DOI:
10.1039/C6RA04458A
(Paper)
RSC Adv., 2016,
6, 32863-32873
Manipulating the multifunctionalities of polydopamine to prepare high-flux anti-biofouling composite nanofiltration membranes†
Received
19th February 2016
, Accepted 24th March 2016
First published on 28th March 2016
Abstract
A high-flux anti-biofouling composite nanofiltration (NF) membrane was facilely prepared by simultaneously manipulating the three functionalities (adhesion, reaction and separation) of polydopamine (PDA) via a two-step dip-coating method. Stemming from the adhesion and separation functionalities, PDA was in situ deposited on a polyethersulfone (PES) substrate and formed an ultrathin dense layer, which endowed the membrane with a satisfying rejection for a broad range of small organic molecules (methyl orange of 65.65%, orange GII of 79.05%, congo red of about 98.78%, methyl blue and alcian blue of nearly 100%) and a relatively high water flux of 249.45 L m−2 h−1 MPa−1 under the operation pressure of 0.2 MPa. Stemming from the reaction functionality, PDA could reduce silver ions when exposed to AgNO3 solution and trigger the in situ generation of silver nanoparticles (AgNPs) on the membrane surface, which conferred excellent anti-biofouling properties to the membrane with low bioadhesion and high antibacterial efficiency (almost 100%) against Escherichia coli. Moreover, the adhesion and reaction functionalities have a close relation to the separation functionality of PDA. The effects of PDA deposition time, AgNO3 treatment time and AgNO3 concentration on the separation performance of the resulting composite NF membranes were systematically investigated.
1. Introduction
Nanofiltration (NF) has been applied in a wide range of fields, such as bioseparation, water softening, the pharmaceutical industry and wastewater treatment.1,2 In those processes, membranes may suffer from severe fouling caused by complex contaminants in diverse water sources. In particular, biofouling has become a ubiquitous problem in efficient implementation of water treatment membranes including seawater desalination and wastewater treatment membranes.3 Compared with organic fouling caused by oils, proteins and natural organic matters (NOMs), biofouling is much more complicated and hard to mitigate, due to the complex compositions of biofoulants (bacterial cells, extracellular polymeric substances (EPS) and cell debris, etc.), as well as the complex fouling behaviors (physicochemical adhesion, biological proliferation and biofilm formation).4 Even trace amounts of attached cells may lead to eventual formation of an overwhelming biofilm, resulting in drastic decline of membrane performance.5,6 To endow membranes with anti-biofouling properties, one common, effective strategy has been devised in constructing anti-adhesive membrane surfaces with hydrophilic groups (e.g. PEG and zwitterionic groups) or non-polar low surface energy groups (e.g. silicone and fluoroalkyl groups), which could minimize the adhesion and settlement of organisms.7–12 In spite of the broad applicability and great popularity, there still exist inherent limitations in this strategy, owing to its unavailability in suppressing the colonization of bacteria, which may cause potential threats after long-term operation. Another alternative strategy is to construct antimicrobial membrane surfaces by introducing antimicrobial agents, either organic or inorganic, which could inhibit microbial colonization and prevent biofilm formation.13–23 Silver nanoparticles (AgNPs) have drawn much attention due to the broad-spectrum, long-lasting antimicrobial activity, and in recent years numerous approaches have been explored to fabricate anti-biofouling membranes with pre-synthesized or in situ generated AgNPs.20,24–35
Polydopamine (PDA), which can be generated under mild conditions by in situ spontaneous oxidative self-polymerization of dopamine, has been evolved as an emerging material in surface modification, because of its unique multi-functionalities and wide-applicability.36–38 First, PDA can tightly adhere to virtually all solid surfaces through multiple interactions, such as covalent bonding, coordination, hydrogen bonding and π–π stacking.36,39–43 Second, the residual catechol, quinone and amine groups of PDA could enable further reactions with functional molecules.44,45 On one hand, the quinone groups could react with amine and thiol groups via Michael addition or Schiff base reactions.46,47 On the other hand, the catechol moiety could strongly chelate with various metal ions and facilitate mineralization of inorganic particles (e.g. calcium carbonate vaterite, hydroxyapatite, metallic Au and Ag) on PDA surfaces via co-precipitation or redox reactions.36,48–51 Except for the adhesion and reaction functionalities, PDA is able to reduce the substrate pore size and form an ultrathin dense layer, which exhibits desirable separation performance for molecular sieving.52,53 Li et al. first fabricated a novel composite membrane with an ultrathin and stable active PDA layer on a microporous substrate by simply dip-coating method. The as-prepared composite membranes showed durable separation performance for pervaporative desulfurization.52 This approach opened a route for preparing composite materials with high efficiency and stability. In another work, Li et al. employed a similar dip-coating method to prepare a hydrophilic composite NF membrane, which exhibited an acceptable rejection for CaCl2.53 Liu et al. constructed a superhydrophobic separation layer over a Al2O3 disk by dip-coating in dopamine solution followed by Ag metallization and fluorination. The resultant membrane showed competitive pervaporation performance for bioalcohol recovery.54
To the best of our knowledge, the mussel-inspired surface modification approaches for anti-biofouling membranes are mostly based on two functionalities of PDA, adhesion and reaction, to endow various surfaces with anti-biofouling properties.26,33,55–60 For instance, Miller et al. modified ultrafiltration (UF) and NF membranes with PDA and PDA-g-PEG and the prepared membranes showed reduced short-term adhesion of P. aeruginosa.59 Tang et al. modified polysulfone (PSU) porous membranes with PDA followed by in situ generation of AgNPs to migrate biofouling. The hydrophilic PDA enhanced the membrane's bacterial anti-adhesive properties, while AgNPs imparted strong antimicrobial properties to the membrane, simultaneously.33 In these approaches, PDA was employed merely as a platform for further modification and hence the PDA deposition time was limited to avoid pore blockage and flux decline. However, the separation functionality of PDA layer is often neglected when constructing anti-biofouling membranes. In an effort to take maximum functionalities of PDA, Li et al. proposed a novel approach for fabricating antifouling high-flux NF membranes, involving dip-coating of PDA on a porous substrate and grafting of fluorinated polyamine via Michael addition reaction.2 The resultant membrane exhibited good separation performance for dyes and excellent antifouling properties. Nevertheless, the study merely focused on improving the anti-adhesive properties.
In this study, we proposed to prepare anti-biofouling composite NF membranes through the rational manipulation of three functionalities of PDA, i.e., adhesion, reaction and separation. The ultrathin PDA layer endowed the composite NF membrane with good separation performance for dyes, while the in situ generated AgNPs on PDA surface endowed the composite NF membrane with excellent anti-biofouling properties by elevating the surface hydrophilicity and releasing silver ions slowly. The surface morphologies and chemical compositions of the resultant membranes were probed employing scanning electron microscope (SEM), transmission electron microscope (TEM), atomic force microscopy (AFM), Fourier transform infrared (FTIR) spectroscopy, X-ray photoelectron spectroscopy (XPS), X-ray diffraction (XRD), Raman spectroscopy and water contact angle measurement. The separation performance was evaluated by the filtration of different kinds of dyes, while the anti-biofouling performance was assessed with Escherichia coli (E. coli) as a model foulant.
