DOI:
10.1039/C6RA04433F
(Review Article)
RSC Adv., 2016,
6, 46711-46722
Gold nanoparticle-based nanosystems for the colorimetric detection of Hg2+ ion contamination in the environment
Received
18th February 2016
, Accepted 21st April 2016
First published on 26th April 2016
Abstract
This review highlights the impact of heavy-metal contamination on the human population and the need for its detection. Minute amounts of mercury ions present in a soluble form in water enter the life cycle of man and produce an impact to an extent that can even affect the foetus inside the womb. Therefore, detection of heavy-metal contamination in water sources is essential. This review proposes the development of simple gold nanoparticle-based sensors for the visual detection of mercury. Gold nanoparticles that have been modified using various functional units are employed in the detection of Hg2+ contamination. Simple, colorimetric methods have been employed in the detection and the major advantage of these sensors is that they are not only simple but also very effective. As the presence/absence of Hg2+ contamination can be detected using the naked eye, these sensors are furthermore user-friendly and, above all, very cheap when compared to other methods that employ the use of highly sophisticated and costly instruments.
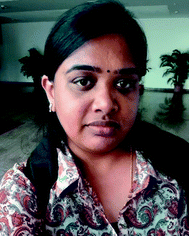 Velswamy Poornima | Miss Velswamy Poornima is a woman scientist fellow at the Biological Materials Lab, Central Leather Research Institute (CSIR-CLRI), Chennai, India. She received her BSc from Bharathidasan University and procured her MTech in Nanoscience and Nanotechnology (with distinction) from the University of Madras, Chennai, India. Her main research areas include the development of noble metal nanomaterials for environmental applications, and semiconducting quantum dots for biological imaging. Her current focus is on the development of simple nanomaterial-based sensors for the detection of low dose ionizing radiation. |
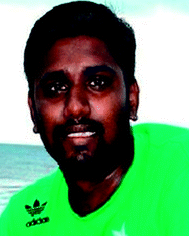 Vincent Alexandar | Mr Vincent Alexandar is currently working as a research fellow at Chettinad University, Chennai. He obtained his BSc (with distinction) from Bangalore University, India and secured first rank in the university level examination in his MSc (Gene Technology) at the BDU, Trichy, India. His current areas of research include molecular medicine and systems biology. He works on interdisciplinary fields and is involved in the development of paper-based microfluidic sensors. He has also developed a multiomic database for major cardiovascular disease (Cardiogenbase) under a single portal which is the first of its kind. |
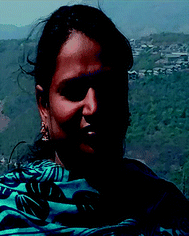 S. Iswariya | Mrs S. Iswariya is a woman scientist fellow at the Biological Materials Laboratory, Central Leather Research Institute (CSIR-CLRI), Chennai, India. She passed her MSc in Microbiology and MPhil in Biotechnology with first class honours and distinction from BDU, Trichy, India. Her prime interests remain in the isolation and extraction of collagen from marine sources. Her skill levels are very high with respect to various chromatographic techniques. She specializes in the development of hydrogels from polymers. Her inquisitive knowledge on plants has driven her work towards the green synthesis of nanomaterials and exploring their biomedical applications. |
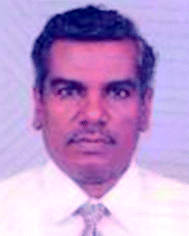 Paramasivan T. Perumal | Dr P T. Perumal is a Chief Scientist at the Dept. of Organic Chemistry in CLRI and he is in charge of 25 scientific staff and researchers. His work mainly focuses on the synthesis of heterocyclic compounds using Inverse Electron Demand Diels–Alder (IED), using Vilsmeier reagent, multi component reactions, acylation, alkylation and oxidation reactions. He is also studying the green chemistry approach to the synthesis of heterocyclic compounds by ionic liquid mediated reactions; solvent free reactions; microwave assisted reactions and water mediated reactions. He has a number of publications in highly cited journals. |
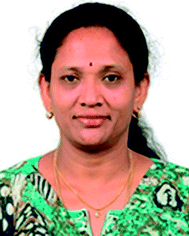 Tiruchirappalli Sivagnanam Uma | Dr T. S. Uma is a scientist at the Biological Materials Lab, CSIR-CLRI, Chennai. Her work areas include the exploitation of microbial technology for the development of biomaterials and biosensors. Her group possess expertise in electrospun nanofibers for their application in wound healing. Her current research includes the development of inorganic nanomaterial-based sensors for dosimetric studies and layered double hydroxide materials for drug delivery applications. She has a number of publications in peer reviewed journals and has written two book chapters. She currently has 8 students in her team who are working on highly interdisciplinary fields. |
1. Introduction
