DOI:
10.1039/C6RA04734C
(Review Article)
RSC Adv., 2016,
6, 30582-30597
Nanotechnology to rescue bacterial bidirectional extracellular electron transfer in bioelectrochemical systems
Received
22nd February 2016
, Accepted 11th March 2016
First published on 17th March 2016
Abstract
An electrically active bacterium transports its metabolically generated electrons to insoluble substrates such as electrodes via a process known as extracellular electron transport (EET). Bacterial EET is a crucial process in the geochemical cycling of metals, bioremediation and bioenergy devices such as microbial fuel cells (MFCs). Recently, it has been found that electroactive bacteria can reverse their respiratory pathways by accepting electrons from a negatively poised electrode to produce high-value chemicals such as ethanol in a process termed as microbial electrosynthesis (MES). A poor electrical connection between bacteria and the electrode hinders the EET and MES processes significantly. Also, the bidirectional EET process is sluggish and needs to be improved drastically to extend its practical applications. Several attempts have been undertaken to improve the bidirectional EET by employing various advanced nanostructured materials such as carbon nanotubes and graphene. This review covers the recent progress in the bacterial bidirectional EET processes using advanced nanostructures in the light of current understandings of bacteria–nanomaterial interactions.
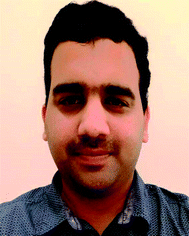 Shafeer Kalathil | Dr. Shafeer Kalathil received his master degree from the Dept. of Chemical Oceanography, Cochin University of Science and Technology, India in 2009. He has a Ph.D. Degree (2013) in Chemical Engineering & Technology from Yeungnam University, South Korea and later he moved to The University of Tokyo as a Japan Society for the Promotion of Science (JSPS)-Postdoctoral Fellow. His research interests encompass both the fundamental electrochemistry involved in bacterial extracellular electron transport and bionanotechnology. He has published 19 international peer-reviewed papers (h-index: 15) and has 8 patents to his credit. Currently he is working as a Postdoctoral Fellow at KAUST. |
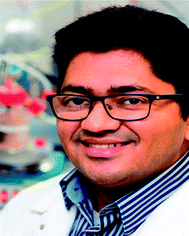 Deepak Pant | Dr. Deepak Pant is a Senior Scientist at the Flemish Institute for Technological Research (VITO), Belgium. His research focuses on bioenergy, specifically, the design and optimization of bio-electrochemical systems for energy recovery from wastewater and microbial electrosynthesis for production of value-added chemicals through electrochemically driven bio-processes. He has 1 book (published by Springer), 1 patent, 55 peer-reviewed publications with >2750 citations (h-index 26) and 16 book chapters to his credit. He is a member of scientific communities including ISMET, ISE, BES, BRSI, IFIBiop and AMI. He serves as an Editorial board member for the Journals: ‘Bioresource Technology’, ‘Electronic Journal of Biotechnology’, ‘Biofuel Research Journal’, ‘Heliyon’ and ‘Frontiers in Environmental Science’. |
1. Electrochemically active bacteria and extracellular electron transfer
Certain electrochemically active bacteria (EAB) commonly referred to as “exoelectrogens” (e.g., Geobacter and Shewanella) transport their metabolically generated electrons to insoluble substrates such as solid electrodes via a process known as extracellular electron transport (EET).1,2 The bacterial EET process plays a crucial role in bioremediation, energy recovery in systems known as microbial fuel cells (MFCs) and geochemical cycling of metals.3–5 Together, these systems are referred to as bioelectrochemical systems (BESs). Among the different BESs, MFCs have gained considerable attention over the years, owing to their dual functions in electricity generation and wastewater treatment.6,7 Shewanella oneidensis MR-1 and Geobacter sulfurreducens are the most extensively studied exoelectrogens to date.2
1.1. Electron transfer mechanism-electron release
Bacterial EET involves the release of metabolically generated electrons through a series of protein networks to the external insoluble electron acceptors. Even though, numerous studies are available on the molecular mechanism involved in the EET, the actual mechanism still remains as enigmatic. There are three primary mechanisms available to describe the bacterial EET process namely, (1) direct electron transfer via outer membrane c-type cytochromes (OM c-Cyts),8 (2) shuttling of electrons via cell-secreted flavin9 and (3) via bacterial nanowires.1,10 A collective contribution of the above three mechanisms mostly notices in the bacterial EET process. In contrast to shuttling mechanism directed by cell-secreted flavins such as riboflavin (RF) and flavin mononucleotide (FMN), Okamoto et al. has recently proposed that RF, and FMN specifically bind to OM c-Cyts, OmcA and MtrC proteins, respectively and form bound-flavin semiquinones.11–14 These bound-flavin semiquinones enhance the bacterial EET by 103 to 105-times more than the free flavin. These findings suggest that the interaction of flavin/OM c-Cyts is critical for the bacterial EET process.
Microbial nanowires have been considered as the sophisticated electrical cables produced by bacteria to transfer the electrons to solid electron acceptors. There is a misleading terminology regarding with bacterial nanowires and pili in the existing literature. Most of the reports describe nanowires as the extensions of the bacterial pili.10 However, a recent study on Shewenella nanowires has proposed that the nanowires are extensions of the outer membrane and periplasm which is against the previous notion of pilin based structures.10 Multiheme cytochromes are localized on theses nanowires which provide them excellent electrical conductivity. Deciphering the role of microbial nanowires on the EET process has recently received much attention among microbiologists, physicists, and chemists.15–19 A detailed understanding on the microbial nanowires will have tremendous implications in various nanobiotechnological applications, bioelectricity generation, corrosion and bioremediation.16–18,20 A breakthrough work from Malvankar et al.21,22 demonstrated that nanowires produced by G. sulfurreducens behave like metallic conductors. The conductivity of the nanowires was significantly enhanced by cooling, which is a signature of metallic conduction. Also, there was two orders of high conductivity in the nanowires with decreasing pH. This observation suggested that pili were doped with protons and the protonation resulted in p-type carriers similar to the previous observations in organic metals such as polyaniline.23 Similarly, nanowires produced by S. oneidensis MR-1 exhibit p-type, tunable electronic behavior, and holes are found to be the primary charge carriers in the bacterial nanowires.24 However, a live debate is still going on for establishing a consensus in the molecular mechanism involved in the bacterial EET process.25–31
1.2. Electron transfer mechanism-electron uptake
Interestingly, some microbes sense negatively charged surfaces by up-taking electrons and use the surfaces as the electron donors for driving their metabolism. Recent studies have shown this exciting activity of the microbes that can accept electrons from a cathode to synthesize high-value chemicals and fuels such as acetate and methane via a process known as microbial electrosynthesis (MES, Fig. 1).32–36 The MES is considered as a promising technology for the production of chemicals and fuels from CO2.37 Only limited studies are available for describing the bacterial electron uptake mechanism from a cathode and a better understanding on the molecular mechanism is needed for the practical application of this technology.35 Electrochemical analyzes of c-Cyts in Geobacter species showed that the electron transfer ability of c-Cyts may be reversible.35 Also, some studies proposed the collective role of c-Cyts and hydrogenase enzymes in the bacterial electron uptake.35,38–40 A recent report revealed that S. oneidensis MR-1 reverse its respiratory pathway for reductive metabolism.41 S. oneidensis reduced fumarate to succinate by accepting electrons from the negatively poised graphite electrode. Deletions of OM c-Cyt anchoring protein-MtrB and periplasmic cytochrome MtrA severely reduced the fumarate reduction in S. oneidensis. Further investigations are needed to elucidate the exact mechanism involved in the bacterial electron uptake from the cathode.
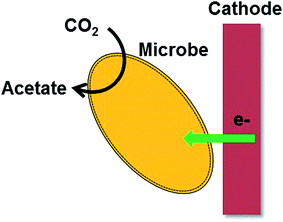 |
| Fig. 1 A cartoon representation of microbial electrosynthesis of acetate from CO2. | |
1.3. Role of electrode material in EET
The finding of bacterial bidirectional EET has led to the development of MFC and MES technologies. However, these technologies are still far from practical applications due to many inherent obstacles such as reduced biofilm conductivity and poor bacteria-electrode electrical connection and limited understanding of bidirectional EET mechanism. Biofilm conductivity is believed to be a critical factor for the efficient EET process.42 Unfortunately, biofilm conductivity by itself is not enough to transfer electrons fluently to/from the electrode. Recently, material scientists have started to employ various advanced nanostructured materials such as carbon nanotubes, graphene, inorganic nanomaterials, noble metal nanoparticles, semiconductors and conducting polymers as bioanode or biocathode materials to enhance the bidirectional EET process. Especially, the efforts using nanostructured materials have led to a steep improvement in the bidirectional EET process. This review covers the recent progress in the bacterial bidirectional EET processes under the umbrella of nanostructured materials. A prior understanding of the fundamental mechanisms involved in the bacterial attachment to solid surfaces is essential as the biofilm formation is one of the critical process in the both MFCs and MES. Several attempts including theoretical and experimental studies have focused on investigating the bacterial attachment to various nanostructured surfaces. All the merits of using such nanomaterials in improving electron transfer in BESs are discussed along with current challenges and further perspectives.
2. Current understandings on bacteria–electrode interactions
In this Section, the current understandings of the bacterial interactions with electrode surfaces are discussed. The knowledge evolved from the bacteria–electrode interactions can be directly utilized to set up strategies for the development of efficient bioanode and biocathode materials. Bacteria usually prefer to form biofilms on solid surfaces to shelter from various environmental perturbations and toxicity.43 The bacterial biofilm formation has been extensively investigated, and it is known that bacteria secrete extracellular polymeric substance (EPS) to stick on a solid surface.43 Genetic analyses have proposed that flagella and pili are also associated with the biofilm formation.44 There are many factors that affect the formation of a biofilm such as surface topography, surface charge, wettability (of both the biofilm as well as the electrode) and the amount of EPS production, cell–cell signaling.45–48 Among them, the surface topography is known to play a critical role in the early stages of biofilm formation.49
Nanotechnology has emerged as a potential tool to investigate bacterial life on nano-sized crevices.50,51 Nanomaterials provide us various possibilities to tune the surface of the electrode to understand the interaction of bacterial cells with the modified surfaces. For example, it is possible to investigate the role of surface porosity or roughness on the biofilm attachment by applying different porous nanomaterials as the electrodes. Bacterial cells are highly sensitive to electrode surfaces and hence a prior understanding on bacterial affinity towards electrode is necessary to develop efficient electrode materials. Recently, silicon (Si) nanowire was employed to examine the bacteria–nanomaterial interaction as physical interactions between the bacteria and nanowires can be precisely measured. Sakimoto et al.52 submerged Si nanowires to a growth media containing Sporomusa ovata cells under a constant applied potential. The authors observed that S. ovata cells aligned parallel to the nanowires at high ionic concentration while at low ionic strength, bacterial cells attached to the nanowires in a random orientation (Fig. 2). The results have suggested a salt-induced orientation and self-assembly of the bacterial cells on the nanowires. Similarly, a recent report has elegantly shown that how S. oneidensis cells sense Si nanowires during their swimming and attach to the Si nanowire surfaces.53 The study revealed that S. oneidensis preferred to stay on the Si nanowires rather than the planar bottom silicon substrate and demonstrated that nano-sized surfaces play a crucial role in the development of biofilms.