2. Experimental
2.1. Materials
Polyethersulfone (PES) UF membranes were used as the porous support for deposition of the PDA layer and were prepared via non-solvent phase separation (NIPS) method, which was described in our previous work.1 Dopamine hydrochloride was purchased from Yuancheng Technology Development Co. Ltd. (Wuhan, China). Tris(hydroxymethyl)aminomethane (Tris) was purchased from Sigma-Aldrich Co. (Shanghai, China). Silver nitrate (AgNO3) was purchased from Tianjin Guangfu Company. Alcian blue (Mw = 1298.86) was purchased from Aladdin Industrial Corporation (Shanghai, China). Hydrochloric acid (HCl), nitric acid (HNO3), methyl orange (Mw = 327.33), orange GII (Mw = 452.36), congo red (Mw = 696.88) and methyl blue (Mw = 799.80) were purchased from Kewei Chemicals Co. (Tianjin, China). Wild Escherichia coli (E. coli) was obtained from Beijing TransGen Biotech Co., Ltd. Deionized water used throughout this study was obtained from a three-stage Milli-Q Plus system (Millipore, Billerica, USA). All the reagents were of analytical grade and used without further purification.
2.2. Membrane preparation
Dopamine aqueous solution (2.0 g L−1) was obtained by dissolving 50 mg dopamine hydrochloride in 25 mL Tris–HCl buffer solution (pH = 8.5 ± 0.2, 50.0 mM). The prepared PES UF membranes were cut into circular pieces (diameter of 7.2 cm) and submerged in dopamine solution. Then the system was shaken at room temperature for a scheduled period of time (2 to 24 h) with the solution left open to the air. After taken out from the solution, the membranes were rinsed for several times with deionized water under ultrasonication and gently dried in the air. The resultant membranes, designated as PDA/PES composite membranes, were used for characterization and further surface modification.
Subsequently, the PDA/PES composite membranes were incubated in 25 mL AgNO3 aqueous solution with the AgNO3 concentration varying from 0.1 g L−1 to 5 g L−1. Then the system was shaken at room temperature for a scheduled period of time (2 to 36 h) without contacting with air or light. PDA/PES composite membranes treated by pure water were taken as the control membranes when altering PDA deposition time and AgNO3 treatment time. Then the resultant membranes, designated as AgNPs–PDA/PES composite membranes, were taken out and rinsed for several times with deionized water under ultrasonication before further characterization and evaluation.
The membranes prepared with different PDA deposition time, AgNO3 concentrations and AgNO3 treatment time were named as #n (n is the membrane number) and listed in Table 1 respectively.
Table 1 The resultant composite membranes with different preparation conditions (PDA deposition time, AgNO3 concentration and AgNO3 treatment time)a
Membraneb |
PDA deposition timec (h) |
AgNO3 concentrationb (g L−1) |
AgNO3 treatment time (h) |
All the resultant composite membranes were prepared at room temperature. PDA–PES composite membranes post-treated by pure water were taken as the control membranes when altering PDA deposition time and AgNO3 treatment time. PDA deposition was carried out with dopamine concentration of 2 g L−1 and pH of 8.5. |
#0 |
0 |
0 |
0 |
#1 |
4 |
0 |
0 |
#2 (#2′) |
4 |
0(1) |
2 |
#3 (#3′) |
4 |
0(1) |
4 |
#4 (#4′) |
4 |
0(1) |
8 |
#5 (#5′) |
4 |
0(1) |
12 |
#6 (#6′) |
4 |
0(1) |
24 |
#7 (#7′) |
4 |
0(1) |
36 |
#8 (#8′) |
2 |
0(1) |
12 |
#9 (#9′) |
8 |
0(1) |
12 |
#10 (#10′) |
12 |
0(1) |
12 |
#11 (#11′) |
24 |
0(1) |
12 |
#12 |
4 |
0.1 |
12 |
#13 |
4 |
0.5 |
12 |
#14 |
4 |
2 |
12 |
#15 |
4 |
5 |
12 |
2.3. Membrane characterization
Attenuated total reflectance Fourier transform infrared spectroscopy (ATR-FTIR). In order to analyze the functional groups on the membrane surfaces, ATR-FTIR (Vector 22 FTIR spectrometer, Bruker Optics) was used and the reflection spectra were recorded over a range of 400 to 4000 cm−1 after baseline correction. The resolution was 4 cm−1 and the scan number was 32.
X-ray photoelectron spectroscopy (XPS). The membrane surface chemical composition was characterized by XPS (PHI-1600 X-ray photoelectron spectrometer, USA) using Mg Kα (1254.0 eV) as radiation source. Survey spectra of the membranes were collected in the region of 0–1100 eV with the photoelectron take-off angle at 90°.
Scanning electron microscopy (SEM). Surface and cross-section morphologies of the membrane samples were observed with a field emission scanning electron microscope (FESEM, Nanosem 430) under the operating voltage of 10 kV. The membrane samples frozen in liquid nitrogen were broken and sputtered with gold before SEM analysis.
Atomic force microscopy (AFM). To measure the membrane surface roughness and morphologies, AFM (Multimode 3, Bruker Co.) with Si3N4 cantilevers and resonance frequency of 1.0 Hz was employed with a scan size of 5 μm × 5 μm. Moreover, AFM could also be used to evaluate the interaction forces between the membrane surfaces and AFM tips, which were modified with model molecules (e.g. bovine serum albumin (BSA)).
Raman spectra. Raman spectra were measured using a Laser Micro-Raman Spectrometer (DXR Microscope, Thermo Finnigan, USA). All the samples were excited under a He–Ne laser (532 nm) with 1.0 mW incident laser power level.
X-ray diffraction (XRD). The crystallization properties of the membrane surfaces were investigated by XRD (D/MAX-2500 X-ray diffractometer (Cu Kα)) under wide-angle patterns with the diffraction angle (2θ) ranging from 5° to 80°.
Transmission electron microscope (TEM). The AgNPs on membrane surfaces were further characterized by high-resolution transmission electron microscopy (HRTEM, JEM-100CX II) equipped with Schottky FEG and energy dispersive X-ray detector (EDX). The samples were prepared by dissolving the AgNPs–PDA/PES composite membranes in N,N-dimethylformamide (DMF). The solution was centrifuged and re-dispersed in DMF under ultrasonication. Then the procedure was repeated for three times and the samples were dispersed in water and loaded on copper grids before TEM analysis.
Silver release determination. The silver release rate of the AgNPs–PDA/PES composite membrane was quantified by a batch test for up to 14 days.27 A piece of the prepared membrane sample (1 cm × 1 cm) was immersed in 20 mL deionized water and shaken at 150 rpm under room temperature. Every 24 h, the water was collected and replaced by fresh water. All the water samples were acidified by 1% HNO3 before measurement of Ag+ content by an inductively coupled plasma optical emission spectrophotometer (ICP, ICP-9000 (N+M), Thermo Jarrell-Ash Co., USA). The total mass of released silver was calculated by summarizing the mass of the released silver each day.
Thermogravimetric analysis (TGA). The total amount of the AgNPs on the AgNPs–PDA/PES composite membrane was determined by TGA (NETZSCH TG209 F3) under air flow at a heating rate of 10 °C min−1.
