n-C12H26) on iron catalyst at 1073 K. A large dependence of the morphology, structure and grow kinetics of the CNFs on the precursor gas was discovered. Detailed characterization revealed that the size of iron catalyst nucleated in the incubation period dramatically altered with the type of the precursor gas. Small iron catalyst particles with smooth surface nucleated in C2H4 precursor gas, possessing the highest activity, developed the typical filamentous CNFs (∼50 nm) with highly ordered graphene layers. Iron catalyst particles with moderate sizes nucleated in n-C6H14 precursor gas and extruded into smaller nano-catalyst to develop chain-like CNFs (∼80 nm). Large iron catalyst particles with irregular shape and rough surface generated in n-C12H26 precursor gas, possessing much lower activity, developed the thick CNFs (∼500 nm) with numerous micropores and mesopores in the disordered graphene layers. Also it was found that the filamentous CNFs possessed a much higher field emission property than the other two, while the microporous/mesoporous structures of the chain-like CNFs and thick CNFs certainly make them more appealing for use in heterogeneous catalysis.
Acknowledgements
This project is supported by the National Natural Science Foundation of China under the contract of 51431003.
Notes and references
- V. P. Veedu, A. Cao, X. Li, K. Ma, C. Soldano, S. Kar, P. M. Ajayan and M. N. Ghasemi-Nejhad, Nat. Mater., 2006, 5, 457–462 CrossRef CAS PubMed.
- M. Kumar and Y. Ando, J. Nanosci. Nanotechnol., 2010, 10(6), 3739–3758 CrossRef CAS PubMed.
- Y. He, W. Chen, C. Gao, J. Zhou, X. Li and E. Xie, Nanoscale, 2013, 5, 8799–8820 RSC.
- S. Shahgaldi and J. Hamelin, Carbon, 2015, 94, 705–728 CrossRef CAS.
- P. Serp, M. Corrias and P. Kalck, Appl. Catal., A, 2003, 253, 337–358 CrossRef CAS.
- A. Vinu, K. Ariga, T. Mori, T. Nakanishi, S. Hishita, D. Golberg and Y. Bando, Adv. Mater, 2005, 17, 1648–1651 CrossRef CAS.
- E. Hammel, X. Tang, M. Trampert, T. Schmitt, K. Mauthner, A. Eder and P. Pötschke, Carbon, 2004, 42, 1153–1158 CrossRef CAS.
- A. Bianco, K. Kostarelos, C. D. Partidos and M. Prato, Chem. Commun., 2005, 571–577 RSC.
- S. Iijima and T. Ichihashi, Nature, 1993, 364, 737 CAS.
- D. Sebastián, I. Suelves, M. Lázaro and R. J. Moliner, Power Sources, 2009, 192, 51–56 CrossRef.
- A. V. Melechko, V. I. Merkulov, T. E. McKnight, M. Guillorn, K. L. Klein, D. H. Lowndes and M. L. J. Simpson, Appl. Phys., 2005, 97, 041301 Search PubMed.
- M. Meyyappan, J. Phys. D: Appl. Phys., 2009, 42, 213001 CrossRef.
- Ç. Öncel and Y. Yürüm, Fullerenes, Nanotubes, Carbon Nanostruct., 2006, 14, 17–37 CrossRef.
- J. Zhu, A. Holmen and D. Chen, ChemCatChem, 2013, 5(2), 378–401 CrossRef CAS.
- D. H. Long, W. Li, M. Jin, W. M. Qiao, L. C. Ling, M. Isao and S. H. Yoon, Chem. Mater., 2011, 23(18), 4141–4148 CrossRef CAS.
- S. H. Park, S. B. Yoon, H. K. Kim, J. T. Han, H. W. Park and J. Han, et al., Sci. Rep., 2014, 4 Search PubMed.