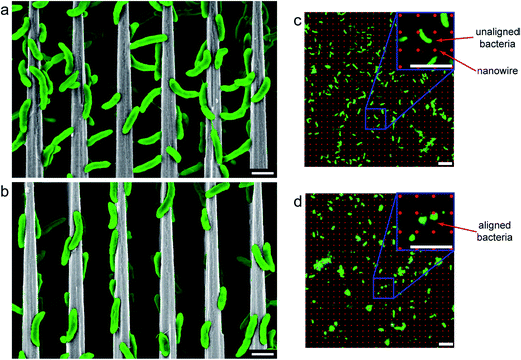 |
| Fig. 2 Scanning electron microscopy (SEM) and fluorescence micrographs of S. ovata on nanowire arrays. (a, b) SEM and (c, d) fluorescence images were taken of S. ovata cells grown on Si nanowire arrays of 4 μm periodicity under (a, c) normal ionic concentrations (∼100 mM) and 100 kPa H2 : CO2, showing random orientations with respect to the nanowire axis, and (b, d) with +200 mM NaCl and 500 kPa H2 : CO2, showing alignment with the nanowire. Both (a, b) side view SEM and (d, e) top down in situ fluorescence microscopy show alignment, with (c) unaligned bacteria appearing as rods in fluorescence micrographs, and (d) aligned bacteria appearing as circles. Scale bars are (a, b) 2 and (c, d) 5 μm (reproduced from ref. 52 with the permission from American Chemical Society).52 | |
Singh et al.54 reported that nanostructured titania film with a surface roughness of 20 nm enhances biofilm formation, and a further enhancement in the surface roughness inhibits the bacterial attachment. Mitik-Dineva et al.55 showed that surface with a nano-scale roughness stimulated the bacterial attachment three-fold higher than the micro or macro-sized surface roughness. They investigated the role of nano-roughness on the bacterial attachment by forming a Pseudomonas isachenkonni (a proteobacterium) biofilm on a glass substrate with a modified nano-scale roughness. A higher number of bacterial cells was attached to the modified glass substrate compared to the unmodified glass. Also, there was a morphological transformation of the cells on the nano-sized rough surface by an increase in the cell size and EPS production. These results clearly indicated that nano-surface induced the cellular metabolism of the attached bacterial cells. Recently Ding et al.56 demonstrated the crucial role of surface wettability on the biofilm attachment and the bacterial EET process. To investigate the role of wettability on the EET, tin-doped In2O3 (ITO) with different wettability were used to develop Shewanella loihica PV-4 biofilms at a constant applied potential. The biofilm formed on the hydrophilic ITO glass substrate delivered 5-times higher EET activity than the biofilm grown on the hydrophobic substrate (Fig. 3). Electrochemical measurements revealed that the surface wettability enhanced the electrical connection between the microbes and electrode by altering local polarity at microbe/electrode interface. The alteration in the local polarity affected the redox state of OM c-Cyts, which allowed them to stay mostly in the reduced state. The redox state variation in the OM c-Cyts with the hydrophilic surface drastically enhanced the EET process. In short, high surface roughness or porosity allows the bacteria to attach firmly on the electrode surface that helps them to transfer the electrons easily to the electrode. Also, hydrophilic surface promote the biofilm formation and regulates the EET process.
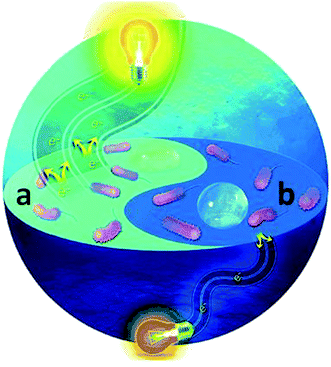 |
| Fig. 3 Graphical illustration showing a higher current generation from a S. PV-4 biofilm developed on a (a) hydrophilic electrode surface (more electrons moving towards the resistor (light bulb)) (a) than (b) a hydrophobic surface (less electrons are shown moving) (color image only) (reproduced from ref. 56 with permission from John Wiley and Sons).56 | |
3. Enhancement of bacterial EET by nanostructured materials
The previous decade has seen a lot of research efforts being made towards the development of newer electrode materials as both anode and cathode for BESs.57,58 These include different types of carbon and metal based catalysts.59,60 Of late, several nanomaterials have emerged as promising materials for both anode and cathode construction.61 These are believed to improve the BES performance in terms of bacterial adhesion, electron transfer and electrochemical efficiency. The application of various nano-structured electrode materials significantly enhanced the performance of the BESs and Table 1 shows a comparison of the performance of BESs with different nanostructured bioanode materials.
Table 1 Shows performance of biolectrochemical system (BES) with different nanostructured bioanode materials
Type of BES |
Type of electrode materials |
Inoculum |
Current density (mA cm−2) |
Reference |
H-shaped MFC |
CNT–textile anode, carbon cloth (0.5 mg cm−2 10 wt% Pt on XC-72) cathode |
Domestic wastewater |
0.72 |
62 |
H-shaped MFC |
CNT–sponge anode, CNT–sponge–Pt cathode |
Domestic wastewater |
2.13 |
63 |
H-shaped MFC |
Crinkled CNT anode, carbon cloth (0.5 mg cm−2 10 wt% Pt on XC-72) cathode |
Domestic wastewater |
0.24 |
64 |
Three-electrode system |
NanoWeb-RVC anode, Ag/AgCl reference electrode, Ti mesh counter electrode |
Anodic effluent from an existing BES |
6.8 |
65 |
Two chamber MFC separated by Nafion membrane |
Fe3O4/CNT anode, 20 wt% Pt coated carbon cloth cathode |
E. coli K12 |
0.19 |
66 |
Micro-sized MFC (1.25 μL) |
MWCNTs anode, carbon cloth cathode |
Mixed culture |
0.0197 |
67 |
Single chamber MFC |
CNT–hydrogel anode, Pt-coated (0.05 mg cm−2) carbon cloth cathode (air cathode) |
Anaerobic sludge |
0.032 |
72 |
H-type MFC |
CNT/MnO2 anode, Pt coated carbon paper cathode |
Anaerobic sludge |
0.0262 |
73 |
Micro-sized MFC |
Graphene sheet on nickel anode, Pt coated carbon cloth cathode (air cathode) |
Domestic wastewater |
0.0034 |
81 |
Two chamber MFC separated by Nafion membrane |
Chitosan/vacuum stripped graphene anode, plain carbon cloth cathode |
Pseudomonas aeruginosa |
0.255 |
83 |
Three electrode system |
Ionic liquid functionalized graphene nano-sheets anode, Ag/AgCl reference electrode, Pt mesh counter electrode |
Shewanella oneidensis MR-1 |
0.28 |
87 |
Three electrode system |
Graphene oxide nanoribbons anode, Ag/AgCl reference electrode, Pt wire counter electrode |
Shewanella oneidensis MR-1 |
0.19 |
88 |
H-type MFC |
Graphene–CNT hybrid anode, carbon felt cathode |
Shewanella oneidensis MR-1 |
0.123 |
92 |
Single chamber MEC |
Au nanoparticle-coated graphite disc anode, carbon cloth (0.5 mg cm−2 10 wt% Pt on XC-72) cathode |
Shewanella oneidensis MR-1 |
0.0744 |
93 |
Single chamber MEC |
Pd coated graphite disc anode, carbon cloth (0.5 mg cm−2 10 wt% Pt on XC-72) cathode |
Shewanella oneidensis MR-1 |
0.0088 |
93 |
H-type MFC |
Au nanoparticle-coated carbon paper anode, ferricyanide solution catholyte |
Domestic wastewater |
0.118 |
94 |
Three electrode system |
Goethite nanowhiskers modified carbon paper anode, Ag/AgCl reference electrode, Pt wire counter electrode |
Shewanella loihica PV-4 |
0.048 |
108 |
Single chamber MFC |
Carbon coated titanium dioxide core–shell nanoparticle anode, carbon cloth (0.5 mg cm−2 10 wt% Pt on XC-72) air-cathode |
Anaerobic sludge |
1.2 |
109 |
H-type MFC |
NiO nanoflakes/carbon cloth anode, ferricyanide catholyte |
Shewanella putrefaciens |
0.25 |
113 |
H-type MFC |
Polypyrrole membrane anode, ferricyanide catholyte |
Shewanella oneidensis MR-1 |
0.21 |
118 |
Three electrode system |
Polypyrrole nano-arrays anode, Ag/AgCl reference electrode, Pt wire counter electrode |
Shewanella loihica PV-4 |
0.32 |
119 |
Three electrode system |
Nano-colloidal Fe2O3 coated ITO photo bioanode, Ag/AgCl reference electrode, Pt wire counter electrode |
Shewanella loihica PV-4 |
0.033 |
126 |
Three electrode system |
Fe2O3 coated ITO photo bioanode, Ag/AgCl reference electrode, Pt wire counter electrode |
Shewanella oneidensis MR-1 |
0.25 |
127 |
3.1. Carbon nanotube-based nanostructures
The combination of the remarkable conductivity, high specific surface area, and biocompatibility make carbon nanotube (CNT) as a suitable candidate for the development of bioanode materials in MFCs. CNT-based electrode materials significantly improved the performance of the bioanode by enhancing the bacterial EET. Xie et al.62 used a three-dimensional (3D) macro-porous CNT–textile anode to improve the MFC performance drastically as compared to traditional electrodes such as carbon cloths. 3D macro-scale porous structure enabled bacteria to colonize both interior and exterior of the anode allowing an efficient electroactive biofilm formation. In contrast, 2D structure precludes biofilm formation at the interior of the electrode making less surface area available for the bacterial attachment. The macroporous structure further allows unhindered diffusion of the substrate to the inner part of anode, which maintained internal bacterial colonization. Also, CNT–textile fibers facilitated bacterial EET by forming a compact attachment with the microbial nanowires (Fig. 4). Electrochemical impedance spectroscopy (EIS) revealed a 10-fold lower charge transfer resistance of CNT–textile fibers as compared to a carbon cloth. A follow-up work using CNT coated-sponge as an anode electrode produced higher current density than that of the CNT–textile fiber electrode.63 The increased performance of CNT–sponge electrode was attributed to lower internal resistance, controllable and tunable pore sizes and excellent mechanical stability. Similar to the CNT–textile fiber, 3D and macroporous structure of the CNT–sponge allowed enhanced diffusion of the electrolyte for the internal bacterial colonization. A crinkled CNT anode showed a better performance for the production of bioelectricity relative to an uncrinkled CNT due to its enhanced interaction with exoelectrogens by forming an intimate contact with microbial nanowires.64 The performance of crinkled CNT as the bioanode material was comparable with the CNT doped-sponge electrode.63 Flexer et al.65 developed an efficient bioanode for MFCs by decorating CNTs on macroporous reticulated vitreous carbon (RVC) via chemical vapor deposition (NanoWeb-RVC). The modified bioanode exhibited three times higher current density over the control by mixed culture bacteria as biocatalysts. The macroporous structure of the electrode provided excess electroactive surface area for the biofilm formation and scanning electron micrographic (SEM) images clearly showed thick reddish colored biofilms on the electrode surface. A rich c-Cyts content may be responsible for the red coloration of the biofilm and rod shape microbes were mainly identified in the biofilm, which were very similar to Geobacter species. Cyclic voltammetry (CV) analysis showed that the NanoWeb electrode greatly enhanced the bacterial EET by making a healthy interaction with OM c-Cyts and bacterial nanowires.