The human environment is constantly being subjected to various pollutants that are hazardous to its flora and fauna. The majority of these pollutants are poisonous in nature and come from both natural and manmade sources. One such harmful material which is found to cause a major environmental threat is heavy-metal contamination. Heavy metals are high-density metals and are divided into two types, namely, essential and nonessential metals. Nonessential heavy metals are those that pose a serious toxic threat to the surroundings even at very low concentrations.1 Major sources of heavy-metal contamination in the environment are broadly classified into the following six categories – natural, agricultural, industrial, domestic effluents, atmospheric, and other sources. Volcanic eruptions, marine aerosols, forest fires and wind-blown dust contribute to the natural sources of heavy-metal contamination. Agricultural sources include those from fertilizers, insecticides, and pesticides. Contaminants from mining operations, ore refining, sludge disposal, radioactive paint processing, untreated water, waste water passed over sewage, etc. contribute to the heavy-metal contamination from industrial and domestic sources.2 Other sources include those from anthropogenic sources such as fly ash from soil, incinerations, material processing and paint alloys.3 The major concern here is mercury ion toxicity as this is highly soluble in water and is the most toxic among all of the nonessential heavy metals. Two-thirds of mercury contamination occurs naturally while the remaining one-third is contributed by mankind.4 Mercury, present in all of the four spheres of Earth, is a major area of concern as environmental pollution spreads on a global scale.5 The current situation demands the need for the development of easier and faster methods for detection of mercury contamination. Gold nanoparticles, with their excellent tunable optical properties, play a vital role in fulfilling this purpose. Modified gold nanoparticles are employed for the detection of mercury contamination by colorimetric methods that can be seen with the naked eye. This is by far the simplest and quickest among the various methods devised for the detection of mercury contamination.
2. Mercury toxicity
2.1. Sources and major health problems
Mercury toxicants are found in the ground, rainwater and seawater. Inorganic mercury present in the air settles down into the aquatic system where it is converted into lipophilic, organic MeHg which becomes interlaced into the aquatic ecosystem. Alongside the damage incurred, MeHg accumulates in meat and vegetables. Mercury has an ease of access to mankind through various pathways such as air, water and food and also vaccines.6 The high solubility of mercury in water makes it easily available to mankind by entering the food chain via fish for example, resulting in major medical complications to the human population. Mercury originating from volcanic eruptions enters nearby water resources. Due to its high solubility, it remains dissolved in water thus entering into the aquatic ecosystem. Algal bodies and plants surrounding the water medium are found to accumulate dissolved mercury. This absorbed mercury interferes with plant metabolism thereby hindering photosynthesis and causing a decrease in the total chlorophyll content of plants. It also inhibits algal growth. Mercury content present within plants/algae is a direct function of the extent of contamination of the water body.
Methyl mercury obtained from the bacterial conversion of inorganic mercury is highly soluble in water and cannot be separated thus leading to bio-magnification. It gets accumulated in fish and, when ingested, enters the human system and causes various medical complications, including central nervous system damage to the foetus present inside the womb as shown in Fig. 1. Development of the central nervous system takes place right from the embryo stage and continues until the child reaches adolescence. In such cases, exposure to even minute quantities of mercury toxicant might lead to a number of disorders such as motor dysfunction, nervous disorder and loss of verbal memory. It also causes neurological, nephrological, immunological, cardiac and reproductive disorders. As it is able to cross the blood–brain barrier due to it highly lipophilic nature, mercury may also cause genetic disorders in an individual and is found to be one of the major factors leading to Alzheimer's and Parkinson's disease, and Amyotrophic Lateral Sclerosis (ALS). Mercury contamination occurs via various means such as by inhaling mercury vapors from the atmosphere, ingesting water and food contaminated with mercury and through atopic applications.7 Consumption of methyl mercury-contaminated fish is a major source of mercury poisoning in humans. The harmful effects arising due to the consumption of heavy metals lead to what is known as the “bio-toxic effect” which could be acute, chronic or sub-chronic.8 Mercury penetrates the blood, accumulates in erythrocytes, and causes severe damage to various organ systems.