Static water contact angle measurements. Static water contact angles (CA) of the membranes were measured at room temperature, using a contact angle goniometer (JC2000C Contact Angle Meter, Powereach Co., Shanghai, China). A water droplet (5 μL) was dropped onto the membrane surface with a microsyringe and the CA was measured after 5 s. At least six contact angles at different locations on each membrane surface were averaged to acquire a reliable value.
2.4. Evaluation of membrane separation performance
A dead-end stirred cell filtration system was employed to evaluate the separation performance. The system contained a filtration cell (model 8200, Millipore Co.) with an inner diameter of 62 mm and a volume capacity of 200 mL. The effective area of the NF membrane sample was 28.7 cm2. The filtration cell was equipped with a nitrogen gas cylinder and a solution reservoir. All the tests were conducted under the constant pressure of 0.20 MPa (maintained by nitrogen gas) and temperature of 25 ± 1 °C. The feeding pH was 6.0 ± 0.2. The pressure was initially fixed at 0.25 MPa for about 0.5 h to get each membrane compacted and obtain a stable flux. Then the pressure was lowered to the operation pressure of 0.20 MPa. The pure water flux (Jw) was calculated by eqn (1):1 |
 | (1) |
where V (L) was the permeated water volume, A (m2) was the membrane effective area and Δt (h) was the operation time.
Organic dyes were used to determine the rejection properties of the composite membranes. The filter cell was filled with aqueous solutions of dyes (0.1 g L−1, pH = 6.0 ± 0.2). The permeate solution was collected until the fluxes and dye concentrations reached the equilibrium values. The rejection (R) was calculated by eqn (2):1
|
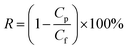 | (2) |
where
Cp and
Cf were dye concentrations of permeate and retentate solutions, respectively. Dye concentrations were determined using a UV-vis spectrophotometer (Hitachi UV-2800, Hitachi Co., Japan) with the wavelength adjusted to the maximum adsorption of each dye solution in visible light range.
2.5. Evaluation of membrane anti-biofouling performance
The anti-biofouling performance of the prepared membranes was investigated via disk diffusion assay, antibacterial efficiency test and SEM observation with E. coli, a Gram-negative bacterium, as test microorganisms.7,25,27,61 The E. coli was inoculated into liquid lysogeny broth (LB, 20 g L−1) and incubated overnight under 37 °C. Then the culture was centrifuged and resuspended in LB solution to obtain a bacterial stock solution (approximately 105 colony forming units (CFUs) per mL).
Disk diffusion assay. The anti-biofouling activities of the composite membranes were investigated by a disk diffusion method in an agar medium, which was reported elsewhere.27 200 μL of E. coli bacterial stock solution was spread uniformly over a solidified nutrient agar plate. After sterilized by ultraviolet radiation for 30 min, membrane samples (diameter of 3 cm) were then placed onto the culture plate with the active layer in contact with the agar surface. Then the culture plates were incubated at 37 °C for 24 h before observation. The anti-biofouling activities of the composite membranes were estimated by the existence of a clear inhibition zone around membrane samples.
SEM observation and antibacterial efficiency test. To assess the anti-biofouling activities of the composite membranes quantitatively, antibacterial efficiency tests were carried out. 200 μL of E. coli bacterial stock solution was added into liquid lysogeny broth (LB, 20 g L−1, 15 mL). Membrane samples (diameter of 3 cm) after sterilization were immersed into the suspensions separately, followed by shaking at 250 rpm at 37 °C for 24 h. Then the membrane samples were taken out and immersed into aqueous glutaraldehyde solution (2.5 wt%) at 4 °C for 6 h to fix the bacteria onto membrane surfaces. After rinsed for three times with PBS solution, the membrane samples were step-dehydrated with ethanol series, dried in the air and sputtered with gold before SEM observation.After taking out the membrane samples, each suspension was pipetted out and diluted by 108 times, subsequently. 200 μL of the diluted suspension was spread over a solidified nutrient agar plate. After an overnight incubation at 37 °C, the number of the viable colonies was counted respectively. The antibacterial efficiency was calculated by the following equation:62
|
 | (3) |
where
N0 is the number of the colonies on the solidified nutrient agar plate which was incubated with the control suspension without any treatment,
N is the number of the colonies on the solidified nutrient agar plate which was incubated with the membrane-treated suspension.
3. Results and discussion
3.1. Preparation mechanism of the composite membranes
The preparation procedure of the AgNPs–PDA/PES composite membrane, including two simple immersion steps, was illustrated in Scheme 1. In the PDA deposition step, dopamine, with catechol and amine groups, was oxidized and self-polymerized into PDA nanoaggregates through multiple interactions, including covalent bonding, hydrogen bonding and π–π stacking.39,63 The PDA nanoaggregates, with residual catechol groups, primarily adhered to the polar PES surface through hydrogen bonding interaction. Afterwards, the PDA nanoaggregates were further anchored to the PES surface by hydrophobic and π–π interactions, resulting in pore shrinkage and in situ formation of a thin PDA layer.37 In the surface metallization step, the silver ions first chelated with the catechol groups on PDA surface, because the catechol groups were strong bidentate ligands. Moreover, the catechol groups have a relatively high reductive ability with a redox potential of −795 mV.64 Hence they could easily give electrons to silver ions and generate AgNPs, converting themselves into quinone groups simultaneously.65 The resultant quinone groups could subsequently participate in the self-polymerization process, while the residual catechol groups immobilized the AgNPs on PDA surface. In this way, a AgNPs–PDA hybrid coating was formed on the PES support. Besides, the apparent color changes of the membrane surface from white (virginal PES) to dark brown (PDA/PES) and then to silvery grey (AgNPs–PDA/PES) confirmed the preparation mechanism of the AgNPs–PDA/PES composite membrane.
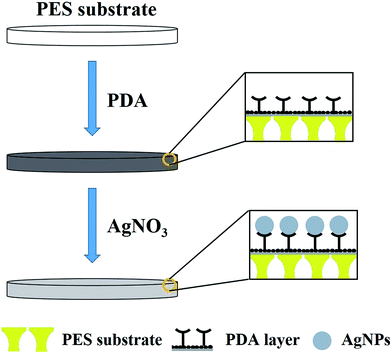 |
| Scheme 1 Schematic illustration for the preparation procedure of the AgNPs–PDA/PES composite membrane. | |
3.2. Characterization of the composite membranes
In order to further demonstrate the hypothesized modification procedure, the chemical composition of the resultant membranes was systematically analyzed. FTIR spectra revealed the successful deposition of PDA, but failed to prove the generation of AgNPs (Fig. S1†).