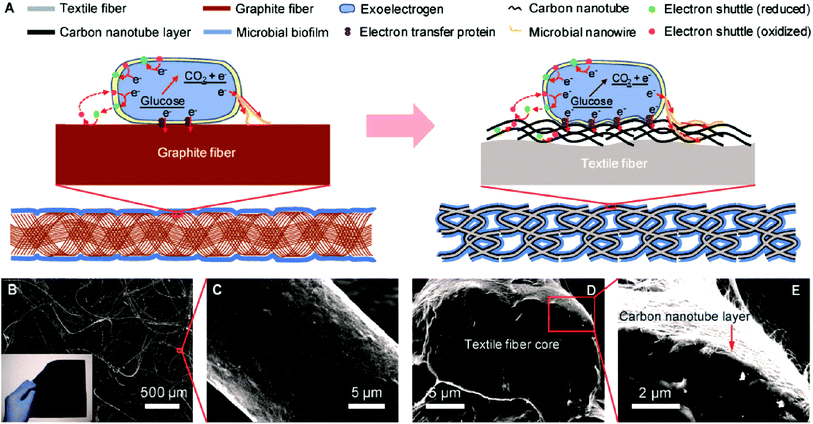 |
| Fig. 4 Carbon nanotube–textile (CNT–textile) composite. (A) Schematic of the electrode configuration and electron-transfer mechanisms for the CNT–textile anode (right), compared with the widely used carbon cloth anode (left). (B) Scanning electron microscope (SEM) image of the CNT–textile showing the open macroscale porous structure. The inset is a 15 cm × 15 cm piece of CNT–textile with a sheet resistance of 4 Ω per square. (C) SEM image of a textile fiber conformally coated with CNTs. (D, E) Cross section of a CNT–textile fiber. The diameter of the CNT–textile fiber is about 20 μm, and the thickness of the CNT coating is about 200 nm (reproduced from ref. 62 with permission from American Chemical Society).62 | |
The combination of CNTs with other conductive materials such as hematite and magnetite also exhibited remarkable improvements in the efficiency of bioanodes. Mono-dispersed magnetite (Fe3O4)/CNT nano-composite modified carbon paper exhibited an increased current generation over the control using E. coli as a biocatalyst.66 Magnetic properties of Fe3O4 allowed CNTs to attach to the carbon paper firmly while CNTs offered excellent conductivity for the bacterial EET process. CV analysis revealed the improved electrochemical activity of E. coli biofilm due to the presence of Fe3O4/CNT nano-composite, which was further confirmed by the impedance measurement. The role of Fe3O4 in the bacterial EET process will be discussed in detail in the Section 3.4. Vertically aligned, forest type multi-walled CNT with nickel silicide anode produced a high power density in a micro-sized MFC (1.25 μL).67 Similarly, a CNT modified anode showed a large current generation in a micro-sized MFC (75 μL) due to high surface area and outstanding conductivity of the CNT.68 Advances in micro-sized MFCs will allow them to power ultra-low-power electronics. A 3D hierarchical macroporous chitosan dispersed multi-walled CNT bioanode permitted to form an efficient electroactive G. sulfurreducens biofilm.69 The synergetic effect of macroporous structure and the excellent conductivity of CNTs induced the EET process of G. sulfurreducens significantly. Liu et al.70 experimentally and theoretically demonstrated that CNT-networks can significantly enhance the EET process in S. oneidensis MR-1 by producing a ten times larger current generation in an MFC as compared to a carbon paper electrode. CV analysis showed that there was a strong interaction between CNT-network and OM c-Cyts, which promoted the EET process. Mutant experiment lacking MtrC and OmcA significantly decreased the current production indicating the inevitable role of OM c-Cyts in the EET process. Molecular dynamic simulations confirmed the increased interaction of Fe2+ in reduced heme groups of OM c-Cyts and CNTs. Peng et al.71 also observed a similar promoting effect of CNTs on the direct electron transfer in S. oneidensis MR-1.
Doping of CNTs with different materials showed improved performance of the bioanodes. A CNT-doped hydrogel deposited on a carbon paper produced larger current relative to the control.72 A high content of oxygen-containing functional groups on the surface of conducting CNTs and large specific surface area were responsible for the improved performance of the MFC. XPS analysis confirmed the presence of a quinone structure on the surface of CNTs, which could act as a redox mediator for the EET process. Recently, an MFC with CNT/MnO2 modified anode produced a higher current generation than that of a plain carbon paper.73 Large surface area, excellent conductivity and supercapacitance behavior of MnO2 were attributed to the enhanced performance of the CNT/MnO2 modified electrode. Zhang et al.74 demonstrated that thicker biofilm formation on CNT-modified graphite bioanode deteriorated the MFC performance and thin biofilms (<5 μm) are only feasible to accelerate the EET process. This observation raises a significant concern for the usage of CNT-modified bioanode as G. sulfurreducens, a high current producing bacterium usually form a thick biofilm (>20 μm).75 Erbay et al.76 systematically investigated the role of the geometry of CNTs on the performance of MFCs. They demonstrated that longer CNTs delivered higher current density than the smaller CNTs, which is possibly due to the large surface area of the former. Also, densely packed CNT network showed a lower performance as compared to loosely packed CNTs. The dense CNT networks hindered the passage of microbes into the interior, resulting in a biofilm formation only on the top of CNT-assembly while loosely packed CNTs allowed bacterial colonization in both exterior and interior of the bioanode. An addition of amorphous carbon on the CNT-modified anode significantly reduced the current density due to the hindrance in the bacterial EET. These results are highly useful to design efficient CNT-modified bioanodes for high-performance MFCs.
In addition to the ability of CNT to enhance the EET, it can even alter the bacterial metabolism. Recently Yan et al.77 reported that addition of CNTs into S. oneidensis MR-1 shifted the intracellular reduction of nitrobenzene (NB) to extracellular. It indicated that CNTs could alter the electron flow route in S. oneidensis. Wild-type and mutant strains lacking OM c-Cyts exhibited similar NB reduction rate. Intriguingly, the addition of CNTs to the wild-type MR-1 accelerated NB reduction while mutant strains were unaffected by CNTs. The extracellular NB reduction could slow down the diffusion of NB into the interior of the cells, allowing a high microbial activity due to the lower toxic effect of the NB. The results suggested the existence of an intimate contact of CNTs with OM c-Cyts to enhance the extracellular NB reduction and possibilities of other nanostructures to alter the electron transfer route in Shewanella. A very recent report demonstrated that CNTs have a strong affinity towards Saccharomyces cerevisiae cells, and the CNT/S. cerevisiae bioanode exhibited an increased current generation in the MFC.78
3.2. Graphene-based nanostructures
Similar to CNTs, graphene has been extensively used for developing electrodes in MFCs due its outstanding electrical conductivity, high specific surface area, and excellent mechanical strength. Graphene modified stainless steel mesh (GMS) bioanode produced 18-times higher electricity in an MFC as compared to the bare stainless steel mesh.79 The GMS provided large surface area for the bacterial adhesion and lowered the charge transfer resistance significantly to enhance the EET process. 3D graphene sponge bioanode prepared by an ice template method showed a better performance over graphite felts in MFCs owing to its high surface area and low charge transfer resistance.80 A micrometer-sized MFC equipped with a graphene anode produced a power output of ∼I nW,81 which is sufficient to run ultra-low power electronics.82 A biocompatible chitosan/vacuum stripped graphene scaffold (CHI/VSG) bioanode produced a 78-times higher current density over the control in a Pseudomonas aeruginosa bio-catalyzed MFC.83 P. aeruginosa usually secretes endogenous mediators such as phenazine to accelerate the EET process, and a previous report demonstrated that graphene enhances the production of phenazine.84 Here, the VSG significantly stimulated the secretion of endogenous redox mediators and also provided large surface area for the biofilm formation. The macroporous structure of the CHI/VSG composite permitted an undisturbed flow of the substrate to the inner core of the anode, promoting internal bacterial colonization. Also, EIS measurement revealed a reduced charge transfer resistance, which facilitated the EET process in P. aeruginosa.
Graphene like other carbon materials is highly hydrophobic, which makes less affinity towards the bacterial attachment as compared to other carbonaceous materials.85 Yong et al.85 deposited a conducting polymer, polyaniline (PANI) which is highly hydrophilic in nature on graphene nanostructures to make PANI hybridized 3D graphene bioanode in MFCs. The surface hydrophilicity allowed a thicker biofilm formation on the modified bioanode and a very recent study suggested that the surface hydrophilicity can stimulate the EET process.56 The PANI/graphene bioanode delivered improved current generation relative to traditional carbon electrodes. SEM images revealed the formation of biofilm in both interior and exterior of the modified electrode while bare 3D graphene possessed biofilm at exterior only. PANI is positively charged in the neutral condition, which can have an intimate electrostatic contact with negatively charged bacterial membrane surfaces.86 CV analysis confirmed that PANI/graphene foam facilitated direct electron transfer in S. oneidensis. This enhanced direct electron transfer mechanism depicts that OM c-Cyts created a firm contact with PANI/graphene by developing an efficient electronic conduit. Ionic liquid functionalized graphene nano-sheets decorated on a carbon paper anode (CP-IL-GNS) delivered 7-fold higher bio-current generation over the control in a S. oneidensis MFC.87 The IL-GNS created a positively charged surface on the carbon paper for the electrostatic attraction of negatively charged Shewanella cells (Fig. 5). Also, the IL-GNS provided high conductivity and large surface area for the improved electron transfer from bacteria to the electrode.