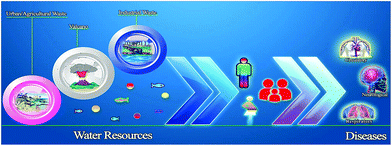 |
| Fig. 1 Graphical representation of methyl mercury poisoning in humans. | |
Different forms of mercury affect the human system in different ways as depicted in Table 1. Higher levels of MeHg in cord blood compared to those in maternal blood is proof that MeHg crosses the placental barrier.9 Foetal methyl mercury poisoning affects the foetus resulting in brain damage, tremors and seizures to the developing foetus.7
Table 1 Effect of elemental, inorganic and methyl mercury poisoning in the human population
Mercury |
Effect |
References |
Acute effects |
Elemental |
CNS effect: tremors, irritability, insomnia, memory loss, neuromuscular changes, headaches, slowed sensory and motor nerve function, and reduction in cognitive function |
9 and 10 |
Kidney effects: acute renal failure |
Gastrointestinal effects and respiratory effects: chest pains, dyspnea, cough, pulmonary function impairment, and interstitial pneumonitis |
Inorganic |
Acute oral exposure to inorganic mercury compounds includes a metallic taste in the mouth, nausea, vomiting, and severe abdominal pain in humans |
9–11 |
Methyl mercury |
CNS effects including blindness, deafness, and impaired level of consciousness in humans |
9–12 |
![[thin space (1/6-em)]](https://www.rsc.org/images/entities/char_2009.gif) |
Chronic effects |
Elemental |
CNS effects: erethism (increased excitability), irritability, excessive shyness, insomnia, severe salivation, gingivitis, and tremors |
9, 10 and 13 |
Acrodynia syndrome: severe leg cramps, irritability, paresthesia (a sensation of prickling on the skin), painful pink fingers and peeling hands, feet, and nose |
9 and 10 |
Inorganic |
Kidney damage |
9–11, 13 and 14 |
Mercury-induced autoimmune glomerulonephritis (induction of an immune response to the body's kidney tissue) in humans |
Acrodynia syndrome |
Methyl mercury |
CNS effects: paresthesia, blurred vision, and malaise. Effects at higher doses include deafness, speech difficulties, and constriction of the visual field |
9, 10 and 12 |
Neurologic abnormalities in human infants |
15 |
![[thin space (1/6-em)]](https://www.rsc.org/images/entities/char_2009.gif) |
Reproductive/developmental effects |
Elemental |
Miscarriages, spontaneous abortions, birth defects |
9 and 13 |
Inorganic |
Alterations in testicular tissue, abnormalities of development |
9, 13 and 16 |
Methyl mercury |
CNS effect: mental retardation, ataxia, and deafness, constriction of the visual field, blindness, and cerebral palsy. At lower methyl mercury concentrations, developmental delays and abnormal reflexes |
9, 10 and 12 |
![[thin space (1/6-em)]](https://www.rsc.org/images/entities/char_2009.gif) |
Cancer risk |
Elemental |
Cancer in humans |
9, 10 and 13 |
Inorganic |
Forestomach and thyroid tumors in rats |
16 |
An increased incidence of renal tumors in mice |
Carcinogenicity in rats and mice |
Methyl mercury |
Carcinogenic effects of methyl mercury in humans, and renal tumors in mice |
15 |
2.2. Need for detection of mercury contamination
Mercury contamination is found to be elevated among people consuming large amounts of food originating from the aqueous environment. The general symptoms of mercury contamination in animals include weight loss, loss of appetite and laziness. Furthermore, mercury is known to cause a reduction in the reproductive rate among contaminated fishes, thus leading to embryogenicity and teratogenicity.10 High concentrations of mercury prevent mothers from becoming pregnant, and even if they do, it results in a stillborn baby. Lower doses of mercury may result in the birth of a live baby, but with serious neurological symptoms.11 Prenatal exposure to organic mercury results in extreme neurologic disorders such as autism among children. Autoimmune disorders are known to be caused by low dose contamination. Alongside acute doses of mercury, a very low dose of the toxicant in water has been found to cause adverse health effects, which have created the need for the development of new methods for the detection of mercury contamination and to create a sense of environmental awareness among the public. Nanotechnology has led to the development of a novel, simple method for the detection of methyl mercury contamination in water.
A literature search on ISI web of science using the keywords MERCURY and GOLD NANOPARTICLE and DETECTION in the title search returned around 218 publications. Fig. 2 represents the number of publications on the colorimetric detection of mercury using gold from 2000 to January 2016. The count is seen to be gradually increasing indicating the demand for mercury detection and stressing its importance.