- X. Ge, M. Chen, J. Wang, D. Long, L. Ling, W. Qiao, M. Isao and S. H. Yoon, RSC Adv., 2016, 6(8), 6443–6450 RSC.
- R. Baker, M. Barber, P. Harris, F. Feates and R. Waite, J. Catal., 1972, 26, 51–62 CrossRef CAS.
- A. La Torre, M. d. C. Giménez-López, M. W. Fay, G. A. Rance, W. A. Solomonsz, T. W. Chamberlain, P. D. Brown and A. N. Khlobystov, ACS Nano, 2012, 6, 2000–2007 CrossRef CAS PubMed.
- G. Hong, Y. Chen, P. Li and J. Zhang, Carbon, 2012, 50, 2067–2082 CrossRef CAS.
- R. Baker, Carbon, 1989, 27, 315–323 CrossRef CAS.
- M. Li, Y. Zhu and C. Zhou, Corros. Sci., 2015, 98, 81–87 CrossRef CAS.
- J.-W. Snoeck, G. Froment and M. J. Fowles, Catalysts, 1997, 169, 240–249 CAS.
- J. Chinthaginjala, D. Thakur, K. Seshan and L. Lefferts, Carbon, 2008, 46, 1638–1647 CrossRef CAS.
- N. Jeong and J. J. Lee, Catalysts, 2008, 260, 217–226 CAS.
- C. T. Wirth, B. C. Bayer, A. D. Gamalski, S. Esconjauregui, R. S. Weatherup, C. Ducati, C. Baehtz, J. Robertson and S. Hofmann, Chem. Mater., 2012, 24, 4633–4640 CrossRef CAS.
- J. Ge, G. Fan, Y. Si, J. He, H.-Y. Kim, B. Ding, S. S. Al-Deyab, M. El-Newehy and J. Yu, Nanoscale, 2016, 8, 2195–2204 RSC.
- N. A. Jarrah, J. G. van Ommen and L. J. Lefferts, Catalysts, 2006, 239, 460–469 CAS.
- B. Sirjean; E. Dames; D. Sheen; X. You; C. Sung; A. Holley; F. Egolfopoulos; H. Wang; S. Vasu and D. Davidson. JetSurF version 1.0, 2009 Search PubMed.
- L. Isett and J. Blakely, Surf. Sci., 1976, 58, 397–414 CrossRef CAS.
- J. Yu and S. Eser, Ind. Eng. Chem. Res., 1997, 36, 574–584 CrossRef CAS.
- S. V. Kalpathy, N. B. Poddar, S. P. Bagley and M. J. Wornat, Proc. Combust. Inst., 2015, 35, 1833–1841 CrossRef CAS.
- J.-W. Snoeck, G. Froment and M. J. Fowles, Catalysts, 1997, 169, 240–249 CAS.
- I. J. Alstrup, Catalysts, 1988, 109, 241–251 CAS.
- W. Arabczyk and U. Narkiewicz, Surf. Sci., 1996, 352, 223–227 CrossRef.
- E. Kukovitsky, S. L'vov and N. Sainov, Chem. Phys. Lett., 2000, 317, 65–70 CrossRef CAS.
- L. Isett and J. Blakely, Surf. Sci., 1976, 58, 397–414 CrossRef CAS.
- A. Tanaka, S.-H. Yoon and I. Mochida, Carbon, 2004, 42, 591–597 CrossRef CAS.
- C.-W. Huang, H.-C. Wu, W.-H. Lin and Y.-Y. Li, Carbon, 2009, 47, 795–803 CrossRef CAS.
- R. H. Fowler and L. Nordheim, Proc. R. Soc. London, Ser. A, 1928, 119, 173–181 CrossRef CAS.
- J. H. Kang, D. H. Shin, K. N. Yun, F. A. Masud, C. J. Lee and M. J. Kim, Carbon, 2014, 79, 149–155 CrossRef CAS.
|
This journal is © The Royal Society of Chemistry 2016 |