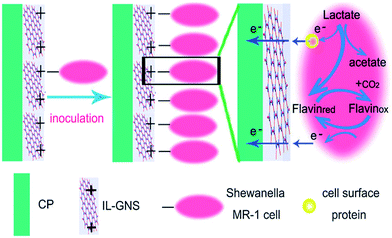 |
| Fig. 5 Schematic illustration of the interactions between the CP/IL-GNS anode and S. oneidensis cells (reproduced from ref. 87 with permission from Royal Society of Chemistry).87 | |
Graphene oxide (GO) nano-ribbons deposited on a carbon paper significantly enhanced direct electron transfer process in S. oneidensis MR-1.88 The authors proposed that GO nano-ribbons behaved as conductive microbial nanowires to facilitate the bacterial EET process. Reduced GO decorated on nickel foam (rGO/Ni) showed an improved electricity production in a S. oneidensis MFC.89 The enhanced performance was attributed to macro-porosity and high conductivity of the rGO/Ni. Yong et al.90 developed a self-assembled 3D rGO/bacterial biofilm via in situ reduction of GO by S. oneidensis MR-1. As-formed rGO/Shewanella biofilm showed 25-fold enhancement in the EET as compared to naturally occurring biofilms. The 3D hybrid biofilm demonstrated an outstanding electrochemical activity and improved direct electron transfer rate between the cells and electrode. The rGO interconnected the cells in the biofilm, which lowered the charge transfer resistance significantly over the natural Shewanella biofilms. Similarly, in situ reduction of GO via microbial respiration enhanced the bacterial EET by forming a graphene/biofilm scaffold.91 Electrochemical measurements demonstrated that as-formed graphene structure induced exoelectrogens to accelerate the EET rate. A recent report from Zhao et al.92 demonstrated that Shewanella biofilm formed on a hybrid graphene–CNT electrode exhibited an improved EET activity for bioelectricity generation. The modified electrode was developed by inoculating S. oneidenis MR-1 cells to a solution containing GO and CNTs with lactate as the electron donor. After two days of the inoculation, the non-conducting GO was converted to conducting graphene structures by accepting electrons from Shewanella cells. As-formed G-CNT/biofilm was collected by centrifugation and deposited on an acid-treated carbon felt electrode. The acid-treatment created a positively charged surface on the carbon felt, which attracted the negatively charged G-CNT/biofilm. Fig. 6 shows a schematic illustration of the formation of hybrid G-CNT/biofilm and the EET process from the biofilm to the electrode. The modified electrode resulted in an enhanced bacterial loading and improved direct electron transfer process via OM c-Cyts.
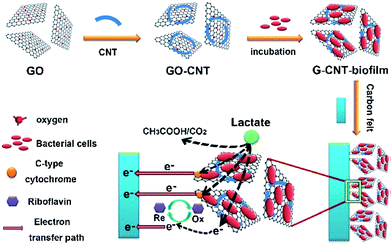 |
| Fig. 6 Fabrication of hybrid electroactive biofilm with built-in bacteria, and electron transfer process from hybrid biofilm to electrode (reproduced from ref. 92 with permission from John Wiley and Sons).92 | |
3.3. Noble metal nanomaterials
Platinum and platinum group metals have been used for a long time in MFC electrodes especially cathodes.59,60 It is not surprising therefore that noble metal nanoparticles have been explored as materials for improved catalytic performance of these systems. Gold (Au) and palladium (Pd) nanoparticles decorated graphite electrodes delivered excellent current production in MFCs over the controls.93 Significant enhancement in the current generation observed with particle sizes greater than 200 nm, possibly due to the solid direct contact with the bacterial cells. A negative correlation between the current density and the nanoparticle density was observed. In contrast, Alatraktchi et al.94 found an increased current generation with Au nanoparticle density. DNA sequencing has shown that the high density of Au nanoparticles increased the population of exoelectrogenic bacteria. Similarly, Au nanoparticles deposited on a carbon paper produced 2.9 times higher current over the bare carbon paper.95 Using single crystal approach, Maestro et al.96 demonstrated that crystal geometry of Au nanoparticles is critical for the bacterial EET. Three different single crystal forms of Au–Au (110), Au (111) and Au (210) were employed to investigate the effect of the crystal orientation on the EET activity in G. sulfurreducens. The crystal orientation, Au (210) exhibited enhanced current generation over the remaining crystal orientations. The result sheds light on the fact that similar to the type of electrode materials, the crystallographic nature of the material is also crucial for the development of efficient bioanodes.
Beyond Au, Pd has also been investigated for its ability to improve EET in BESs. Wu et al.97 demonstrated that membrane bound-Pd nanoparticles significantly improved the bacterial EET by facilitating electron transfer between cells and electrode. Similarly, membrane bound-Au nanoparticles in S. oneidensis cells greatly enhanced the direct electron transfer from cells to the electrode surface.98 Outer membrane proteins MtrC and OmcA are believed to be the key redox proteins for the EET in S. oneidensis MR-1.8 Intriguingly, mutant strains lacking OmcA/MtrC showed a similar EET rate with the wild-type in the presence of membrane-bound Au nanoparticles implying that the EET chain in S. oneidensis could be repaired by the Au nanoparticles. A similar report proposed that magnetite nanoparticles can compensate c-Cyts (MtrC/OmcA) in G. sulfurreducens, and it will be discussed in the Section 3.4. later.99 The results led to the conclusion that nanomaterials can mimic membrane-bound proteins, which warrant further extensive research. A seminal work from Xiao et al.100 demonstrated that Au nanoparticles wired-flavin adenine dinucleotide (FAD) transferred electrons to a conductive electrode much faster (∼5000 per second) compared with the rate at which molecular oxygen, the natural co-substrate of the enzyme, accepted electrons (∼700 per second). Here, the Au nanoparticle acted as an “electrical nanoplug” to firmly attach the enzyme on the conductive surface, which enhanced the electrical conductivity of the redox enzyme. As a series of redox enzymes are involved in the bacterial EET, this information is crucial for the understanding of Au nanoparticle effect in the EET. However, depending on noble metals for the development of bioanode materials is not affordable for practical applications.
3.4. Inorganic nanomaterials
Various inorganic nanomaterials such as iron sulfide, goethite, magnetite, hematite, and nickel have been extensively used to accelerate the bacterial EET. Shewanella uses sulfur-mediated electron shuttling process for the metal reduction.101 In situ production of iron sulfide (FeS) nanoparticles in S. loihica PV-4 delivered two times larger current production in MFCs than the cells lacking the FeS nanoparticles.102 FeS is an abundant biogenic mineral existing in the nature. Mutant experiments lacking OM c-Cyts delivered 70% lower current density as compared to the wild-type. The substantial reduction in the current generation with mutant strains suggested that the Fe 3d orbital in the biogenic FeS nanoparticles interacted with OM c-Cyts forming an electrical conduit for the EET process. Similarly, S. loihica PV-4 with in situ generated biogenic FeS nanoparticles produced 500 pA current which was 3–4 times higher than the current generated from a single cell Shewanella or Geobacter.103 The FeS nanoparticles established an efficient electronic conduit for the EET process by interconnecting the remote bacterial cells that were not in direct contact with the electrode surface.
Magnetite (Fe3O4), an abundant natural mineral plays critical roles in bioremediation and geochemical cycling of metals.104,105 Many studies have proposed that Fe3O4 can facilitate EET processes in iron-reducing microbes.104,105 Kato et al.106 reported that nano-Fe3O4 enhanced interspecies electron transfer (IET) between G. sulfurreducens and Thiobacillus denitrifcans, accompanying acetate oxidation coupled to nitrate reduction. The Fe3O4 nanoparticles constructed an electrical bridge between these bacterial species to facilitate the IET process, revealing the role of conductive minerals in the bacterial EET process. In contrast, the addition of semiconductive-hematite (Fe2O3) did not exhibit any impact on the IET. Peng et al.107 also observed an enhanced current generation in MFCs by the addition of nano-Fe3O4. The investigation of the molecular mechanism behind the improved bacterial EET associated with Fe3O4 revealed that the Fe3O4 nanoparticles behave like c-type cytochrome, OmcS.99 A repressed expression of OmcS gene was observed in wild-type strains in the presence of Fe3O4, suggesting that the cells need to produce less OmcS, if Fe3O4 is available. The transmission electron microscopic images (Fig. 7) depicted that the nanoscale Fe3O4 were well dispersed in the Geobacter species by preferentially attaching on the conductive pili, which was responsible for the improved EET rate in G. sulfurreducens.
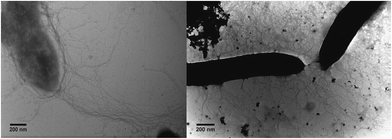 |
| Fig. 7 Transmission electron micrograph of magnetite-free (left) and magnetite-amended (right) G. metallireducens/G. sulfurreducens co-cultures. The white arrows point to magnetite associated with pili (reproduced from ref. 99 with permission from John Wiley and Sons).99 | |
Goethite nanowhiskers modified carbon paper bioanode delivered 60% higher current generation in an MFC relative to the bare carbon paper.108 The modified anode provided improved surface area for the biofilm formation, and also exhibited a low charge transfer resistance for the EET in S. loihica PV-4. Carbon coated titanium dioxide (TiO2) core–shell 3D anode demonstrated a high performance in MFCs due to its enhanced electrochemical capacitance.109 Previous reports suggested that TiO2 can increase the MFC performance by facilitating the EET process.110–112 Nickel oxide nanoflakes deposited on a carbon cloth (NiO nanoflakes/carbon cloth) rendered an extensive electroactive surface area and low interfacial charge transfer resistance to facilitate the EET in S. putrefaciens.113 Also, the deposition of NiO on the carbon cloth created a hydrophilic surface for the enhanced bacterial adhesion. Some studies reported a decline in the EET rate of S. oneidensis MR-1 over time due to the formation of dense biofilm, which hindered the diffusion of substrate and electron mediators such as flavins.114,115 A very recent report from Zhang et al.116 demonstrated that a complete suppression of biofilm formation on a carbon electrode by hexagonal WO3 nanorods promoted the flavin-mediated EET process in S. oneidensis. The smaller size and high crystallinity of the WO3 nanorods prevented the biofilm growth on the electrode surface, and planktonic Shewanella cells used flavins as shuttling molecules to enhance the EET process. This observation is concordant with a previous report from Lieber and co-workers.117 In this report, the authors designed a bio-electrochemical set up with two types of working electrodes to probe the EET activity in S. oneidensis MR-1. The first type of working electrode was developed by constructing nano-holes on a conducting ITO glass that can preclude cell attachment to the electrode surface. The second electrode was designed in such a way that the cells can easily attach to the electrode surface. Interestingly, S. oneidensis exhibited a similar EET activity with both electrodes. However, replacement of supernatant with fresh media severely reduced the EET activity in Shewanella and reintroduction of the original cell-free media caused a quick increase in the EET process. These observations clearly suggested that there is no need for direct contact of MR-1 cells to the electrode surface (no need of biofilm) and shuttling molecules are mainly responsible for the EET activity in MR-1 cells. However, these observations may not be applicable to G. sulfurreducens as they secrete very less shuttling molecules and prefer to make thick biofilms on the electrode surface for the direct electron transfer.