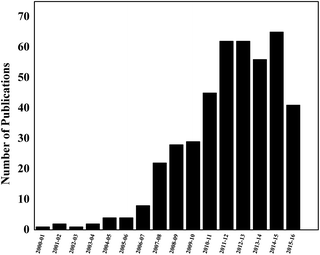 |
| Fig. 2 Yearwise statistics for the colorimetric detection of mercury ions using AuNPs (2001–2016). | |
2.3. Nanosensors for detection of mercury contamination
Drastic effects in the environment due to mercury pollution have created the demand for the development of highly sensitive and selective methods for the detection of mercury.12 Traditional methods, such as atomic absorption spectroscopy,13 inductively coupled-plasma mass spectroscopy (ICPS) and fluorescence spectroscopy14 require complex sample preparation methods and are very expensive as they involve the use of highly sophisticated and bulky equipment.15 Moreover, they lack sensitivity which has led to the emergence of new analytical methods16 based upon the use of molecular fluorophores, DNA, polymers, proteins,17 organic dyes and oligonucleotides.18 All of the aforementioned methods follow simple sample preparation procedures and do not involve the use of expensive equipment.19 Though they have the convenience of being used for the analysis of water samples, they lack sensitivity and selectivity owing to their poor solubility and cross sensitivity.20 Though fluorescence sensors are found to have a better selectivity, they present the problem of fluorescence quenching and also require organic solvent systems for the detection of heavy metals.21 As an alternative, solution-based optical probes were developed that could surpass the drawbacks of traditional methods22 for use in food analysis, environmental sciences and in biomedical assays.23 These colorimetric sensors have an added advantage that they can be visualized with the naked eye24 and are found to possess the requirements of next-generation sensors with the advantage of being cost effective, simple and quick with the possibility of in-field detection.25 Optical readouts are carried out in the solution phase and are helpful in overcoming the difficulties faced with template-based methods.26 Among the various colorimetric tools available, metallic nanoparticles are found to be very effective in biological sensing.27 Metallic gold, especially in its nano form, is found to be an efficient tool for the colorimetrical sensing of not only mercury but also other heavy metals.28
3. Gold nanoparticles and surface plasmon resonance
Among the various types of sensor available, Surface Plasmon Resonance (SPR)-based optical sensors have their own benefits and are based on label-free detection.29 SPR is defined as the collective oscillation of electrons, which is dependent on both the nature and size of the material.30 The extreme sensitivity of SPR to slight changes in the refractive index compared with the medium surrounding the particle is exploited for highly sensitive detection and quantification of the analyte using absorption spectrometry.31 Change in the mass concentration is also dependent on the molecular weight of the analyte.32 As it is not advisable to present the metal surface directly to the analyte, surface functionalization is performed to facilitate the molecular reaction between the target and sensor.33 SPR mainly focuses upon the development of new methodologies in combination with various techniques for biomolecular interaction analysis.34 Gold nanoparticles possess unique size- and shape-dependent properties, which are highly significant and are attributed to their localized surface plasmon resonance (LSPR), a condition wherein their electrons exhibit a collective oscillation upon exposure to electromagnetic radiation.35 This review deals with the different methods employed for the detection of mercury contamination and also the limits of detection for various techniques, the highlight being that each of the techniques employed leads to the colorimetric detection of mercury with the naked eye.
3.1. Synthesis and characterization of gold nanoparticles
An acidic solution of 0.01 M tetrachloro auric acid was prepared by dissolving HAuCl4 in dilute acetic acid. Gold nanoparticles (AuNPs) with different capping agents were prepared by adding the different capping and reducing agents (NaBH4, chitosan, and trisodium citrate) to 10 mL of the gold chloride solution under optimal temperature conditions. The reaction was carried out until the color of the gold solution turned wine red. Table 2 provides details of the temperature conditions maintained for the different reducing agents employed in the reduction of HAuCl4. The as-prepared gold nanoparticles were analyzed using UV-Vis spectroscopy. The results obtained confirmed the formation of gold nanoparticles with size < 20 nm. These gold nanoparticles are modified variously for the detection of mercury contamination. The increase in extinction with reaction time reveals the formation of an increasing population of gold nanoparticles. The nanoscale electronic effect known as the SPR effect causes metallic nanoparticles to absorb and scatter electromagnetic radiation of wavelengths considerably larger than the particles themselves. These effects are particularly noticeable in the visible part of the spectrum for gold. The optical absorbance spectrum of gold nanoparticles is a good indicator of size and shape.36 In preliminary studies, no transparent or color variation occurred in the mixture of gold solution with a chitosan acidic solution. At the same time, no distinct absorption peak of gold nanoparticles occurred on the UV-Vis spectra. According to the literature, the distinct absorption peak from the surface plasmon absorption of the gold nanoparticles is located between 510 and 530 nm. UV-Vis absorption peaks obtained were well within this range and indicate the formation of gold nanoparticles in the size range <20 nm.
Table 2 Synthesis of gold nanoparticles using different capping and reducing agents
Sample no. |
Reducing agent |
Capping agent |
T (°C) |
Time (min) |
1 |
NaBH4 |
Uncapped |
RT |
60 |
2 |
NaBH4 |
Chitosan |
RT |
30 |
3 |
Chitosan |
Chitosan |
Boiling |
30 |
4 |
TSC |
Uncapped |
Boiling |
30 |
Fig. 3 shows transmission electron microscopic (TEM) images of the gold nanoparticles synthesized using different capping and reducing agents. Fig. 4 shows UV-Vis spectroscopic images of gold nanoparticles. All the results confirmed that the size of the nanoparticles formed was less than 20 nm.
