DOI:
10.1039/C6RA04733E
(Paper)
RSC Adv., 2016,
6, 32722-32726
p-tert-Butylthiacalix[4]arenes equipped with guanidinium fragments: aggregation, cytotoxicity, and DNA binding abilities†
Received
22nd February 2016
, Accepted 16th March 2016
First published on 21st March 2016
Abstract
Mono-, di- and tetracationic thiacalix[4]arenes in a 1,3-alternate conformation functionalized with guanidinium groups showed a strong dependence of the aggregation properties with the ratio of guanidinium/n-decyl fragments attached to phenolic groups. Increasing the amount of guanidinium fragments improved the macrocycles solubility in water as well as the sorption capacity towards polynucleotide molecules. The synthesized thiacalixarenes showed relatively high toxicity comparable with that for similar receptors based on the classical calixarene.
Introduction
The molecular design of synthetic receptors that can effectively recognize anionic and polyanionic substrates is an important task of supramolecular chemistry.1 Growing interest in anion recognition is caused by wide spreading of anionic “guests” in biological systems: DNA, RNA, most enzyme substrates, and cofactors are anionic molecules.2
On the other hand, design of synthetic receptors for anionic and polyanionic substrates is a challenge due to the wide variety of their geometric forms, sensitivity to pH values, and strong solvation in polar media.3 Applying various types of colloid receptors, including cationic lipids, polymers, dendrimers, and peptides, which are able to effectively interact with polyanionic surfaces of biomacromolecules, is one of the most promising approaches in supramolecular chemistry.4–8 In this regard design of preorganized receptors, which can be assembled into nanosized colloid structures, may help in solving this complex task.
Among all types of functional groups applied in receptors for polyanionic substrates, the guanidinium fragment provides the greatest affinity9 due to geometrical and charge complementarity to carboxylate and phosphate groups (Fig. 1).1
 |
| Fig. 1 The complementarity of the guanidinium moiety to a carboxylate and phosphate groups. | |
Applying molecular platforms like calixarenes and thiacalixarenes allows to construct molecules that combine different types of functional groups to adjust the affinity of colloid receptors towards anionic and polyanionic substrates.10,11 In p-tert-butylthiacalix[4]arene, the bond length between the aromatic residue and bridging group is 15% larger than that in methylene bridged p-tert-butylcalix[4]arene,12,13 which allows for the design of conformationally more flexible receptors compared with conventional calix[4]arene14,15 for more effective recognition of biological anionic substrates.16
Herein, we continue to develop our approach towards synthesis of preorganized guanidinium receptors based on stepwise functionalization of the lower rim of the p-tert-butylthiacalix[4]arene platform.17,18 We studied aggregation and cytotoxicity of cationic thiacalixarenes functionalized with guanidinium fragments, as well as their binding affinity towards polynucleotide molecules.
Results and discussion
The three cationic thiacalix[4]arenes in 1,3-alternate conformation, differing in the ratio of guanidinium/n-decyl fragments attached to phenolic groups, were included in this study (Fig. 2). Synthesis of the target compounds 1–3 based on differences in the reactivity of N-(3-bromopropyl)phthalimide and N-(2-bromoethyl)phthalimide during interaction with p-tert-butylthiacalix[4]arene in the presence of alkali metal carbonates.19 The synthesis of compounds 1 and 2 was carried out in our previous studies.17,18 Scheme 1 shows the synthetic route to compound 3.
 |
| Fig. 2 Molecular structures of compounds 1–3. | |
 |
| Scheme 1 Synthetic route to 3. | |
Compound 1 is water-soluble and forms nanoaggregates, which dissociate during interaction with DNA.17 Solubilization of amphiphilic compound 2 via formation of solid lipid nanoparticles (SLNs) was described earlier16 so the same nanoprecipitation technique was used for water insoluble compound 3.20–22 The size and morphology of the obtained SLNs were characterized by scanning electron microscopy (SEM), and the results are presented in Fig. 3. Fig. 3A shows that 2-based SLNs were localized on the surface as raspberry-like aggregates with an average 102 nm diameter. The average diameter of 3-based SLNs was 94 nm. The surfaces of 3-based SLNs were much smoother (Fig. 3B) compared with aggregates based on compound 2, so it is assumed that the 3-based SLNs tried to minimize their surface area and were not as stable as 2. This assumption was proven by further self-precipitation of 3-based SLNs by a slow precipitation procedure and hence all further studies were performed with compounds 1 and 2. The aggregation behaviour of compounds 1–3 is summarized in Fig. 4.