XPS analysis was employed to further determine the surface chemical composition of the membranes quantitatively. The wide scan XPS spectra of various membranes were shown in Fig. 1(a). For the PDA/PES composite membrane (#2), a new N1s signal appeared in the spectrum, which was ascribed to the deposition of PDA on the membrane surface. After AgNO3 treatment for 2 h, apparent Ag3d signals could be detected for the AgNPs–PDA/PES composite membrane (#2′). With the increase of the AgNO3 treatment time to 12 h, the intensity of the Ag3d signals were obviously increased and additional signals, including Ag3s, Ag3p, Ag4s, Ag4p and Ag4d, were presented in the spectrum of the AgNPs–PDA/PES composite membrane (#5′). In order to further confirm the status of the Ag element on the membrane surface, high-resolution Ag3d XPS spectrum of the AgNPs–PDA/PES composite membrane (#2′) was analyzed in detail (Fig. 1(b)). The doublet signals at 368.2 eV and 374.2 eV coincided exactly with the standard binding energy of Ag3d5/2 and Ag3d3/2 for pure Ag0 without any shift.66 This revealed that the silver existed on the membrane surface was at zero valence,56 confirming the formation of AgNPs. The surface elemental composition data were listed in Table 2. It could be seen that the atomic percentage of Ag was increased from 0.8 at% to 5.4 at%, which was in agreement with the spectra results. Moreover, the atomic percentage of S became negligible for all the composite membranes (#2, #2′ and #5′). This was because the thickness of the PDA layer could reach nearly 30 nm after 4 h PDA deposition (observed from Fig. S2† and agreed with the data reported by Lee et al.36), which was larger than the XPS detection deepness (∼10 nm).
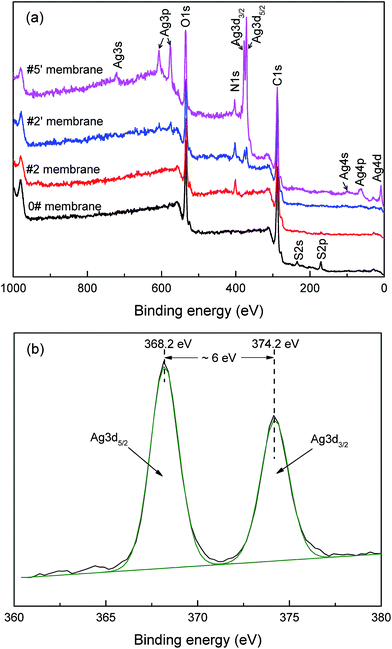 |
| Fig. 1 (a) Wide scan XPS spectra of the PES substrate (#0), the PDA/PES composite membrane (#2) and the AgNPs–PDA/PES composite membranes (#2′ and #5′) and (b) high-resolution Ag3d XPS spectrum of the AgNPs–PDA/PES composite membrane (#2′). | |
Table 2 Surface elemental composition of the PES substrate (0#), the PDA/PES composite membrane (2#) and the AgNPs–PDA/PES composite membranes (2′# and 5′#)
Membrane |
Surface elemental composition (at%) |
C |
O |
N |
S |
Ag |
0# |
70.4 |
27.1 |
— |
2.5 |
— |
2# |
73.5 |
19.9 |
6.0 |
— |
— |
2′# |
71.6 |
21.5 |
6.1 |
— |
0.8 |
5′# |
70.0 |
19.1 |
5.5 |
— |
5.4 |
SEM was utilized to observe the surface structure of the as-prepared composite membranes and the results were shown in Fig. 2. The PES substrate (#0) exhibited a clear and smooth surface (Fig. 2(a)). From the enlarged image (Fig. 2(a′)), a distribution of nanosized pores could be observed over the PES surface. After PDA deposition, the membrane surface became relatively rough (Fig. 2(b)) with several scattered PDA particles with different sizes (Fig. 2(b′)). This was attributed to the accumulation and conjugation of the PDA nanoaggregates via multiple interactions including covalent bonding, hydrogen bonding and π–π stacking.39,63 Moreover, no pore could be visible on the PDA/PES and membrane surfaces, indicating that PDA had formed a continuous layer and led to pore blockage to the substrate, as presented in Fig. S2.† After AgNO3 treatment, the membrane surface was covered by numbers of nanoparticles with diameters ranging from about 30 nm to about 60 nm (Fig. 2(c) and (c′)), owing to the generation of AgNPs. As the AgNO3 treatment time increased from 2 h to 12 h, more AgNPs were formed without apparent change in size, covering almost the whole membrane surface with the presence of several large aggregates (Fig. 2(d) and (d′)).
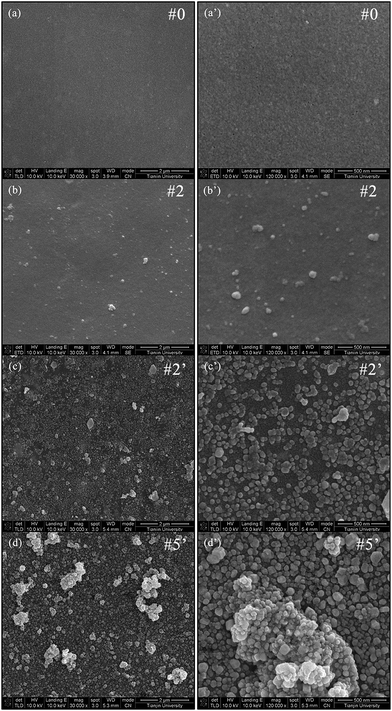 |
| Fig. 2 SEM images with different scales of the membrane surface structure: (a) and (a′) the PES substrate (0#), (b) and (b′) the PDA/PES composite membrane (2#), (c) and (c′) the AgNPs–PDA/PES composite membrane (2′#), (d) and (d′) the AgNPs–PDA/PES composite membrane (5′#). | |
AFM analysis was employed to further observe the surface morphology and topology of the membranes. Fig. 3 showed the 4 μm × 4 μm three-dimensional AFM images of the membrane surfaces as well as their mean roughness (Ra). The mean roughness (Ra) of the PES substrate (#0) was 7.43 nm, while that of the PDA/PES composite membrane (#2) was 8.73 nm. The slight increase of the roughness was caused by the existence of the PDA nanoparticles on the modified membrane surface, as presented in Fig. 2(b′). After AgNO3 treatment, the mean roughness (Ra) was dramatically increased to 15.30 nm and 44.90 nm for the AgNPs–PDA/PES composite membranes (#2′ and #5′), respectively, originating from the generation and accumulation of AgNPs. The exact consistence between the AFM results and the SEM observations confirmed the successful deposition of PDA, generation and immobilization of AgNPs.
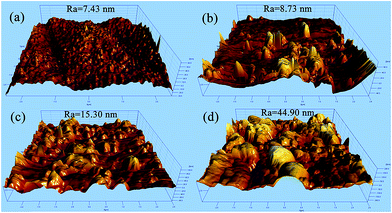 |
| Fig. 3 AFM morphology of (a) the PES substrate (#0), (b) the PDA/PES composite membrane (#2), (c) the AgNPs–PDA/PES composite membrane (#2′), (d) the AgNPs–PDA/PES composite membrane (#5′). | |
In addition, Raman spectroscopy was utilized to investigate the surface modification process and the results were shown in Fig. 4. Apparently, two broad peaks appeared at 1364 cm−1 and 1585 cm−1 in the spectrum of the PDA/PES composite membrane (#2) with the disappearance of the characteristic peaks of the PES substrate (#0). These two peaks were attributed to the residual catechol groups of PDA. Moreover, after AgNO3 treatment, the two peaks were remarkably enhanced without any shift, as shown in the spectra of the resultant AgNPs–PDA/PES composite membranes (#2′ and #5′). This phenomenon might be caused by the surface-enhanced Raman scattering (SERS) effect of AgNPs, which was reported as a kind of efficient plasmonic material.67 Therefore, the hypothesis of the formation of AgNPs was proved to a certain extent.