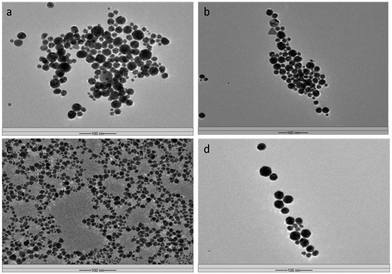 |
| Fig. 3 TEM images of (a) TSC reduced, (b) NaBH4 reduced, (c) NaBH4 reduced chitosan capped, and (d) chitosan capped and reduced gold nanoparticles. | |
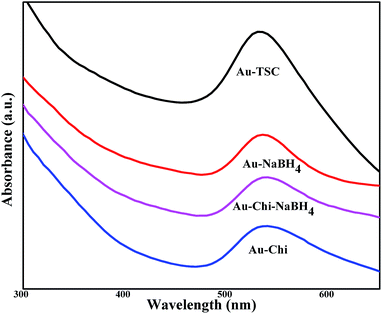 |
| Fig. 4 UV-Vis spectroscopy of AuNPs prepared using different reducing and capping agents. | |
3.2. Modification of gold nanoparticles for the detection of mercury
The as-prepared gold nanoparticles are modified/functionalized in various ways for the detection/quantification of methyl mercury. Tables 3 and 4 list the materials used in the functionalization of the gold nanoparticles, the mechanism of functionalization and the limits of detection achieved. Fig. 5 shows the various agents used in the detection, and the limits of detections achieved. All of the detection methods followed the same mechanism of visual inspection for detection. The limits of detection (LOD) achieved using different moieties varied with the moiety used and are discussed below in detail.
Table 3 Different modifying units and the methods of modification of gold nanoparticles
Sample no. |
Type of AuNP |
Modifying unit |
Mechanism of modification |
Reference |
1 |
Turkevich method |
Probe A-Au-3′S-ATGCTCAACTCT5′ |
Simple wet chemical synthesis at room temperature by coordination chemistry |
12 |
Probe B-Au-5′S-CGCATTCAGGAT3′ |
Probe C-oligonucleotide linker |
2 |
Reduction by NaBH4 |
Thymine acetamidoethanethiol |
Thymine-1-ylacetic acid with cysteamine |
13 |
3 |
Nanogold aptamer |
Single-stranded DNA (ssDNA) |
Self-assembly of the aptamer by catalytic reaction |
16 |
4 |
Gold chromophore |
Thiourea |
Desulfurization reaction of thiourea with AuNP |
18 |
5 |
AA stabilized AuNP |
Glycine |
Photochemical synthesis by Irgacure 2959 |
35 |
6 |
DTET–AuNPs |
Dithioerythritol |
Ligand exchange interaction |
14 |
7 |
Thiol group |
Thiol |
Fixing AuNP onto amine functionalized glass slides |
17 |
8 |
NTA–AuNP |
3-Nitro-1-H-1,2,4-triazole |
Triazole ring of NTA interacts with AuNP surface |
15 |
9 |
Green synthesis |
Chitosan |
By means of core/shell formation |
28 |
10 |
Quantum dot |
Mercury sulfide (HgS) quantum dots |
Formation of HgS QD shell onto AuNP surface |
23 |
11 |
Buffer stabilized |
Tween 20 |
Ion redox modulated surface chemistry |
47 |
12 |
Anti-aggregation |
L-Cysteine |
Wet chemical synthesis |
19 |
13 |
DNA–AuNP |
Mercury-specific DNA |
Incubation in HEPES at room temperature |
37 |
14 |
T–SH–AuNP |
N-1-(2-Mercaptoethyl) thymine |
Ligand exchange interaction |
41 |
15 |
Anti-aggregation |
4,4′-Dipiridyl (DPy) |
Coordination chemistry between AuNP and DPy |
22 |
16 |
Protein–AuNP |
Papain |
Obtained by incubating papain solution in PBS |
29 |
17 |
Peptide AuNP |
NH2–Leu-Aib-Tyr-Ome |
In situ reduction by the carboxylated peptide |
27 |
18 |
Acid stabilized AuNP |
3-Mercaptopropionic acid |
Coordination chemistry |
43 |
19 |
DNA–AuNP |
Probe A: 5′HS-C10-A10-T-A10-3′ |
Through the thiol group present in DNA |
24 |
Probe B: 5′HS-C10-T10-T-T10-3′ |
|
20 |
Amino acid |
L-Cysteine |
Through the S-atom present in L-Cys |
42 |
21 |
Optically modified |
MPA–HCys–PDCA |
Incubating AuNP with MPA–HCys–PDCA |
21 |
22 |
Acid modified |
3-MPA and AMP |
Electrostatic interaction |
45 |
23 |
Naphthol |
1,8-Naphtholimide |
Aqueous phase synthesis |
48 |
24 |
Quaternary ammonium |
Hydrophilic (11-mercapto undecyl)-trimethyl ammonium (MTA) |
Ligand exchange interaction |
44 |
25 |
DNA functionalized |
Polythymine (T33) oligo in Mn2+ |
Mn2+ groups on AuNP bind to backbone of DNA |
38 |
Table 4 Summary of the variously modified gold nanoparticles, their working pH, medium, size, LOD and mode of action of Hg2+
Sample no. |
Size (nm) |
Functionalization |
pH |
Medium |
Detection time (min) |
LOD |
Detection mechanism |
Reference |
1 |
11.7 |
Carboxylated peptide |
2.0 |
Water (RT) |
10 |
200 pM |
Dipole–dipole interaction |
26 |
2 |
13.1 |
Polythymine oligonucleotide |
7.4 |