 |
| Fig. 3 SEM images of 2-based (A) and 3-based (B) SLNs (scale bars are 200 nm). | |
 |
| Fig. 4 Aggregation behaviour of synthesized compounds 1–3. | |
The differences in aggregation behaviour of compounds 1–3 is attributed to the differences in their structures. Stepwise replacement of hydrophilic 3-guanidiniumpropyl fragments in compound 1 to hydrophobic n-decyl groups led to insolubility of compound 2 and 3 in water. Moreover, the presence of three n-decyl groups in compound 3 resulted in low stability of 3-based SLNs compared with 2-based SLNs.
Interaction between aggregates based on compound 1 and 2 with polynucleotide (phMGFP) was examined by the gel electrophoresis method (Fig. 5). The gel analysis showed the total binding of pDNA occurs at up to 15.4 and 1060 μM for compounds 1 and 2, respectively. Thus macrocycle 1 possesses higher DNA sorption capacity than 2 (69 times); we associate this with the difference in the number of charged guanidinium fragments, and there being a significant amount of compound 2 located inside the SLNs that is not able to interact with polynucleotides.
 |
| Fig. 5 Agarose gel electrophoresis of pDNA (25 μg mL−1) incubated with increasing amounts of compounds 1 (top) and 2 (bottom). [Values are expressed in μM, P – pure plasmid DNA, GR – GeneRuler 1 kb DNA Ladder]. | |
To assess in vitro cytotoxicity of compounds 1 and 2, increasing amounts of them were incubated with samples of three different cell lines: CV-1 (monkey kidney cell line), saiga kidney cell line (SK) and L-mouse fibroblast cell line (MF). Table 1 shows the obtained results.
Table 1 IC50 and IC100 values (μM) of compounds 1 and 2 on cell viability
|
1 |
2 |
IC50 |
IC100 |
IC50 |
IC100 |
CV-1 |
1.2 |
2.5 |
1.8 |
7.4 |
SK |
1.2 |
5.0 |
3.7 |
7.4 |
MF |
0.6 |
2.5 |
1.8 |
7.4 |
It turns out that despite the significant difference in DNA sorption capacity, the compounds possessed similar cytotoxicity toward the chosen cell lines.
The cytotoxicity of synthesized compounds 1 and 2 was compared with the cytotoxicity of previously described calix[4]arene derivatives 4–8 (Fig. 6).23 Table 2 groups the IC50 values of compounds 4–8 for tumor cell lines.
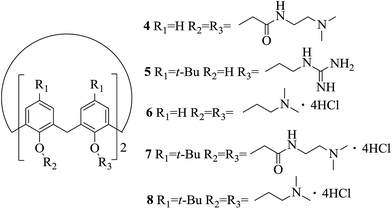 |
| Fig. 6 Molecular structures of compounds 4–8 based on calix[4]arene. | |
Table 2 IC50 values (μM) of compounds 4–8 on a tumor cells viability23
|
4 |
5 |
6 |
7 |
8 |
HUVEC |
3 |
3 |
2 |
8 |
2 |
2H11 |
>100 |
80 |
0.7 |
15 |
4 |
Fibroblasts |
35 |
5 |
0.2 |
6 |
1 |
FSAII |
>20 |
10 |
0.7 |
10 |
2 |
MA148 |
0.5 |
40 |
1.5 |
15 |
3 |
A549 |
2 |
8 |
0.8 |
6 |
1 |
SCK |
100 |
4 |
0.7 |
9 |
0.7 |
B16F10 |
80 |
100 |
1 |
8 |
2 |
It should be kept in mind the direct comparison of the IC50 values of our compounds and compounds listed in Table 2 is not strictly correct due to the different nature of the cell lines. However, as a first-order approximation it can be concluded that synthesized macrocycles 1 and 2 possess similar cytotoxicity as the most toxic compounds 6 and 8, based on the calixarene platform.