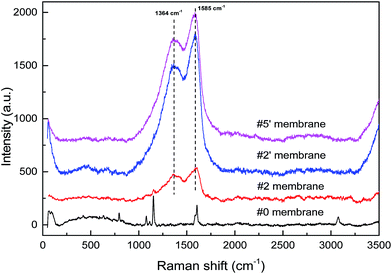 |
| Fig. 4 Raman spectra of the PES substrate (#0), the PDA/PES composite membrane (#2) and the AgNPs–PDA/PES composite membrane (#2′ and #5′). | |
In order to ascertain the structure of the AgNPs mediated by PDA, several techniques were utilized, such as XRD and HRTEM. XRD was carried out to characterize the crystalline structure of the AgNPs. As shown in Fig. 5, the PES substrate (#0) had no additional diffraction peak but merely a broad peak as same as the PDA/PES composite membrane (#2) around 2θ of 20°, demonstrating that PDA is a non-crystalline material.56 After AgNO3 treatment for 12 h, four sharp and intense diffraction peaks emerging at 38.10°, 44.25°, 64.54° and 77.60° could be observed in the XRD pattern of the AgNPs–PDA/PES composite membrane (#5′), corresponding to the (111), (200), (220) and (311) crystalline planes of face centered cubic (FCC) silver crystal (JCPDS no. 65-2871), respectively. Meanwhile, the results were further confirmed by the TEM observations (Fig. S3†).
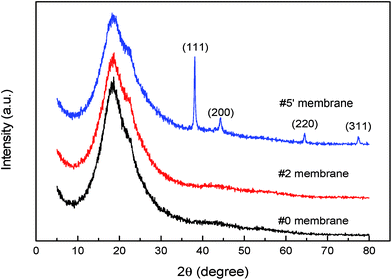 |
| Fig. 5 XRD patterns of the PES substrate (#0), the PDA/PES composite membrane (#2) and the AgNPs–PDA/PES composite membrane (#5′). | |
3.3. Separation performance of the composite membranes
In order to optimize the separation performance of the composite membranes, a series of experiments were implemented to tailor the structure of the active layer through varying PDA deposition time, AgNO3 treatment time as well as AgNO3 concentration. Orange GII aqueous solution (0.1 g L−1, pH = 6.0 ± 0.2) was used as the testing solution to evaluate the selectivity property of the membranes. The results were shown in Fig. S4–S6,† respectively. From the results, it can be concluded that PDA deposition may cause pore blockage, leading to the decrease of pure water flux and the increase of rejection. Moreover, from Fig. S4 and S5,† AgNO3 treatment further decreased the pure water flux and increased the rejection. Two reasons could be proposed to rationalize this phenomenon. One is that the in situ generated AgNPs might block the pores on the PDA layer surface. The other is that silver ions might cause the oxidation of residual catechol groups, facilitating the self-crosslinking of the PDA oligomers or nanoaggregates in the PDA layer.
Furthermore, to confirm the broad applicability of the resultant composite membranes for NF applications (especially dye removal), a series of dyes with different molecular weights were chosen for evaluation. As shown in Fig. 6, under optimized conditions, the AgNPs–PDA/PES composite membrane (#10′) showed a satisfying rejection performance with 65.65% for methyl orange, 79.05% for orange GII, 98.78% for congo red and above 99.50% for both methyl blue and alcian blue. This can be explained be the synergistic effect of the size exclusion and Donnan effect. For relatively large dye molecules (congo red, methyl blue and alcian blue), size exclusion was mainly responsible for the excellent rejections. For smaller dye molecules (methyl orange and orange GII) that were able to pass through the pores within the PDA layer, the Donnan effect was mainly responsible for the dye rejections. This was due to the repulsive interaction between the negatively charged PDA layer and dye molecules.1 Additionally, the pure water flux was 249.45 L m−2 h−1 MPa−1, which outperformed most of the NF membranes in previous studies (listed in Table S1†).
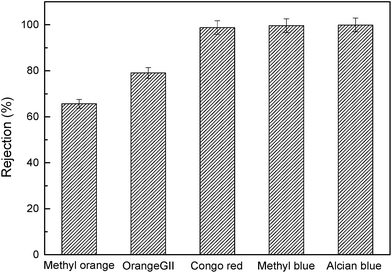 |
| Fig. 6 The separation performance of the AgNPs–PDA/PES composite membrane (#10′) for different dyes (methyl orange, orange GII, congo red, methyl blue and alcian blue). | |
3.4. Anti-biofouling performance of the composite membranes
As a major premise for anti-biofouling performance, the antibacterial activities of the composite membranes were first investigated by a disk diffusion method with E. coli as the model foulant. As shown in Fig. 7(a), the PES substrate (#0) showed no inhibition against the E. coli growth with numerous E. coli colonies generated all over the culture plate. After PDA deposition, there existed an indistinct inhibition zone around the PDA/PES composite membrane (#2) sample, where few E. coli colonies formed (Fig. 7(b)). This was because PDA, with a certain amount of catechols, might possess a certain extent of antibacterial capacity like other polyphenols.13 In Fig. 7(c) and (d), a clear inhibition zone could be observed around the membrane samples, indicating excellent antibacterial properties of the AgNPs–PDA/PES composite membranes (#2′ and #5′). The antibacterial properties of the AgNPs–PDA/PES composite membranes were endowed by the AgNPs, which could release biotoxic silver ions. Moreover, the increase of AgNO3 treatment time from 2 to 12 h did not apparently affect the width of the inhibition zone, revealing that even a short-time AgNO3 treatment could bring a satisfying antibacterial activity to the composite membrane. In this way, the aggregation of AgNPs on the membrane surface as shown in Fig. 2(d) can be avoided.
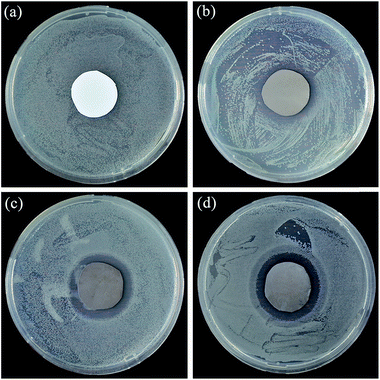 |
| Fig. 7 The antibacterial results of (a) the PES substrate (#0), (b) the PDA/PES composite membrane (#2), (c) the AgNPs–PDA/PES composite membrane (#2′) and (d) the AgNPs–PDA/PES composite membrane (#5′) against E. coli in disk diffusion assays. | |
In order to quantitatively measure the antibacterial activities of the composite membranes, antibacterial efficiency test was carried out and the results were shown in Fig. 8 and Table 3. The PES substrate (#0) showed a negligible antibacterial efficiency of 9.01%, while the PDA/PES composite membrane (#2) exhibited an antibacterial efficiency of 82.88%, proving the antibacterial activity of PDA as described in Fig. 7. However, there still existed some E. coli colonies on the recultivated agar (Fig. 8(c)). This was because that PDA could hardly be released and only inhibit the growth of the bacterial cells which contacted the membrane surface. After AgNO3 treatment, the antibacterial efficiency of the AgNPs–PDA/PES composite membranes (#2′ and #5′) could as high as 100%, indicating that the AgNPs–PDA/PES composite membranes showed great potency in inhibiting the proliferation of biofoulants.