PBS (RT) |
5 |
10 nM |
Salt induced aggregation |
39 |
3 |
13.3 ± 0.5 |
Mercaptopropionic acid |
9 |
Tris borate buffer (RT) |
60 |
100 nM |
Metal induced aggregation |
43 |
4 |
42 |
Papain |
6 |
Water (RT) |
— |
200 nM |
Metal induced aggregation |
28 |
5 |
— |
DNA |
— |
— |
— |
100 nM |
Thymidine–Hg2+–thymidine coordination chemistry |
24 |
6 |
16 |
L-Cysteine |
— |
Water (RT) |
10–30 |
100 nM |
UV radiation |
42 |
7 |
4 |
MPA–HCys–PDCA |
— |
Water (RT) |
10 |
5 ppb |
Non-linear optical properties |
21 |
8 |
16.0 ± 1.9 |
Dithioerythritol |
6.6 |
PBS (RT) |
10 |
100 nM |
Sulfur–Hg2+–sulfur interaction |
14 |
9 |
100 |
Chemodosimeter |
— |
Water (RT) |
10 |
10 μM |
Scattering property |
47 |
10 |
50 |
1,6-Hexanedithiol |
— |
— |
— |
1–10 nM |
Single-nanoparticle-based metal ion sensing |
17 |
11 |
13.1 |
3-MPA–AMP, R6G/MPA/AMP |
7.4 |
PBS (RT) |
30 |
50–500 nM |
Metal induced aggregation |
43 |
12 |
13 ± 1 |
Tween 20 |
7.9 |
Drinking water and seawater (RT) |
5 |
0.001 nM |
Citrate induced aggregation |
20 |
13 |
13.1 ± 0.5 |
DNA |
— |
Water (RT) |
10 |
60 nM |
T–Hg–T complex induced aggregation |
37 |
14 |
13 |
Tween 20 (1) |
7.2 |
Water (RT) |
30 |
5 nM |
Aggregation |
46 |
15 |
12.2 |
Cysteine |
5.0 |
Drinking water (RT) |
— |
50 nM |
Au S bond – Hg–cys complex induced aggregation |
19 |
16 |
5 ± 2 |
Glycine |
7.5 |
Water (RT) |
— |
10 μM |
Heavy metal induced aggregation |
35 |
17 |
13 |
Adenosine triphosphate |
7.4 |
Sodium borate buffer (RT) |
3 |
193 nM |
Thiourea-induced aggregation |
18 |
18 |
13 |
Thymine |
9.0 |
Sodium borate buffer (45–50 °C) |
10 |
500 nM |
T–Hg–T complex induced aggregation |
13 |
19 |
13 |
Quaternary ammonium |
|
— |
— |
1000 nM |
T–Hg–T mismatch |
44 |
20 |
14–30 |
DNA |
7 |
PBS (RT) |
5 |
10 nM |
T–Hg–T coordination chemistry |
12 |
21 |
— |
N-1-(2-MPET) |
7.4 |
PBS (RT) |
5 |
2.8 nM |
T–Hg–T complex formation |
41 |
22 |
13 |
4,4′-Dipyridine |
7.0 |
Tris HCl and tap water (RT) |
— |
15 nM |
Anti-aggregation |
22 |
23 |
10 |
sDNA |
— |
Water (60 °C) |
15 |
1.7 pM |
T–Hg–T mismatch |
48 |
24 |
— |
HgS |
3.57 |
Water (RT) |
90 |
0.5 nM |
SPR |
23 |
25 |
— |
Chitosan |
7 |
— (RT) |
— |
5 pM |
SPR |
27 |
26 |
∼13 |
Nitrotriazole |
8.0 |
Tris buffer (RT) |
60 |
7 nM |
Salt induced anti aggregation |
15 |
27 |
13.1 ± 4 |
Citrate |
— |
Lake water |
— |
200 nM |
Salt induced anti aggregation |
40 |
28 |
13 |
DNA |
— |
Tris–acetate |
20 |
10 nM |
Hg2+–bis-thymine complex |
38 |
29 |
13 |
Polythymine DNA |
— |
50 mM NaCl |
— |
10 fM |
T–Hg2+–T coordination |
— |
30 |
13 |
DNA |
7.4 |
Tris–HCl buffer |
— |
1 nM |
Hg2+–DNA complex |
— |
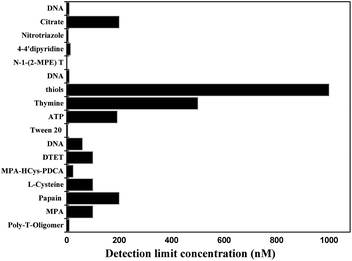 |
| Fig. 5 Different functionalization units vs. detection limit concentration. | |
3.3. Methods of modification of gold nanoparticles
A number of methods have been devised for the functionalization of gold nanoparticles. The method of functionalization depends on the end application of the as-prepared nanoparticles. For colorimetric detection of mercury, gold nanoparticles have been chosen as the appropriate nanomaterial as they possess SPR, which is an important attribute for these kinds of color changing reactions. Functional units have been designed such that they increase the reactivity between the AuNPs and Hg2+ ions when present in the solution. Single-stranded DNA with simple modifications is found to have an increased affinity for gold nanoparticles. DNA modification of AuNPs is achieved by a simple wet chemical method. A similar method is adapted for amino acid functionalization. Esters, polythiols, nitrazole, carboxylic acids and other such groups are attached onto the surface of the gold nanoparticles which are synthesized using different capping and reducing agents. Though simple methods are adapted for the modification of gold nanoparticles, a few precautionary steps must be considered while functionalizing the AuNPs such that the integrity of the gold nanoparticles