Conclusions
This study showed aggregation behaviour of synthesized thiacalix[4]arenes functionalized with guanidinium groups strongly depended on the ratio of hydrophilic/hydrophobic fragments in the receptor structures. Increasing the amount of guanidinium fragments improved the receptor solubility in water as well as their sorption capacity towards polynucleotide molecules. The in vitro cytotoxicity assay showed high toxicity of synthesized compounds comparable with that for similar receptors based on the classical calixarene.
Experimental
General
Plasmid DNA phMGFP was purchased from Promega. Cell cultures (CV-1 (monkey kidney cell line), saiga kidney cell line and L-mouse fibroblast cell line) were taken from the collection of State Science Institution National Research Institute of Veterinary Virology and Microbiology of the Russian Academy of Agricultural Sciences. N,N′-bis(tert-butoxycarbonyl)-N′′-triflyl-guanidine was obtained as described.24
NMR spectroscopy
The 1H, 13C, 2D 1H–1H NOESY NMR spectra were obtained on a Bruker Avance-400 spectrometer. Chemical shifts were determined relative to the signals of residual protons of the deuterated solvent (CDCl3).
FT-IR spectroscopy
IR spectra were obtained using a Fourier Transform Spectrum 400 IR spectrometer (Perkin Elmer).
Elemental analysis
Elemental analysis was performed with a Perkin Elmer 2400 Series II instrument.
MALDI MS
Mass spectra were obtained with the MALDI-TOF Dynamo Finnigan mass analyzer using p-nitroaniline as a matrix.
Synthesis of 5,11,17,23-tetra-tert-butyl-25,26,27-tridecyl-28-[2-phthalimidoethoxy]-2,8,14,20-tetrathiacalix[4]arene (1,3-alternate) (3b)
In a round bottomed flask equipped with a magnetic stirrer and reflux condenser, a mixture of 1.50 g (1.14 mmol) of the compound 3a, 1.51 g (6.84 mmol) 1-bromodecane, 2.23 g (6.84 mmol) of freshly powdered cesium carbonate and 60 mL of acetone was refluxed for 48 hours. After cooling the reaction mixture, the precipitate was filtered off, the solvent from the filtrate was distilled off under reduced pressure and the residue was recrystallized from methanol. Yield 82%. Found: C, 72.81; H, 8.51; N, 1.05; S, 9.64. C80H115NO6S4 requires C, 73.07; H, 8.81; N, 1.07; S, 9.75. MS (MALDI-TOF): calculated [M+] m/z = 1313.8, found [M + H]+ m/z = 1314.6, [M + Na]+ m/z = 1336.5, [M + K]+ m/z = 1352.6. νmax/cm−1 1267 (COC); 1715, 1775 (C
O) δH (400 MHz; CDCl3): 0.80–1.37 (57H, br.m, (CH2)8CH3), 1.28 (9H, s, (CH3)3C), 1.29 (9H, s, (CH3)3C), 1.34 (18H, s, (CH3)3C), 3.60 (2H, m, CH2N), 3.86 (6H, m, CH2O), 4.13 (2H, m, CH2O), 7.32 (2H, s, ArH), 7.37 (4H, m, ArH), 7.70 (2H, d, J = 2.4 Hz, ArH), 7.73 (2H, m, Pht), 7.88 (2H, m, Pht). δC (125 MHz; CDCl3): 14.2, 14.3, 22.8, 22.9, 25.94, 25.97, 28.8, 29.1, 29.4, 29.5, 29.69, 29.73, 29.8, 29.9, 30.0, 31.5, 32.0, 32.1, 34.34, 34.37, 34.5, 36.4, 64.4, 68.5, 69.0, 123.4, 127.6, 128.1, 128.2, 128.47, 128.50, 128.52, 128.7, 132.3, 134.0, 145.4, 146.0, 157.0, 157.2, 167.9. Spectrum 1H–1H NOESY (the most important cross-peaks): H4b/H7′, H4+b/H7′.