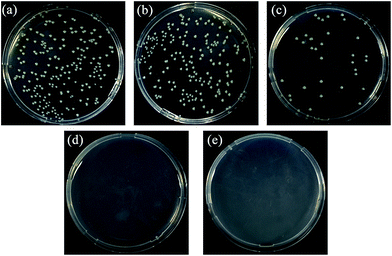 |
| Fig. 8 Photographs of the recultivated E. coli colonies on agar from the incubated suspensions with (a) no addition of membrane and addition of (b) the PES substrate (#0), (c) the PDA/PES composite membrane (#2), (d) the AgNPs–PDA/PES composite membrane (#2′) and (e) the AgNPs–PDA/PES composite membrane (#5′). | |
Table 3 Antibacterial efficiency of the resultant membranes
Membrane |
Number of colonies |
Antibacterial efficiency (%) |
The colonies on the solidified nutrient agar plate was incubated with the control suspension without any treatment. |
Nonea |
222 |
— |
#0 |
202 |
9.01 |
#2 |
38 |
82.88 |
#2′ |
0 |
100 |
#5′ |
0 |
100 |
Since the antibacterial property was attributed to the silver ions released from the AgNPs, the release rate should determine the long-term stability of the AgNPs–PDA/PES composite membranes. The release rate silver ions of was measured via a batch test with the AgNPs–PDA/PES composite membrane (#5′) as the testing sample. As presented in Fig. 9, the initial release rate of silver ions was 0.63 μg cm−2 per day. Afterwards, the release rate quickly levelled off to around 0.10 μg cm−2 per day after 5 days and remained nearly constant till the end of the test. After 14 days, the total mass of the released silver was 2.57 μg cm−2. The results were comparable to those of literature, indicating the slow leaching rate.27 According to the relatively high content of the AgNPs for the AgNPs–PDA/PES composite membrane (#5′) (Fig. S7 and Table S2†), it could be derived that the antibacterial activity of the membrane could be effective for long-time operation. On one hand, the long durability of the composite membrane might result from the large content of the AgNPs generated on the membrane surface. On the other hand, the silver release in water was governed by oxidation of the metallic Ag(0) as shown in eqn (4) and (5).68 However, the PDA-mediated AgNPs were less sensitive to oxygen, leading to a slower release rate.69,70 Furthermore, the strong coordination interactions between the catechol groups and the AgNPs as well as the silver ions could also slow down the release rate. In addition, the slow leaching rate as well as the high flux could also maintain the lower silver concentration of the membrane permeate than the established maximum contaminant limit in drinking water (i.e. 100 μg L−1).33
|
2Ag2O + 4H+ → 4Ag+ + 2H2O
| (5) |
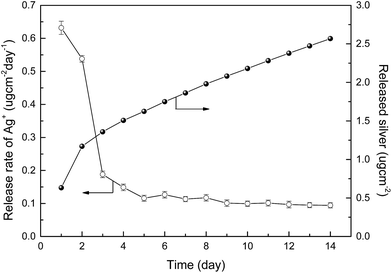 |
| Fig. 9 Silver ion release rate of the AgNPs–PDA/PES composite membrane (#5′) in the batch test. | |
Expect for antibacterial activity, anti-bioadhesion property was also significant for anti-biofouling performance of the membranes. The interaction forces between the membranes and cells were probed in detail using an AFM tip immobilized with BSA, which was applied as a model for extracellular polymeric substances (EPS). As presented in Fig. 10(a)–(c), the interaction force between the membrane surface and BSA-immobilized AFM tip was obviously increased from 5.39 nN for the PES substrate (#0) to 11.28 nN for the PDA/PES composite membrane (#2) and then dramatically decreased down to 4.12 nN for the AgNPs–PDA/PES composite membrane (#5′). The strong interaction force for the PDA/PES composite membrane (#2) was arisen from the multiple interactions between the functional groups of PDA and the proteins. The low interaction force for the AgNPs–PDA/PES composite membrane (#5′) was probably attributed to the enhancement of the surface hydrophilicity of the composite membranes after AgNPs immobilization, which was proved by the static water contact angle results (Fig. S4†). Moreover, the AgNPs might create a barrier and reduce the contact area between the proteins or cells and the PDA layer and hence hinder the settlement.
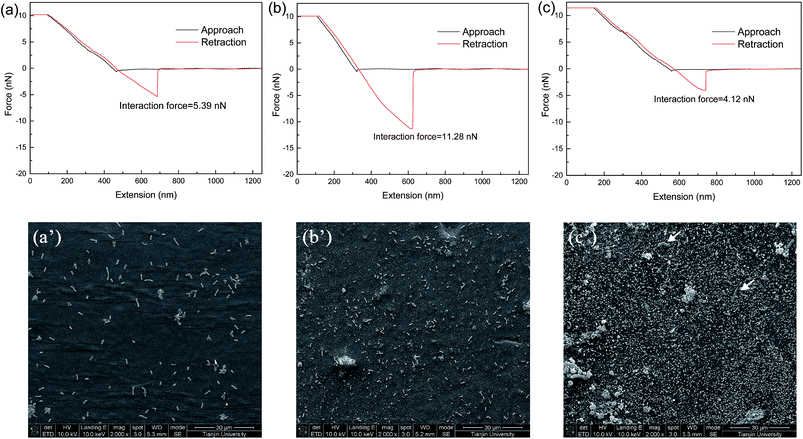 |
| Fig. 10 (a)–(c): SEM surface images of the PES substrate (#0), the PDA/PES composite membrane (#2) and the AgNPs–PDA/PES composite membrane (#5′) after immersed in the incubated suspensions, respectively. (a′)–(c′): AFM force–extension curves and interaction forces of the PES substrate (#0), the PDA/PES composite membrane (#2) and the AgNPs–PDA/PES composite membrane (#5′) recorded using an AFM tip immobilized with BSA, which was applied as a model for extracellular polymeric substances (EPS). | |
SEM images were utilized to observe the membrane samples incubated along with the E. coli suspensions. As shown in Fig. 10(a′), rod-shaped E. coli cells appeared on the PES substrate (#0) surface. After PDA deposition, the amount of attached cells was still increased although the sample exhibited antimicrobial activity to some extent (Fig. 10(b′)). This was due to the strong adhesive property of PDA which robustly anchored E. coli cells. On the contrary, the AgNPs-PDA/PES composite membrane (#5′) surface was rather clean with only few disrupted cells attached (as pointed out by arrows in Fig. 10(c′)). This revealed a generic anti-biofouling property of the AgNPs–PDA/PES composite membranes, which was attributed to both antimicrobial and anti-adhesive properties.
4. Conclusions
In summary, we demonstrated a facile mussel-inspired approach, which involved two simple dip-coating steps under mild conditions, to fabricate anti-biofouling composite NF membranes. PDA, as a multi-functional agent, adhered to the PES porous substrate and decreased the pore size, forming an ultrathin separation layer. Moreover, the PDA layer also enabled the formation of AgNPs via redox reaction with silver ions. The separation performance, which was governed by the ultrathin PDA layer, could be well controlled by tuning the preparation conditions. Under optimized preparation condition, the composite NF membrane exhibited a good separation performance with a relatively high pure water flux as well as satisfying rejections for a range of small organic molecules. The excellent anti-biofouling properties of the composite NF membranes were afforded by the in situ generated AgNPs, which not only improved the anti-bioadhesion property by enhancing the surface hydrophilicity, but also endowed the membrane with antibacterial property by releasing silver ions. It could be conjectured that by integrating the adhesion, reaction and separation functionalities of PDA, ultrathin functionalized PDA layers could be designed and tailored conveniently. In this way, diverse composite membranes could be fabricated for a variety of separation applications.