3.5. Conductive polymers
Conductive polymers such as polyaniline and polypyrrole are widely used in electronics and sensors due to their good conductivity and polymer character. Recently, conductive polymers has received great attention as the bioanode materials in MFCs with outstanding performances. Polypyrrole (PPy) nanotube coated carbon paper anode delivered 6-times larger bioelectricity relative to the bare carbon paper in S. oneidensis MR-1 MFC.118 The PPy modified electrode provided high conductivity and large surface area for the improved performance of the MFC and EIS measurement revealed that the modified anode exhibited a low charge transfer resistance for the enhanced EET process. Ding et al.119 proposed PPy nano arrays (PPy-NA) as bioanode materials for an enhanced performance in MFCs over the control carbon paper, Au and ITO electrodes. The authors systematically investigated the possibility of different EET mechanisms in the presence of modified bioanode. An addition of RF in the medium with wild type Shewanella showed little effect on the EET activity suggesting that PPy-NA bioanode promoted direct electron transfer (via OM c-Cyts) from Shewanella to the electrode instead of electron shuttling by RF. Mutant experiments lacking major c-Cyt shew2525 severely impaired the EET process, indicating the role of c-Cyts in the EET mechanism. PPy acted as a conductor to transfer the metabolically generated electrons from the bacterial cells to the electrode surface. Also, as PPy was positively charged in the neutral solution, the PPy-NA electrostatically attracted the negatively charged bacterial cells to form a thick biofilm. SEM images showed that Shewanalla formed a 3D porous biofilm on the surface of PPy-NA where cells were bundled tightly by creating an electrical conduit to facilitate the EET process (Fig. 8). Moreover, the macroporous 3D biofilm allowed a free passage of the substrate (lactate) for the improved bacterial respiration. An MFC equipped with a PPy/anthraquinone-2,6-disulphonic disodium salt (PPy/AQDS)-modified anode exhibited 13-times higher current output over the unmodified electrode in the presence of S. decolorationis S12.120 CV and SEM analyses showed that the modified anode provided a large surface area for the bacterial adhesion and improved the EET process. Previous studies have already shown that AQDS can serve as an electron mediator to enhance the EET rate in G. sulfurreducens.121,122
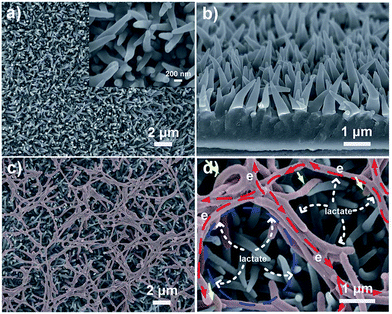 |
| Fig. 8 (a) SEM image of the as-prepared PPy-NA film showing the homogeneous distribution of PPy nanowires at a large scale. Inset: high magnification SEM image of the PPy nanowires where the conical structure is clearly seen. (b) A side-view SEM image of the as-prepared PPy-NA, suggesting its highly ordered and aligned nature. (c) Bacterial cells on a PPy-NA electrode after 60 hours of electrochemical culture at 0.2 V, exhibiting a typical porous structured bacterial network on the whole PPy-NA electrode. The microbes were labeled in red. (d) Enlarged SEM image of microbes on the PPy-NA electrode, where several microbes are bundled tightly together and are connected with neighboring microbe bundles, forming a conduit to facilitate the electron transfer (reproduced from ref. 119 with permission from Royal Society of Chemistry).119 | |
Besides PPy-NA, polyaniline nanoarrays also showed excellent performance in BESs. Ding et al.123 reported that EET in S. loihica PV-4 can be controlled with polyaniline nanoarray (PANI-NA)-bioanode by varying applied potential. Due to the presence of quinone ring in PANI, it can act as a solid electron mediator for the EET process. PANI can exist in different oxidation levels according to the applied potential, which can dictate the electron transfer to the electrode surface. At an applied potential of −0.3 V vs. Ag/AgCl, PANI stayed in reduced state and hence it was not able to mediate the anodic EET, or it blocked the electron transfer from cells to the electrode. On the other hand, at an applied potential of −0.2 V vs. Ag/AgCl, PANI stayed in the oxidized state and mediated the EET process. This observation indicated that when the applied potential became more positive, the ability of PANI as the electron mediator enhanced for the EET process. Also, the PANI-NA electrode provided large surface area for the bacterial adhesion and enabled an intimate contact with microbial nanowires. It has already been demonstrated that 3D PANI–nanowire networks formed on an ITO glass significantly enhanced the EET rate in S. loihica PV-4.124
3.6. Photo-active semiconductors
Photo-active semiconductors such as hematite (Fe2O3) were employed as the bioanode materials in MFCs.125–127 The irradiation of light to the photo-anode of MFC largely increased the photo-current in the presence of live bacterial cells. Photoexcitation of Fe2O3–modified bioanode generated electrons in the conduction band (CB) in the presence of S. loihica cells.125 Fig. 9a shows that the Fe2O3 interconnected the bacterial cells (even remote cells), resulting in a highly electrically connected bacterial networks on the electrode surface. The photo-generated electrons in the CB transferred to the electrode surface via electron hopping where the bacterial cells acted as electrical bridges by establishing long-distance electron transfer conduits. Mutant experiments lacking OM c-Cyts significantly reduced the current production, indicating the involvement of OM c-Cyts in the electron hopping process. In contrast, Qian et al.127 proposed that the photo-excited electrons in the CB of Fe2O3 directly transferred to the electrode while holes generated in the valence band (VB) accepted electrons from the OM c-Cyts of S. oneidensis cells, electron shuttles, or water (Fig. 9d).
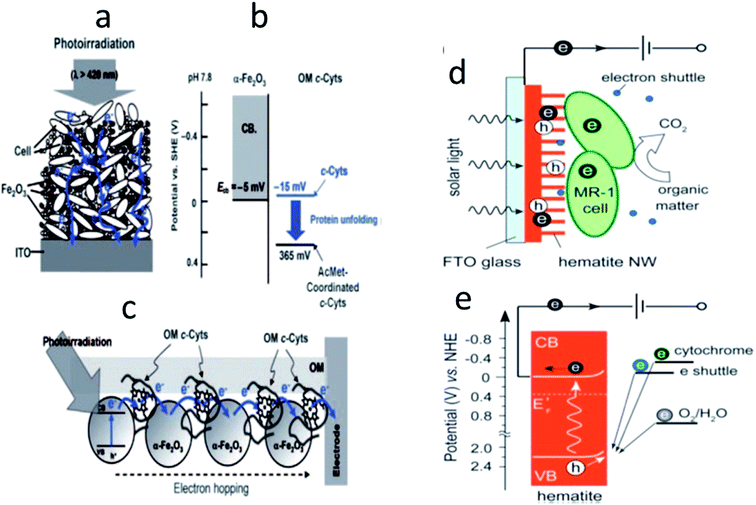 |
| Fig. 9 (a) Photocurrent generation from the bacteria/colloid network. (b) Energy levels of the outer-membrane c-Cyts in the presence of α-Fe2O3 colloids. ECB is the energy level of the conduction band edge of α-Fe2O3. (c) Proposed model for the bacterial long-distance ET process mediated by semiconductive α-Fe2O3. (d) Schematic and (e) corresponding energy diagram of the hybrid interface of the hematite photoanode in a solar microbial photoelectrochemical system. The CB, VB, and EF represent the conduction band, valence band, and Fermi level, respectively. Solid and empty circles represent electrons and holes, respectively (reproduced from ref. 125 and 127 with permission from Wiley and Sons and Royal Society of Chemistry).125,127 | |
4. Enhancement of microbial electrosynthesis process by nanostructured materials
Bacterial extracellular electron uptake from a cathode to produce chemicals is a recent research trend compared to the bacterial EET process. Even though many reports are available for the development of anode materials for the EET process, only limited studies are available for the development of efficient cathode materials for microbial electrosynthesis (MES) process.128 Uncertainty in the molecular mechanism involved in the MES process mainly hinders the progress in designing cathodes for the MES technology. Here, some recent reports on the development of biocathode materials for the MES process are discussed.
Zhang et al.129 employed various kinds of nanostructured materials to develop cathode electrodes to synthesize acetate from CO2 by Sporomusa ovata biofilms. Polyaniline–poly-acrylonitrile (PANI–PAN) microporous composite modified carbon cloth cathode exhibited 3-fold higher acetate production relative to the control. S. ovata biofilm produced 3.4-fold larger acetate production over the control with a cathode made of single walled CNTs deposited on the cotton and polyester textile fibers. Physical deposition of Au, Pd or Ni nanoparticles on a carbon cloth also enhanced the acetate production by S. ovata biofilm over the controls. Nie et al.130 developed an efficient cathode by microwave assisted deposition of Ni nanowires on a graphite surface for the improved acetate production from CO2 using bio-catalytic activity of S. ovata. The deposition of Ni nanowires on the graphite surface without microwave assistance caused the detachment of Ni wires from the graphite surface to the medium during the microbial acetate production. CV analyzes showed that Ni nanowire deposited-graphite exhibited higher cathodic current density over the untreated graphite and Ni nanoparticles coated-graphite. Also, the CV analyses excluded the possibility of involvement of electron shuttles in the microbial production of acetate. SEM images (Fig. 10) depicted thick biofilm formations on the Ni nanowire deposited-graphite surfaces while a thinner biofilm formation was observed on the Ni nanoparticle deposited-graphite surface. Here, Ni nanowires provided a large surface area and excellent conductive networks for the adhesion of S. ovata cells. Also, the negatively charged S. ovata cells131,132 electrostatically attached to the positively charged Ni nanowire deposited-graphite electrode,129 which facilitated the microbial acetate production.