Synthesis of 5,11,17,23-tetra-tert-butyl-25,26,27-tridecyl-28-[2-aminoethoxy]-2,8,14,20-tetrathiacalix[4]arene (3c)
A mixture of 1.00 g of compound 3b and 1 mL (20 mmol) of hydrazine hydrate was refluxed in a 30 mL THF and 30 mL ethanol mixture for 20 h. Then, the solvent was evaporated, 60 mL of water was added and white powder was filtered off. The obtained powder was dried in a desiccator under reduced pressure. Yield 93%. Found: C, 72.62; H, 9.51; N, 1.11; S, 10.64. C72H113NO4S4 requires C, 72.98; H, 9.61; N, 1.18; S, 10.82. MS (MALDI-TOF): calculated [M+] m/z = 1183.8, found [M + H]+ m/z = 1184.6. νmax/cm−1 1266 (COC); 3362 (NH2). δH (400 MHz; CDCl3): 0.85–1.35 (57H, br.m, (CH2)8CH3), 1.28 (18H, s, (CH3)3C), 1.29 (18H, s, (CH3)3C), 2.45 (2H, t, J = 5.1 Hz, CH2N), 3.82 (4H, m, CH2O), 3.90 (2H, m, CH2O), 3.95 (2H, t, J = 5.0 Hz, CH2O), 7.32 (2H, d, J = 2.5 Hz, ArH), 7.35 (2H, d, J = 2.4 Hz, ArH), 7.35 (2H, s, ArH), 7.36 (2H, s, ArH). δC (125 MHz; CDCl3): 14.3, 22.8, 25.8, 25.9, 28.9, 29.2, 29.5, 29.7, 29.8, 29.9, 30.0, 30.2, 31.47, 31.49, 31.51, 32.1, 34.35, 34.38, 34.45, 68.8, 127.1, 127.6, 127.8, 128.0, 128.3, 128.7, 146.0, 157.0.
Synthesis of 5,11,17,23-tetra-tert-butyl-25,26,27-tridecyl-28-[2-(bis-tert-butoxycarbonyl-guanidine)ethoxy]-2,8,14,20-tetrathiacalix[4]arene (1,3-alternate) (3d)
A stoichiometric amount of N,N′-di-(tert-butoxycarbonyl)-N′′-triflyl guanidine in 20 mL of dichloromethane was added to the ice cooled solution of 1.00 g of compound 3c in 40 mL of dichloromethane. After 24 hours, the mixture was washed with 2 M aqueous sodium bisulfate (10 mL) and saturated sodium bicarbonate (10 mL). Each aqueous layer was extracted with dichloromethane (2 × 10 mL). The combined organic phases were washed with brine (10 mL), dried by molecular sieves 3 Å and then the dichloromethane was evaporated under reduced pressure. Obtained white powder was dried in a desiccator under reduced pressure. Yield 61%. Found: C, 69.71; H, 9.13; N, 2.81; S, 8.82. C83H131N3O8S4 requires C, 69.85; H, 9.25; N, 2.94; S, 8.99. MS (MALDI-TOF): calculated [M+] m/z = 1425.88, found [M − 2Boc + H]+ m/z = 1227.8. νmax/cm−1 1263 (COC); 1637 (N–CO); 1616, 1637 (C
N); 1718 (C
O) and 3332 (NH). δH (400 MHz; CDCl3): 0.85–1.33 (57H, br.m, (CH2)8CH3), 1.26 (9H, s, (CH3)3C), 1.27 (9H, s, (CH3)3C), 1.27 (18H, s, (CH3)3C), 1.49 (9H, s, Boc), 1.50 (9H, s, Boc), 3.10 (2H, m, CH2N), 3.84 (6H, m, CH2O), 4.05 (2H, t, J = 7.0 Hz, CH2O), 7.31 (2H, s, ArH), 7.32 (2H, d, J = 2.4 Hz, ArH), 7.34 (2H, s, ArH), 7.57 (2H, d, J = 2.4 Hz, ArH), 8.30 (1H, t, J = 5.6 Hz, NHCH2), 11.37 (1H, s, NHBoc). δC (125 MHz; CDCl3): 14.3, 22.9, 25.9, 26.0, 28.2, 28.5, 29.1, 29.2, 29.4, 29.5, 29.7, 29.8, 29.9, 30.0, 30.1, 31.48, 31.53, 32.0, 32.1, 34.3, 34.4, 40.1, 66.6, 68.9, 69.2, 78.8, 82.9, 128.1, 128.18, 128.20, 128.3, 128.58, 128.61, 128.9, 145.4, 145.5, 145.8, 153.1, 156.2, 156.7, 157.2, 157.4, 163.8. Spectrum 1H–1H NOESY (the most important cross-peaks): H4′b/HBoc, H3′/HBoc.