Acknowledgements
The authors thank the financial support from the National Science Fund for Distinguished Young Scholars (21125627), Tianjin Natural Science Foundation (14JCZDJC37400, 13JCYBJC20500), and the Program of Introducing Talents of Discipline to Universities (no. B06006). We are also grateful for the help from Prof. Xing Fu and Fei He for AFM and XPS measurements, respectively. The assistance from Yue Zhang for the bacterial incubation was appreciated as well.
Notes and references
- R. Zhang, Y. Su, X. Zhao, Y. Li, J. Zhao and Z. Jiang, J. Membr. Sci., 2014, 470, 9–17 CrossRef CAS.
- Y. Li, Y. Su, X. Zhao, X. He, R. Zhang, J. Zhao, X. Fan and Z. Jiang, ACS Appl. Mater. Interfaces, 2014, 6, 5548–5557 CAS.
- J. Mansouri, S. Harrisson and V. Chen, J. Mater. Chem., 2010, 20, 4567–4586 RSC.
- M. Lejars, A. Margaillan and C. Bressy, Chem. Rev., 2012, 112, 4347–4390 CrossRef CAS PubMed.
- M. Herzberg and M. Elimelech, J. Membr. Sci., 2007, 295, 11–20 CrossRef CAS.
- E. Huertas, M. Herzberg, G. Oron and M. Elimelech, J. Membr. Sci., 2008, 318, 264–270 CrossRef CAS.
- C. X. Liu, D. R. Zhang, Y. He, X. S. Zhao and R. Bai, J. Membr. Sci., 2010, 346, 121–130 CrossRef CAS.
- W. Chen, Y. Su, J. Peng, Y. Dong, X. Zhao and Z. Jiang, Adv. Funct. Mater., 2011, 21, 191–198 CrossRef CAS.
- X. T. Zhao, Y. L. Su, Y. F. Li, R. N. Zhang, J. J. Zhao and Z. Y. Jiang, J. Membr. Sci., 2014, 450, 111–123 CrossRef CAS.
- L. Tang, W. Gu, P. Yi, J. L. Bitter, J. Y. Hong, D. H. Fairbrother and K. L. Chen, J. Membr. Sci., 2013, 446, 201–211 CrossRef CAS.
- M. S. Rahaman, H. Thérien-Aubin, M. Ben-Sasson, C. K. Ober, M. Nielsen and M. Elimelech, J. Mater. Chem. B, 2014, 2, 1724 RSC.
- M. Ginic-Markovic, T. Barclay, K. T. Constantopoulos, T. Al-Ghamdi, A. Blok, E. Markovic and A. V. Ellis, RSC Adv., 2015, 5, 63017–63024 RSC.
- Y. S. Choi, H. Kang, D. G. Kim, S. H. Cha and J. C. Lee, ACS Appl. Mater. Interfaces, 2014, 6, 21297–21307 CAS.
- P. K. S. Mural, A. Banerjee, M. S. Rana, A. Shukla, B. Padmanabhan, S. Bhadra, G. Madras and S. Bose, J. Mater. Chem. A, 2014, 2, 17635–17648 CAS.
- Y. Feng, X. Lin, H. Li, L. He, T. Sridhar, A. K. Suresh, J. Bellare and H. Wang, Ind. Eng. Chem. Res., 2014, 53, 14974–14981 CrossRef CAS.
- J. Meng, X. Zhang, L. Ni, Z. Tang, Y. Zhang, Y. Zhang and W. Zhang, Desalination, 2015, 359, 156–166 CrossRef CAS.
- M. Ben-Sasson, K. R. Zodrow, Q. Genggeng, Y. Kang, E. P. Giannelis and M. Elimelech, Environ. Sci. Technol., 2014, 48, 384–393 CrossRef CAS PubMed.
- H. Karkhanechi, R. Takagi, Y. Ohmukai and H. Matsuyama, Desalination, 2013, 325, 40–47 CrossRef CAS.
- G. Yeroslavsky, O. Girshevitz, J. Foster-Frey, D. M. Donovan and S. Rahimipour, Langmuir, 2015, 31, 1064–1073 CrossRef CAS PubMed.
- M. S. Mauter, Y. Wang, K. C. Okemgbo, C. O. Osuji, E. P. Giannelis and M. Elimelech, ACS Appl. Mater. Interfaces, 2011, 3, 2861–2868 CAS.
- Q. Zhao, C. Liu, J. Liu and Y. Zhang, RSC Adv., 2015, 5, 38646–38653 RSC.
- H. Zhen, T. Wang, R. Jia, B. Su and C. Gao, RSC Adv., 2015, 5, 86784–86794 RSC.
- H. Li, L. Peng, Y. Luo and P. Yu, RSC Adv., 2015, 5, 98566–98575 RSC.
- E. Rusen, A. Mocanu, L. C. Nistor, A. Dinescu, I. Calinescu, G. Mustatea, S. I. Voicu, C. Andronescu and A. Diacon, ACS Appl. Mater. Interfaces, 2014, 6, 17384–17393 CAS.
- M. Sile-Yuksel, B. Tas, D. Y. Koseoglu-Imer and I. Koyuncu, Desalination, 2014, 347, 120–130 CrossRef CAS.
- L. Tang, K. A. Huynh, M. L. Fleming, M. Larronde-Larretche and K. L. Chen, J. Colloid Interface Sci., 2015, 451, 125–133 CrossRef CAS PubMed.
- J. Yin, Y. Yang, Z. Hu and B. Deng, J. Membr. Sci., 2013, 441, 73–82 CrossRef CAS.
- M. Zhang, R. W. Field and K. Zhang, J. Membr. Sci., 2014, 471, 274–284 CrossRef CAS.
- X. Liu, S. Qi, Y. Li, L. Yang, B. Cao and C. Y. Tang, Water Res., 2013, 47, 3081–3092 CrossRef CAS PubMed.
- Y. Liu, E. Rosenfield, M. Hu and B. Mi, Water Res., 2013, 47, 2949–2958 CrossRef CAS PubMed.
- H. L. Yang, J. C. Lin and C. Huang, Water Res., 2009, 43, 3777–3786 CrossRef CAS PubMed.
- K. Zodrow, L. Brunet, S. Mahendra, D. Li, A. Zhang, Q. Li and P. J. Alvarez, Water Res., 2009, 43, 715–723 CrossRef CAS PubMed.
- L. Tang, K. J. T. Livi and K. L. Chen, Environ. Sci. Technol. Lett., 2015, 2, 59–65 CrossRef CAS.
- J. A. Prince, S. Bhuvana, K. V. K. Boodhoo, V. Anbharasi and G. Singh, J. Membr. Sci., 2014, 454, 538–548 CrossRef CAS.
- Z. Yu, Y. Zhao, B. Gao, X. Liu, L. Jia, F. Zhao and J. Ma, RSC Adv., 2015, 5, 97320–97329 RSC.