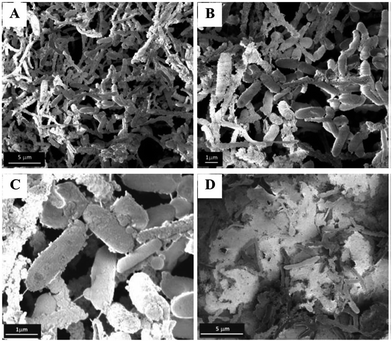 |
| Fig. 10 SEM micrographs of Sporomusa cells adhered (A–C) on nickel nanowire-coated graphite electrode and (D) on nickel nanoparticle-coated graphite (reproduced from ref. 130 with permission from Royal Society of Chemistry).130 | |
The Nanoweb-RVC electrode, which showed excellent performance as a bioanode in the MFC65 was employed as a biocathode for the microbial acetate production by mixed culture bacteria.133 The NanoWeb cathode produced 2.6-higher acetate as compared to a carbon plate electrode. The 3D structure of the NanoWeb electrode allowed thick biofilm formations in both exterior and interior sides of the electrode and the nanostructure enhanced the microbe-electrode interaction significantly. Surprisingly, acetate production was not detected with unmodified RVC and also no biofilm was observed on the bare RVC electrode surface. These observations suggested that the nanostructure is essential for both microbial acetate production and biofilm formation. Yong et al.90 constructed a biocathode by in situ reduction of non-conductive GO to conducting rGO using S. oneidensis MR-1. The rGO/Shewanaella hybrid biofilm-cathode exhibited improved reduction of fumarate to succinate. The hybrid 3D cathode provided a huge electroactive surface area for the bacterial attachment and enhanced the microbe-electrode interaction by lowering the interfacial electron transfer resistance.
5. Conclusions and future perspectives
In summary, various advanced nanostructures significantly improved both bacterial EET and microbial electrosynthesis processes. The synergetic effect of large surface area, high conductivity, and low interfacial charge resistance provided by most of the nanostructured electrodes were mainly attributed to the improved bacterial bidirectional EET process. The nanostructures developed an intimate contact with OM c-Cyts and bacterial nanowires that established an active electronic conduit for the efficient EET process. For instance, some nanomaterials such as CNTs induced cellular metabolism and even altered the electron transfer route in S. oneidensis MR-1.77 Graphene stimulated the production of phenazine, a well-known electron shuttling molecule for the EET process in Pseudomonas.84 Also, Au and Fe3O4 nanoparticles mimicked OM c-Cyts for enhancing the bacterial EET process.98,99 These findings suggest that in addition to traditional understandings on nanomaterials such as their high electrical conductivity, a molecular level understanding of how nanomaterials affect the cellular metabolism is also needed to establish strategies for the development of efficient nanomaterials to enhance the bidirectional EET process.
There is still a lot of scope for the significant enhancement in the bacterial bidirectional EET process. Currently, nanomaterial science is well-developed which can provide various advanced nanostructures as suitable candidates for the electrode development, thanks to nanotechnology. For example, anisotropic nanomaterials with exotic shapes such as prisms and octahedral can be employed to enhance the EET rate as the edges in the nanostructures may be preferable to attach the OM c-Cyts and bacterial nanowires compared to spherical nanomaterials. A very recent study revealed that hydrophilic surfaces can facilitate the EET process56 and hence application of nanostructures with super-hydrophilic surfaces may further promote the bacterial EET process. The nanostructures used for the bioanodes may also be suitable for biocathodes in the MES process as recent studies demonstrated that EET active bacteria such as Shewanella reverse their respiratory pathway for reductive metabolism by following the same EET pathway.41,134 Currently expensive materials such as CNTs, graphene, and noble metals are mostly employed for developing electrode materials and also the electrode fabrication is sometimes extremely complicated. Hence, cheaper materials with simpler fabrication techniques are needed for the bulk production of efficient electrodes for the bacterial bidirectional EET process.
Abbreviations
BES | Bioelectrochemical system |
MFC | Microbial fuel cell |
MES | Microbial electrosynthesis |
MEC | Microbial electrolysis cell |
EET | Extracellular electron transfer |
EAB | Electrochemically active bacteria |
S. oneidensis MR-1 | Shewanella oneidensis MR-1 |
S. loihica PV-4 | Shewanella loihica PV-4 |
S. putrefaciens | Shewanella putrefaciens |
S. ovata | Sporomusa ovata |
G. sulfurreducens | Geobacter sulfurreducens |
P. aeruginosa | Pseudomonas aeruginosa |
OM c-Cyts | Outer-membrane c-type cytochromes |
RF | Riboflavin |
FMN | Flavin mononucleotide |
EPS | Extracellular polymeric substance |
Si | Silicon |
ITO | Indium tin oxide |
CNT | Carbon nanotube |
MWCNT | Multi-walled carbon nanotube |
G-CNT | Graphene-carbon nanotube |
3D | Three dimensional |
EIS | Electrochemical impedance spectroscopy |
RVC | Reticulated vitreous carbon |
CV | Cyclic voltammetry |
Fe3O4 | Magnetite |
Fe2O3 | Hematite |
NB | Nitrobenzene |
GMS | Graphene modified stainless steel mesh |
CHI/VSG | Chitosan/vacuum stripped graphene scaffold |
PANI | Polyaniline |
IL-GNS | Ionic liquid functionalized graphene nano-sheets |
GO | Graphene oxide |
rGO | Reduced graphene oxide |
Au | Gold |
Pd | Palladium |
FeS | Iron sulfide |
IET | Interspecies electron transfer |
TiO2 | Titanium dioxide |
Ni | Nickel |
NiO | Nickel oxide |
PPy | Polypyrrole |
PPy-NA | Polypyrrole nano-arrays |
AQDS | Anthraquinone-2,6-disulphonic disodium salt |
CB | Conduction band |
VB | Valence band |
References
- G. Reguera, K. D. McCarthy, T. Mehta, J. S. Nicoll, M. T. Tuominen and D. R. Lovley, Nature, 2005, 435, 1098–1101 CrossRef CAS PubMed.
- D. R. Lovley, Annu. Rev. Microbiol., 2012, 66, 391–409 CrossRef CAS PubMed.
- S. J. Fuller, D. McMillan, M. B. Renz, M. Schmidt, I. T. Burke and D. I. Stewart, Appl. Environ. Microbiol., 2014, 80(1), 128–137 CrossRef CAS PubMed.
- S. Kalathil, M. M. Khan, J. Lee and M. H. Cho, Biotechnol. Adv., 2013, 31, 915–924 CrossRef CAS PubMed.
- S. Kalathil, J. Lee and M. H. Cho, Bioresour. Technol., 2012, 119, 22–27 CrossRef CAS PubMed.
- S. Kalathil, J. Lee and M. H. Cho, ChemSusChem, 2013, 6, 246–250 CrossRef CAS PubMed.
- D. Pant, G. V. Bogaert, L. Diels and K. Vanbroekhoven, Bioresour. Technol., 2010, 101, 1533–1543 CrossRef CAS PubMed.
- M. Estevez-Canales, A. Kuzume, Z. Borjas, M. Füeg, D. Lovley, T. Wandlowsk and A. Esteve-Núñez, Environ. Microbiol. Rep., 2015, 7, 219–226 CrossRef CAS PubMed.
- E. Marsili, D. B. Baron, I. D. Shikhare, J. A. Gralnick and D. R. Bond, Proc. Natl. Acad. Sci. U. S. A., 2008, 105, 3968–3973 CrossRef CAS PubMed.
- S. Pirbadian, S. E. Barchinger, K. M. Leung, H. S. Byun, Y. Jangir, R. A. Bouhenni, S. B. Reed, M. F. Romine, D. A. Saffarini, L. Shi, Y. A. Gorby, J. H. Golbeck and M. Y. EI-Naggar, Proc. Natl. Acad. Sci. U. S. A., 2014, 111, 12883–12888 CrossRef CAS PubMed.
- A. Okamoto, K. Hashimoto, K. H. Nealson and R. Nakamura, Proc. Natl. Acad. Sci. U. S. A., 2013, 110, 7856–7861 CrossRef CAS PubMed.
- A. Okamoto, S. Klathil, X. Deng, K. Hashimoto, R. Nakamura and K. H. Nealson, Sci. Rep., 2014, 4, 5628 CAS.
- S. Kalathil, K. Hashimoto and A. Okamoto, ChemElectroChem, 2014, 1, 1840–1843 CrossRef CAS.
- A. Okamoto, K. Hashimoto and K. H. Nealson, Angew. Chem., Int. Ed., 2014, 53, 10988–10991 CrossRef CAS PubMed.
- Y. A. Gorby, S. Yanina, J. S. McLean, K. M. Rosso, D. Moyles, A. Dohnalkova, T. J. Beveridge, I. S. Chang, B. H. Kim, K. S. Kim, D. E. Culley, S. B. Reed, M. F. Romine, D. A. Saffarini, E. A. Hill, L. Shi, D. A. Elias, D. W. Kennedy, G. Pinchuk, K. Watanabe, S. Ishii, B. Logan, K. H. Nealson and J. K. Fredrickson, Proc. Natl. Acad. Sci. U. S. A., 2006, 103, 11358–11363 CrossRef CAS PubMed.
- A. G. Smart, Two experiments, two takes on electric bacteria, Phys. Today, 2010, 63, 18–20 CrossRef.
- N. S. Malvankar and D. R. Lovley, ChemSusChem, 2012, 5, 1039–1046 CrossRef CAS PubMed.
- N. S. Malvankar and D. R. Lovley, Curr. Opin. Biotechnol., 2014, 27, 88–95 CrossRef CAS PubMed.
- F. Qian and Y. Li, Nat. Nanotechnol., 2011, 6, 538–539 CrossRef CAS PubMed.
- C. Hauser and S. Zhang, Nature, 2010, 468, 516–517 CrossRef CAS PubMed.
- N. S. Malvankar, M. Vargas, K. P. Nevin, A. E. Franks, C. Leang, B. H. Kim, K. Inoue, T. Mester, S. F. Covalla, J. P. Johnson, V. M. Rotello, M. T. Tuominen and D. R. Lovley, Nat. Nanotechnol., 2011, 6, 573–579 CrossRef PubMed.
- N. S. Malvankar, S. E. Yalcin, M. T. Tuominen and D. R. Lovley, Nat. Nanotechnol., 2014, 9, 1012–1017 CrossRef CAS PubMed.