Synthesis of 5,11,17,23-tetra-tert-butyl-25,26,27-tridecyl-28-[2-guanidiniumethoxy]-2,8,14,20-tetrathiacalix[4]arene chloride (1,3-alternate) (3)
2 mL of concentrated hydrochloric acid were added to a solution of 0.50 g of the compound 3d in 40 mL of tetrahydrofuran. The reaction mixture was stirred for 24 hours. Then, the solvent was evaporated under vacuum and 40 mL of water was added to the reaction mixture. The precipitate was filtered off and washed with water. The obtained white powder was dried in a desiccator under reduced pressure. Yield 65%. Found: C, 68.86; H, 9.16; N, 3.23; S, 9.86. C73H116ClN3O4S4. requires C, 69.40; H, 9.25; N, 3.30; S, 10.15. MS (MALDI-TOF): calculated [M+] m/z = 1261.8, found [M − Cl]+ m/z = 1226.8. νmax/cm−1 1265 (COC); 1663 (C
N); 3333 (NH). δH (400 MHz; CDCl3): 0.85–1.67 (57H, br.m, (CH2)8CH3), 1.28 (36H, s, (CH3)3C), 3.40 (2H, m, CH2N), 3.77 (4H, br.t, J = 8.5 Hz, CH2O), 4.05 (2H, br.t, J = 7.6 Hz, CH2O), 4.14 (2H, br.m, CH2O), 7.34 (4H, s, ArH), 7.40 (2H, s, ArH), 7.50 (2H, s, ArH), 8.93 (1H, br.m, NHCH2). δC (125 MHz; CDCl3): 14.3, 22.9, 25.83, 25.90, 28.6, 29.4, 29.6, 26.72, 29.76, 30.2, 31.4, 31.5, 32.0, 34.4, 34.7, 69.2, 127.9, 128.0, 128.1, 128.2, 129.4, 129.5, 130.1, 131.4, 147.5, 147.6, 156.4.
SLNs preparation
The SLNs suspensions were prepared by dissolving 150 mg (0.119 mmol) of 3 in 5 mL THF. After 5 min of stirring, 50 mL of ultrapure water was added and the solution was stirred for one more minute. The tetrahydrofuran was subsequently evaporated under reduced pressure at 40 °C. The remaining solution was adjusted to 50 mL with ultrapure water to obtain a 3 mg mL−1 (2.4 mM) final concentration.
Scanning electron microscopy
Measurements were carried out by using a field-emission high-resolution scanning electron microscope by Merlin Carl Zeiss. Images were acquired to observe the surface morphology with a 15 kV incident accelerating voltage and 300 pA probe current to minimize the sample being altered by the imaging.
Agarose gel electrophoresis
Gel electrophoresis was conducted according to a common technique.25
Cytotoxicity assay
All cell types were seeded at an 11
000 cells per well concentration and allowed to adhere for 24 h at 37 °C in 5% CO2/95% air before treatments were initiated. The cells were then exposed to various concentrations of thiacalix[4]arenes for 72 h. Inverted optical microscopes Eclipse TS100 (Nikon), and CKX31 (Olympus), as well as a Fluorescence Microscope Olympus IX70, were used to assess cell viability relative to untreated cells. All measurements were carried out in triplicate and the experiments were carried out at least three times.
Acknowledgements
The financial support of the Program of the President of the Russian Federation for the State support of young Russian scientists, scholarships of the President of the Russian Federation (CP-769.2015.4), is gratefully acknowledged. This study was performed according to the Russian Government Program of Competitive Growth of Kazan Federal University.
Notes and references
- A. Hargrove, S. Nieto, T. Zhang, J. Sessler and E. Anslyn, Chem. Rev., 2011, 111, 6603–6782 CrossRef CAS PubMed.
- Enzymes: Biochemistry, Biotechnology and Clinical Chemistry, ed. T. Palmer, Horwood Publishing, Chichester, 2001, p. 416 Search PubMed.
- P. Beer and P. Gale, Anion Recognition and Sensing: The State of the Art and Future Perspectives, Angew. Chem., Int. Ed., 2001, 40, 486–516 CrossRef CAS.