is not affected. These modifying units are found to enhance the sensitivity of the system they are employed in. Table 3 gives the different mechanisms that are involved in the functionalization of gold nanoparticles and the various functionalization units that are discussed during the review. One major point of concern while choosing the functionalization unit is to check that it does not disturb the dispersivity of the gold nanoparticles. In addition, it should be capable of inducing aggregation with the introduction of the analyte (Hg2+), thereby bringing about a color change in the solution. In addition, there are methods that employ anti-aggregation of the gold nanoparticles, but the rule of thumb remains the same, i.e. the capacity to bring about color change, which makes visual detection possible. The color change can be from red to blue (red shift) or its reverse (blue shift). Thus, a qualitative detection is achieved by the naked eye while quantitative detection can be achieved using a sophisticated instrument such as UV-Vis spectrophotometer.
4. Methods of detection of mercury
4.1. DNA
Upon addition to a solution of methyl mercury, DNA oligonucleotide-functionalized gold nanoparticles showed a sharp bathochromic shift from 520 nm to 556 nm with a change in the colour of the solution from wine red to dark purple due to the conversion of MeHg to Hg2+ and due to the aggregation of the gold nanoparticles. The change in colour was visible to the naked eye, and the aggregation was confirmed using transmission electron microscopy.12 Mercury-specific DNA modified AuNP colorimetric probe brought about a detection of mercury of concentration 60 nM in edible fish, which was very much lower than the toxicity level determined by the U.S. Environmental Protection Agency (EPA).36 Itamar et al. devised an oligonucleotide-based method for the colorimetric detection of Hg. The method was based upon the formation of a hairpin bend wherein the spatially separated T residues are linked to Hg2+ leading to the formation of a stiff structure resulting in the aggregation of gold nanoparticles. The LOD of this method was found to be 10 nM.37 Polythymine oligonucleotide functionalized citrate-capped AuNPs brought about the detection of Hg2+ (10 nM) in the presence of Mn2+ ions. When the number of thymine residues was >33, the aggregation was found to take place within 6 min.38 The LOD achieved by this method was 100 nM.24 Single strand DNA modified nanogold probe with its unique optical and electrical properties is considered to be one of the best nanoparticles for nanoanalytical chemistry. The nanogold ssDNA (NGssDNA) probe is employed for the detection of Hg2+ based on the change in the resonant scattering spectra brought about by the aggregation of NGssDNA upon the addition of mercuric ions. The method is based upon the detection of change in the solution color owing to DNA–AuNP aggregation. The major advantage of this method is that it enables the assay to be carried out in aqueous media without the need for other organic co-solvents.
The fundamental principle for the detection of other heavy-metal ions using DNA is achieved by imparting slight modifications in the system and quantification is also achieved by studying the change in DNA melting temperature (Tm). Gold nanoparticles modified with a 15-mer-thrombin binding sensing element were developed for salt-induced gold nanoparticle aggregation. The aptamer molecule consisted of six thymidine and nine guanosine units that interact in a specific manner to form a hairpin-like or a quadruplex structure. In addition to Hg2+ sensing, these AuNP reporting probes can also be used to characterize conformational changes in an oligonucleotide.39 The presence of thiol modification of the surface of gold nanoparticles enhanced the adherence of Hg2+ ions on its surface. Hence, thymidine-modified AuNPs were effective for the detection of Hg2+.