- T. A. Skotheim and J. R. Reynolds, Handbook of Conducting Polymers, CRC, 2007 Search PubMed.
- K. M. Leung, G. Wanger, M. Y. El-Naggar, Y. Gorby, G. Southam, W. M. Lau and J. Yang, Nano Lett., 2013, 13, 2407–2413, DOI:10.1021/nl400237p.
- P. S. Bonanni, D. Massazza and J. P. Busalmen, Phys. Chem. Chem. Phys., 2013, 15, 10300–10306 RSC.
- N. S. Malvankar, M. T. Tuominen and D. R. Lovley, Energy Environ. Sci., 2012, 5, 6247–6249 CAS.
- D. R. Bond, S. M. Strycharz-Glaven, L. M. Tender and C. I. Torres, ChemSusChem, 2014, 5, 1099–1105 CrossRef PubMed.
- S. M. Strycharz-Glaven, R. M. Snider, A. Guiseppi-Elie and L. M. Tender, Energy Environ. Sci., 2011, 4, 4366–4379 CAS.
- S. M. Strycharz-Glaven and L. M. Tender, Energy Environ. Sci., 2012, 5, 6250–6255 CAS.
- S. Pirbadian and M. Y. El-Naggar, Phys. Chem. Chem. Phys., 2012, 14, 13802–13808 RSC.
- D. R. Lovley and N. S. Malvankar, Environ. Microbiol., 2015, 17, 2209–2215 CrossRef CAS PubMed.
- D. R. Lovley, Environ. Microbiol. Rep., 2011, 3, 27–35 CrossRef CAS PubMed.
- K. P. Nevin, T. L. Woodard, A. E. Franks, Z. M. Summers and D. R. Lovley, mBio, 2010, 1, e00103–10, DOI:10.1128/mBio.00103-10.
- K. Rabaey and R. A. Rozendal, Nat. Rev. Microbiol., 2010, 8, 706–716 CrossRef CAS PubMed.
- M. Rosenbaum, F. Aulenta, M. Villano and L. T. Angenent, Bioresour. Technol., 2011, 102, 324–333 CrossRef CAS PubMed.
- M. Sharma, N. Aryal, P. M. Sarma, K. Vanbroekhoven, B. Lal, X. Dominguez Benetton and D. Pant, Chem. Commun., 2013, 49, 6495–6497 RSC.
- S. A. Patil, S. Gildemyn, D. Pant, K. Zengler, B. E. Logan and K. Rabaey, Biotechnol. Adv., 2015, 33, 736–744 CrossRef CAS PubMed.
- L. L. Barton, F. Goulhen, M. Bruschi, N. A. Woodards, R. M. Plunkett and F. J. M. Rietmeijer, BioMetals, 2007, 20, 291–302 CrossRef CAS PubMed.
- M. Guiral-Brugna, M. T. Giudici-Orticoni, M. Bruschi and P. Bianco, J. Electroanal. Chem., 2001, 510, 136–143, DOI:10.1016/s0022-0728(01)00502-2.
- A. S. Pereira, R. Franco, M. J. Feio, C. Pinto, J. Lampreia, M. A. Reis, J. Calvete, I. Moura, I. Beech, A. R. Lino and J. J. G. Moura, Biochem. Biophys. Res. Commun., 1996, 221, 414–421 CrossRef CAS PubMed.
- D. E. Ross, J. M. Flynn, D. B. Baron, J. A. Gralnick and D. R. Bond, PLoS One, 2011, 6, e16649 CAS.
- N. S. Malvankar, M. T. Tuominen and D. R. Lovley, Energy Environ. Sci., 2012, 5, 5790–5797 CAS.
- R. M. Donlan, Emerging Infect. Dis., 2002, 8, 881–890 CrossRef PubMed.
- V. B. Trans, S. J. M. Fleiszig, D. J. Evans and C. Y. Radke, Appl. Environ. Microbiol., 2011, 77, 3644–3652 CrossRef PubMed.
- B. Li and B. E. Logan, Colloids Surf., B, 2004, 36, 81–90 CrossRef CAS PubMed.
- C. H. Bell, B. S. Arora and T. A. Camesano, Environ. Eng. Sci., 2005, 22, 629–641 CrossRef CAS.
- P. Stoodley, K. Sauer, D. G. Davies and J. W. Costerton, Annu. Rev. Microbiol., 2002, 56, 187–2009 CrossRef CAS PubMed.
- E. K. Eboigbodin, A. R. J. Newton, F. A. Routh and A. C. Biggs, Langmuir, 2005, 21, 12315–12319 CrossRef PubMed.
- Y.-W. Chang, A. A. Fragkopoulos, S. M. Marquez, H. D. Kim, T. E. Angelini and A. Fernández-Nieves, New J. Phys., 2015, 17, 033017 CrossRef.
- J. H. Felix and C. Dekker, Science, 2014, 346, 1251821–1251829 CrossRef PubMed.
- A. I. Hochbaum and J. Aizenberg, Nano Lett., 2010, 10, 3717–3721 CrossRef CAS PubMed.
- K. K. Sakimoto, C. Liu, J. Lim and P. Yang, Nano Lett., 2014, 14, 5471–5476 CrossRef CAS PubMed.
- H. E. Jeong, I. Kim, P. Karam, H.-J. Choi and P. Yang, Nano Lett., 2013, 13, 2864–2869 CrossRef CAS PubMed.
- A. V. Singh, V. Vyas, R. Patil, V. Sharma, P. E. Scopelliti, G. Bongiorno, A. Podesta, C. Lenardi, W. N. Gade and P. Milani, PLoS One, 2011, 6, e25029 CAS.
- N. Mitik-Dineva, J. Wang, R. C. Mocanasu, P. R. Stoddart, R. J. Crawford and E. P. Ivanova, Biotechnol. J., 2008, 3, 536–544 CrossRef CAS PubMed.
- C.-M. Ding, M.-I. Lv, Y. Zhu, L. Jiang and H. Liu, Angew. Chem., Int. Ed., 2015, 54, 1446–1451 CrossRef CAS PubMed.
- M. Zhous, M. Chi, J. Luo, H. He and T. Jin, J. Power Sources, 2011, 196, 4427–4435, DOI:10.1016/j.jpowsour.2011.01.012.
- J. Wei, P. Liang and X. Huang, Bioresour. Technol., 2011, 102, 9335–9344 CrossRef CAS PubMed.
- Y. Alvarez-Gallego, X. Dominguez-Benetton, D. Pant, L. Diels, K. Vanbroekhoven, I. Genne and P. Vermeiren, Electrochim. Acta, 2012, 82, 415–426 CrossRef CAS.
- X. Zhang, D. Pant, F. Zhang, J. Liu, W. He and B. E. Logan, ChemElectroChem, 2014, 1, 1859–1866 CrossRef CAS.
- M. Mustakeem, Materials for Renewable and Sustainable Energy, 2015, 4, 22 CrossRef.
- X. Xie, L. Hu, M. Pasta, G. F. Wells, D. Kong, C. S. Criddle and Y. Cui, Nano Lett., 2011, 11, 291–296 CrossRef CAS PubMed.
- X. Xie, M. Ye, L. Hu, N. Liu, J. R. McDonough, W. Chen, H. N. Alshareef, C. S. Criddle and Y. Cui, Energy Environ. Sci., 2012, 5, 5265–5270 CAS.
- X. Xie, W. Zhao, H. R. Lee, C. Liu, M. Ye, W. Xie, B. Cui, C. S. Criddle and Y. Cui, ACS Nano, 2014, 8, 11958–11965 CrossRef CAS PubMed.
- V. Flexer, J. Chen, B. C. Donose, P. Sherrell, G. G. Wallace and J. Keller, Energy Environ. Sci., 2013, 6, 1291–1298 CAS.
- I. H. Park, M. Christy, P. Kim and K. S. Nahm, Biosens. Bioelectron., 2014, 58, 75–80 CrossRef CAS PubMed.
- J. E. Mink, J. P. Rojas, B. E. Logan and M. M. Hussain, Nano Lett., 2012, 12, 791–795 CrossRef CAS PubMed.
- J. E. Mink and M. M. Hussain, ACS Nano, 2013, 7, 6921–6927 CrossRef CAS PubMed.
- K. Katuri, M. L. Ferrer, M. C. Gutièrrez, R. Jimènez, F. D. Monte and D. Leech, Energy Environ. Sci., 2011, 4, 4201–4210 CAS.
- X.-W. Liu, J.-J. Chen, Y.-X. Huang, X.-F. Sun, G.-P. Sheng, D.-B. Li, L. Xiong, Y.-Y. Zhang, F. Zhao and H. Q. Yu, Sci. Rep., 2014, 4, 3732 Search PubMed.
- L. Peng, S.-J. You and J.-Y. Wang, Biosens. Bioelectron., 2010, 25, 1248–1251 CrossRef CAS PubMed.
- X.-W. Liu, Y.-X. Huang, X.-F. Sun, G.-P. Sheng, F. Zhao, S.-G. Wang and H.-Q. Yu, ACS Appl. Mater. Interfaces, 2014, 6, 8158–8164 CAS.
- S. Kalathil, N. V. Hoa, J.-J. Shim, M. M. Khan, J. Lee and M. H. Cho, J. Nanosci. Nanotechnol., 2013, 13, 7712–7716 CrossRef CAS PubMed.
- X. Zhang, M. Epifanio and E. Marsili, Electrochim. Acta, 2013, 102, 252–258, DOI:10.1016/j.electacta.2013.04.039.
- D. R. Bond, S. M. Strycharz-Glaven, L. M. Tender and C. I. Torres, ChemSusChem, 2012, 5, 1099–1105 CrossRef CAS PubMed.
- C. Erbay, X. Pu, W. Choi, M.-J. Choi, Y. Ryu, H. Hou, F. Lin, P.-D. Figueiredo, C. Yu and A. Han, J. Power Sources, 2015, 280, 347–354, DOI:10.1016/j.jpowsour.2015.01.065.
- F.-F. Yan, Y.-R. HE, C. Wu, Y.-Y. Cheng, W.-W. Li and H.-Q. Yu, Environ. Sci. Technol., 2014, 1, 128–132 CAS.
- S. Matsumoto, K. Yamanaka, H. Ogikubo, H. Akasaka and N. Ohtake, Appl. Phys. Lett., 2014, 105, 083904–4, DOI:10.1063/1.4894259.
- Y. Zhang, G. Mo, X. Li, W. Zhang, J. Zhang, J. Ye, X. Huang and C. Yu, J. Power Sources, 2011, 196, 5402–5407 CrossRef CAS.