- H. Lee, S. M. Dellatore, W. M. Miller and P. B. Messersmith, Science, 2007, 318, 426–430 CrossRef CAS PubMed.
- H.-C. Yang, J. Luo, Y. Lv, P. Shen and Z.-K. Xu, J. Membr. Sci., 2015, 483, 42–59 CrossRef CAS.
- S. Xiong, Y. Wang, J. Yu, L. Chen, J. Zhu and Z. Hu, J. Mater. Chem. A, 2014, 2, 7578–7587 CAS.
- S. Hong, Y. S. Na, S. Choi, I. T. Song, W. Y. Kim and H. Lee, Adv. Funct. Mater., 2012, 22, 4711–4717 CrossRef CAS.
- J. Jiang, L. Zhu, L. Zhu, B. Zhu and Y. Xu, Langmuir, 2011, 27, 14180–14187 CrossRef CAS PubMed.
- J. Liebscher, R. Mrowczynski, H. A. Scheidt, C. Filip, N. D. Hadade, R. Turcu, A. Bende and S. Beck, Langmuir, 2013, 29, 10539–10548 CrossRef CAS PubMed.
- R. A. Zangmeister, T. A. Morris and M. J. Tarlov, Langmuir, 2013, 29, 8619–8628 CrossRef CAS PubMed.
- Z. Dong, D. Wang, X. Liu, X. Pei, L. Chen and J. Jin, J. Mater. Chem. A, 2014, 2, 5034–5040 CAS.
- Q. Ye, F. Zhou and W. Liu, Chem. Soc. Rev., 2011, 40, 4244–4258 RSC.
- D. Yang, M. Tian, D. Li, W. Wang, F. Ge and L. Zhang, J. Mater. Chem. A, 2013, 1, 12276–12284 CAS.
- H. Lee, J. Rho and P. B. Messersmith, Adv. Mater., 2009, 21, 431–434 CrossRef CAS PubMed.
- H.-C. Yang, K.-J. Liao, H. Huang, Q.-Y. Wu, L.-S. Wan and Z.-K. Xu, J. Mater. Chem. A, 2014, 2, 10225–10230 CAS.
- S. Kim and C. B. Park, Langmuir, 2010, 26, 14730–14736 CrossRef CAS PubMed.
- J. Ryu, S. H. Ku, H. Lee and C. B. Park, Adv. Funct. Mater., 2010, 20, 2132–2139 CrossRef CAS.
- L. Zhang, J. Wu, Y. Wang, Y. Long, N. Zhao and J. Xu, J. Am. Chem. Soc., 2012, 134, 9879–9881 CrossRef CAS PubMed.
- M. Lee, S. H. Ku, J. Ryu and C. B. Park, J. Mater. Chem., 2010, 20, 8848 RSC.
- B. Li, W. Liu, Z. Jiang, X. Dong, B. Wang and Y. Zhong, Langmuir, 2009, 25, 7368–7374 CrossRef CAS PubMed.
- X.-l. Li, L.-p. Zhu, J.-h. Jiang, Z. Yi, B.-k. Zhu and Y.-y. Xu, Chin. J. Polym. Sci., 2011, 30, 152–163 CrossRef.
- Q. Liu, B. Huang and A. Huang, J. Mater. Chem. A, 2013, 1, 11970–11974 CAS.
- T. S. Sileika, H. D. Kim, P. Maniak and P. B. Messersmith, ACS Appl. Mater. Interfaces, 2011, 3, 4602–4610 CAS.
- J. Ren, P. Han, H. Wei and L. Jia, ACS Appl. Mater. Interfaces, 2014, 6, 3829–3838 CAS.
- S. M. Kang, N. S. Hwang, J. Yeom, S. Y. Park, P. B. Messersmith, I. S. Choi, R. Langer, D. G. Anderson and H. Lee, Adv. Funct. Mater., 2012, 22, 2949–2955 CrossRef CAS PubMed.
- E. Faure, P. Lecomte, S. Lenoir, C. Vreuls, C. Van De Weerdt, C. Archambeau, J. Martial, C. Jérôme, A.-S. Duwez and C. Detrembleur, J. Mater. Chem., 2011, 21, 7901 RSC.
- D. J. Miller, P. A. Araujo, P. B. Correia, M. M. Ramsey, J. C. Kruithof, M. C. van Loosdrecht, B. D. Freeman, D. R. Paul, M. Whiteley and J. S. Vrouwenvelder, Water Res., 2012, 46, 3737–3753 CrossRef CAS PubMed.
- J. Jiang, L. Zhu, L. Zhu, H. Zhang, B. Zhu and Y. Xu, ACS Appl. Mater. Interfaces, 2013, 5, 12895–12904 CAS.
- H. Qin, H. Cao, Y. Zhao, G. Jin, M. Cheng, J. Wang, Y. Jiang, Z. An, X. Zhang and X. Liu, ACS Appl. Mater. Interfaces, 2015, 7, 10785–10794 CAS.
- Y. Liao, Y. Wang, X. Feng, W. Wang, F. Xu and L. Zhang, Mater. Chem. Phys., 2010, 121, 534–540 CrossRef CAS.
- R.-X. Zhang, L. Braeken, P. Luis, X.-L. Wang and B. Van der Bruggen, J. Membr. Sci., 2013, 437, 179–188 CrossRef CAS.
- Y. Lee and T. G. Park, Langmuir, 2011, 27, 2965–2971 CrossRef CAS PubMed.
- H. Y. Son, J. H. Ryu, H. Lee and Y. S. Nam, Macromol. Mater. Eng., 2013, 298, 547–554 CrossRef CAS.
- W. Lu, S. Gao and J. Wang, J. Phys. Chem. C, 2008, 112, 16792–16800 CAS.
- S. Chang, Z. A. Combs, M. K. Gupta, R. Davis and V. V. Tsukruk, ACS Appl. Mater. Interfaces, 2010, 2, 3333–3339 CAS.
- M. Zhang, P. Wang, H. Sun and Z. Wang, ACS Appl. Mater. Interfaces, 2014, 6, 22108–22115 CAS.
- A. GhavamiNejad, A. Rajan Unnithan, A. Ramachandra Kurup Sasikala, M. Samarikhalaj, R. G. Thomas, Y. Y. Jeong, S. Nasseri, P. Murugesan, D. Wu, C. Hee Park and C. S. Kim, ACS Appl. Mater. Interfaces, 2015, 7, 12176–12183 CAS.
- H. Yang, Y. Lan, W. Zhu, W. Li, D. Xu, J. Cui, D. Shen and G. Li, J. Mater. Chem., 2012, 22, 16994–17001 RSC.
Footnote |
† Electronic supplementary information (ESI) available: SEM cross-section images of the PDA/PES and AgNPs–PDA/PES composite membranes (Fig. S1), TEM images and EDX results of the AgNPs–PDA composite (Fig. S1), comparison of the separation performances of different membranes in the literatures (Table S1), TGA results (Fig. S3) and total amount of the AgNPs for the AgNPs–PDA/PES composite membranes (Table S2), static water contact angles of the PDA/PES and AgNPs–PDA/PES composite membranes (Fig. S4). See DOI: 10.1039/c6ra04458a |
|
This journal is © The Royal Society of Chemistry 2016 |