- M. A. Mintzer and E. E. Simanek, Chem. Rev., 2009, 109, 259–302 CrossRef CAS PubMed.
- A. D. Miller, Curr. Med. Chem., 2003, 10, 1195–1211 CrossRef CAS PubMed.
- D. Nguyen, J. Green, J. Chan, R. Langer and D. G. Anderson, Adv. Mater., 2009, 21, 847–867 CrossRef CAS.
- I. Uchegbu, C. Dufes, P. Kan and A. G. Schatzlein, in Gene and Cell Therapy, ed. N. S. Templeton, CRC Press, Boca Raton, 3rd edn, 2009, pp. 321–339 Search PubMed.
- N. Ferrer-Miralles, E. Vazquez and A. Villaverde, Trends Biotechnol., 2008, 26, 267–275 CrossRef CAS PubMed.
- L. Gallego-Yerga, M. Lomazzi, V. Franceschi, F. Sansone, C. Ortiz Mellet, G. Donofrio, A. Casnati and J. M. García Fernández, Org. Biomol. Chem., 2015, 13, 1708–1723 CAS.
- A. Galukhin, K. Shabalin, I. Antipin, A. Konovalov and I. Stoikov, Mendeleev Commun., 2013, 23, 41–43 CrossRef CAS.
- D. Gutsche, in Calixarenes, Royal Society of Chemistry, Cambridge, 2nd edn, 2008, ch. 3, pp. 70–71 Search PubMed.
- N. Iki and S. Miyano, J. Inclusion Phenom. Macrocyclic Chem., 2001, 41, 99–105 CrossRef CAS.
- F. Sansone, M. Dudič, G. Donofrio, C. Rivetti, L. Baldini, A. Casnati, S. Cellai and R. Ungaro, J. Am. Chem. Soc., 2006, 128, 14528–14536 CrossRef CAS PubMed.
- V. Bagnacani, F. Sansone, G. Donofrio, L. Baldini, A. Casnati and R. Ungaro, Org. Lett., 2008, 10, 3953–3956 CrossRef CAS PubMed.
- Supramolecular Science: Where It Is and Where It Is Going, ed. R. Ungaro and E. Dalcanale, Springer, Netherlands, 1999, ch. Supramolecular Catalysis in Transition, pp. 273–286 Search PubMed.
- A. Galukhin, A. Erokhin, I. Imatdinov and Y. Osin, RSC Adv., 2015, 5, 33351–33355 RSC.
- A. Galukhin and I. Stoikov, Mendeleev Commun., 2014, 24, 82–84 CrossRef CAS.
- Y. Yonamine, K. Yoshimatsu, S.-H. Lee, Y. Hoshino, Y. Okahata and K. J. Shea, ACS Appl. Mater. Interfaces, 2013, 5, 374–379 CAS.
- I. Stoikov, A. Galukhin, E. Zaikov and I. Antipin, Mendeleev Commun., 2009, 19, 193–195 CrossRef CAS.
- P. Shahgaldian, M. Cesario, P. Goreloff and A. Coleman, Chem. Commun., 2002, 326–327 RSC.
- A. Coleman, S. Jebors, P. Shahgaldian, G. Ananchenko and J. Ripmeesterc, Chem. Commun., 2008, 2291–2303 RSC.
- P. Shahgaldian, E. Silva, A. Coleman, B. Rather and M. Zaworotko, Int. J. Pharm., 2003, 253, 23–38 CrossRef CAS PubMed.
- R. Dings, J. Levine, S. Brown, L. Astorgues-Xerri, J. MacDonald, T. Hoye, E. Raymond and K. Mayo, Invest. New Drugs, 2013, 31, 1142–1150 CrossRef CAS PubMed.
- K. Feichtinger, C. Zapf, H. L. Sings and M. Goodman, J. Org. Chem., 1998, 63, 3804 CrossRef CAS.
- M. Green and J. Sambrook, Molecular cloning: a laboratory manual, Cold Spring Harbor, New York, 4th edn, 2012, p. 2028 Search PubMed.
Footnote |
† Electronic supplementary information (ESI) available: Full details on charactirization of synthesized compounds. See DOI: 10.1039/c6ra04733e |
|
This journal is © The Royal Society of Chemistry 2016 |
Click here to see how this site uses Cookies. View our privacy policy here.