- W. Chen, Y.-X. Huang, D.-B. Li, H.-Q. Yu and L. Yan, RSC Adv., 2014, 4, 21619–21624 RSC.
- K. E. Mink, R. M. Qaisi and M. M. Hussain, Energy Technol., 2013, 1, 648–652 CrossRef.
- D. M. Karabacak, S. H. Brongersma and M. Crego-Calama, Lab Chip, 2010, 10, 1976–1982 RSC.
- Z. He, J. Liu, Y. Qiao, C. M. Li and T. T. Yang Tan, Nano Lett., 2012, 12, 4738–4741 CrossRef CAS PubMed.
- J. Liu, Y. Qiao, C. X. Guo, S. Lim, H. Song and C. M. Li, Bioresour. Technol., 2012, 114, 275–280 CrossRef CAS PubMed.
- Y.-C. Yong, X.-C. Dong, M. B. Chan-Park, H. Song and P. Chen, ACS Nano, 2012, 6, 2394–2400 CrossRef CAS PubMed.
- B. Lai, X. Tang, H. Li, Z. Du, X. Liu and Q. Zhang, Biosens. Bioelectron., 2011, 28, 373–377, DOI:10.1016/j.bios.2011.07.050.
- C. Zhao, Y. Wang, F. Shi, J. Zhang and J.-J. Zhu, Chem. Commun., 2013, 49, 6668–6670 RSC.
- Y.-X. Huang, X.-W. Liu, J.-F. Xie, G.-P. Sheng, G.-Y. Wang, Y.-Y. Zhang, A.-W. Xu and H.-Q. Yu, Chem. Commun., 2011, 47, 5795–5797 RSC.
- H. Wang, G. Wang, Y. Ling, F. Qian, Y. Song, X. Lu, S. Chen, Y. Tong and Y. Li, Nanoscale, 2013, 5, 10283–10290 RSC.
- Y.-C. Yong, Y.-Y. Yu, X. Zhang and H. Song, Angew. Chem., Int. Ed., 2014, 53, 4480–4483 CrossRef CAS PubMed.
- Y. Yuan, S. Zhou, B. Zhao, L. Zhuang and Y. Wang, Bioresour. Technol., 2012, 116, 453–458 CrossRef CAS PubMed.
- C.-E. Zhao, J. Wu, Y. Ding, V. B. Wang, Y. Zhang, S. Kjelleberg, J. S. Chye Loo, B. Cao and Q. Zhang, ChemElectroChem, 2015, 2, 654–658 CrossRef CAS.
- Y. Fan, S. Xu, R. Schaller, J. Jiao, F. Chaplen and H. Liu, Biosens. Bioelectron., 2011, 26, 1908–1912 CrossRef CAS PubMed.
- F. A. Alatraktchi, Y. Zhang and I. Angelidaki, Appl. Energy, 2014, 116, 216–222 CrossRef CAS.
- F. A. Alatraktchi, Y. Zhang, J. S. Noori and I. Angelidaki, Bioresour. Technol., 2012, 123, 177–183 CrossRef CAS PubMed.
- B. Maestro, J. M. Ortiz, G. Schrott, J. P. Busalmen, V. Climent and J. M. Feliu, Bioelectrochemistry, 2014, 98, 11–19 CrossRef CAS PubMed.
- X. Wu, F. Zhao, L. Rahunen, J. R. Varcoe, C. Avignone-Rossa, A. E. Thumser and R. C. T. Slade, Angew. Chem., Int. Ed., 2011, 50, 427–430 CrossRef CAS PubMed.
- R. Wu, L. Cui, L. Chen, C. Wang, C. Cao, G. Sheng, H. Yu and F. Zhao, Sci. Rep., 2013, 3, 3307 Search PubMed.
- F. Liu, A.-E. Rotaru, P. M. Shrestha, N. S. Malvankar, K. P. Nevin and D. R. Lovley, Environ. Microbiol., 2015, 17, 648–655 CrossRef CAS PubMed.
- Y. Xiao, F. Patolsky, E. Katz, J. F. Hainfeld and I. Willner, Science, 2003, 299, 1877–1881 CrossRef CAS PubMed.
- T. M. Flynn, E. J. O'Loughlin, B. Mishra, T. J. DiChristina and K. M. Kemner, Science, 2014, 344, 1039–1042 CrossRef CAS PubMed.
- R. Nakamura, A. Okamoto, N. Tajima, G. J. Newton, F. Kai, T. Takashima and K. Hashimoto, ChemBioChem, 2010, 11, 643–645 CrossRef CAS PubMed.
- X. Jiang, J. Hu, A. M. Lieber, C. S. Jackan, J. C. Biffinger, L. A. Fitzgerald, B. R. Ringeisen and C. M. Lieber, Nano Lett., 2014, 14, 6737–6742 CrossRef CAS PubMed.
- S. Kato, R. Nakamura, F. Kai, K. Watanabe and K. Hashimoto, Environ. Microbiol., 2010, 12, 3114–3123 CrossRef CAS PubMed.
- S. Kato, K. Hashimoto and K. Watanabe, Environ. Microbiol., 2012, 14, 1646–1654 CrossRef CAS PubMed.
- S. Kato, K. Hashimoto and K. Watanabe, Proc. Natl. Acad. Sci. U. S. A., 2012, 109, 10042–10046 CrossRef CAS PubMed.
- X. Peng, H. Yu, L. Ai, N. Li and X. Wang, Bioresour. Technol., 2013, 144, 689–692 CrossRef CAS PubMed.
- L. Wang, L. Su, H. Chen, T. Yin, Z. Lin, X. Lin, C. Yuan and D. Fu, Mater. Lett., 2015, 141, 311–314, DOI:10.1016/j.matlet.2014.11.121.
- J. Tang, Y. Yuan, T. Liu and S. Zhou, J. Power Sources, 2015, 274170–274176, DOI:10.1016/j.jpowsour.2014.10.035.
- Z. Wen, S. Ci, S. Mao, S. Cui, G. Lu, K. Yu, S. Luo, Z. He and J. Chen, J. Power Sources, 2013, 234, 100–106 CrossRef CAS.
- Y. Qiao, S.-J. Bao, C. M. Li, X.-Q. Cui, Z.-S. Lu and J. Guo, ACS Nano, 2008, 2, 113–119, DOI:10.1021/nn700102s.
- T. Yin, Z. Lin, L. Su, C. Yuan and D. Fu, ACS Appl. Mater. Interfaces, 2015, 7(1), 400–408, DOI:10.1021/am506360x.
- Y. Qiao, X.-S. Wu and C. M. Li, J. Power Sources, 2014, 266, 226–231, DOI:10.1016/j.jpowsour.2014.05.015.
- J. N. Roy, S. Babanova, K. E. Garcia, J. Cornejo, L. K. Ista and P. Atanassov, Electrochim. Acta, 2014, 126, 3–10, DOI:10.1016/j.electacta.2013.07.075.
- S. T. Read, P. Dutta, P. L. Bond, J. Keller and K. Rabaey, BMC Microbiol., 2010, 10, 98 CrossRef PubMed.
- F. Zhang, S.-J. Yuan, W.-W. Li, J.-J. Chen, C.-C. Ko and H.-Q. Yu, Electrochim. Acta, 2015, 152, 1–5 CrossRef CAS.
- X. Jiang, J. Hu, L. A. Fitzgerald, J. C. Biffinger, P. Xie, B. R. Ringeisen and C. M. Lieber, Proc. Natl. Acad. Sci. U. S. A., 2010, 107, 16806–16810 CrossRef CAS PubMed.
- C.-sssssssssE. Zhao, J. Wu, S. Kjelleberg, J. S. Chey Loo and Q. Zhang, Small, 2015, 11, 3440–3443 CrossRef CAS PubMed.
- C. Ding, H. Liu, M. Lv, T. Zhao, Y. Zhu and L. Jiang, Nanoscale, 2014, 6, 7866–7871 RSC.
- C. Feng, L. Ma, F. Li, H. Mai, X. Lang and S. Fan, Biosens. Bioelectron., 2010, 25, 1516–1520, DOI:10.1016/j.bios.2009.10.009.
- M. Adachi, T. Shimomura, M. Komatsu, H. Yakuwa and A. Miya, Chem. Commun., 2008, 2055–2057 RSC.
- O. Schaetzle, F. Barrière and K. Baronian, Energy Environ. Sci., 2008, 1, 607–620 CAS.
- C. Ding, H. Liu, Y. Zhu, M. Wan and L. Jiang, Energy Environ. Sci., 2012, 5, 8517–8522 CAS.
- Y. Zhao, K. Watanabe, R. Nakamura, S. Mori, H. Liu, K. Ishii and K. Hashimoto, Chem.–Eur. J., 2010, 16, 4982–4985 CrossRef CAS PubMed.
- R. Nakamura, F. Kai, A. Okamoto, G. J. Newton and K. Hashimoto, Angew. Chem., Int. Ed., 2009, 48, 508–511 CrossRef CAS PubMed.
- R. Nakamura, F. Kai, A. Okamoto and K. Hashimoto, J. Mater. Chem. A, 2013, 1, 5148–5157 CAS.
- F. Qian, H. Wang, Y. Ling, G. Wang, M. P. Thelen and Y. Li, Nano Lett., 2014, 14, 3688–3693 CrossRef CAS PubMed.
- S. Bajracharya, A. T. Heijne, X. Dominguez Benetton, K. Vanbroekhoven, C. J. N. Buisman, D. P. B. T. B. Strik and D. Pant, Bioresour. Technol., 2015, 195, 14–24 CrossRef CAS PubMed.
- T. Zhang, H. Nie, T. S. Bain, H. Lu, M. Cui, O.-L. Snoeyenbos-West, A. E. Franks, K. P. Nevin, T. P. Russell and D. R. Lovley, Energy Environ. Sci., 2013, 6, 217–224 CAS.
- H. Nie, T. Zhang, M. Cui, H. Lu, D. R. Lovley and T. P. Russell, Phys. Chem. Chem. Phys., 2013, 15, 14290–14294 RSC.
- T. J. Beveridge, J. Bacteriol., 1999, 181, 4725–4733 CAS.
- M. Sára and U. B. Sleytr, J. Bacteriol., 2000, 182, 859–868 CrossRef.
- L. Jourdin, S. Freguia, B. C. Donose, J. Chen, G. G. Wallace, J. Keller and V. Flexer, J. Mater. Chem. A, 2014, 2, 13093–13102 CAS.
- A. Okamoto, K. Hashimoto and K. H. Nealson, Angew. Chem., 2014, 126, 11168–11171, DOI:10.1002/ange.201407004.
|
This journal is © The Royal Society of Chemistry 2016 |