4.2. Amino acids
Amino acid modified gold nanoparticles are found to be highly sensitive in the detection of mercury. Owing to the cheap availability of amino acids, thymine-based derivatives are used for the detection of Hg2+ ions. Thymine acetamido ethanethiol (T–SH) is designed as a recognition unit for the detection of Hg2+. The combined presence of T–SH and Hg2+ brought about the aggregation of gold nanoparticles producing a shift in the absorption spectrum from 520 nm to 660 nm with the limit of detection being 50 nM.13 A mercaptoethyl modified thymine functionalized gold nanoparticle probe had a detection limit of 2.8 nM.40
L-Cysteine modified AuNPs had a LOD of 100 nM.41 Water dispersible, glycine-capped gold nanoparticles are unique in the determination of mercury. The presence of mercury is confirmed by the color change in the solution which is brought about by the aggregation of glycine-capped gold nanoparticles in the presence of metal. The anionic stabilized gold nanoparticle could achieve a sensitivity of 10 μM.35 The presence of a thiol moiety in cysteine enables the facile binding of gold nanoparticles to the amino acid via gold–thiol bonding. The major advantage of the sulphur group is the facile binding of Hg2+ to it. The presence of mercury brings about the aggregation of gold nanoparticles through interparticle cross-linking. Zhang et al. achieved a detection limit of 50 nM.19 Gold nanoparticles modified using carboxylated peptides brought about detection limits as low as 200 pM wherein the N-terminus amino group is anchored to the AuNP and the C-terminus carboxyl group is left available for binding with Hg2+. The major advantage of this method is that the detection time is only 5 min. As compared with other methods of detection, peptides are highly biocompatible and hence can be used for other biotechnological applications.
4.3. Acids, esters and polythiols
The thiophilic nature of Hg2+ has attracted scientists to explore the use of thiol groups for the detection of mercury. Based on this, dithioerythritol (DTET) functionalized gold nanoparticles have been employed for the detection of mercury. The basic mechanism upon which this system works is through the sulphur–Hg2+–sulphur interaction between the DTET-modified gold nanoparticles. The major principle underlying the system is based on Mie Theory, which states that when the interparticle distance between two nanoparticles becomes less than the sum of their radii, this brings about a red shift in the spectrum along with peak broadening and a decrease in the intensity. DTET–AuNP can be used in the detection of Hg2+ with concentrations >100 nM.14 Mercaptopropionic acid modified gold nanoparticles aid in the highly sensitive detection of Hg2+ ions in the presence of 2,6-pyridine carboxylic acid (PDCA). The ionic strength of the solution used plays a crucial role in the detection of heavy-metal ions. The pH of the solution can be tuned by varying the buffer composition and henceforth the surface charge density can also be controlled. MPA ligand density on MPA–AuNP, cooperativity of PDCA and the buffer composite aid in the selectivity and sensitivity of the system. This low-cost system has a limit of detection of 100 nM and is believed to lay the foundation for the development of nanosensors which could be employed for the detection of Hg2+ in both biological and environmental samples.42 For the better assessment of human health and environmental safety, efforts have been made to improve the detection of mercury in water and other resources. The 1,6-hexane thiol modified gold nanoparticle system for the detection of Hg2+ is based upon the shift in the localized surface plasmon resonance (LSPR) of the individual gold nanoparticles owing to the binding of analyte to the gold nanoparticles. The shift across the wavelength spectrum of an individual nanoparticle was tracked with the help of a LSPR-induced scattering spectrum using a dark field microspectroscopy (DFMS) system. Using this method, it was possible to reach sensitivity levels as low as 100 pM.17 A cost-effective system based on the second-order nonlinear optics (NLO) property of the gold nanoparticles has been designed in order to achieve a gold nanoparticle-based colorimetric detection of mercuric ions, which does not involve the complex tagging and labelling of AuNPs which in turn proved to be very costly. The precision of the system based on NLO is found to be around 5 ppb. The hyper-Rayleigh scattering (HRS) technique has been employed in the monitoring of NLO properties. As the system does not involve the application of an electrostatic field and phase matching, it can be used for a wide range of systems. Herein, gold nanoparticles modified using MPA, homocysteine (HCys) and PDCA are used for the detection of Hg2+ and other heavy-metal ions. The major advantage of this method is that it is completely label-free and HRS assay does not involve the use of any fluorescent probe. In addition, it is highly selective, and it takes a maximum of only 7 min for the detection of mercury contamination.21
Adenosine monophosphate (AMP) and MPA modified AuNPs are capable of detecting Hg2+ ions at concentrations as low as 500 nM. High negative charge density on the surface of MPA/AMP modified gold nanoparticles enables the complete dissolution in a high salt solution. Aggregation of nanoparticles in the presence of mercury is by the coordination between the carboxyl group of MPA and Hg2+, which also renders it highly selective in a salt solution. Another major reason for the aggregation of these particles is due to the reduced electrostatic repulsion between the particles that arises due to a decreased zeta potential on each AuNP. It is found that the sensitivity of the system depends on the concentration of AMP and the optimal molar concentration of AMP to MPA is 1
:
500. This molar ratio of AMP to MPA used during the synthesis of AuNP, brings about the visual color change from red to purple upon exposure to Hg2+. The sensitivity of the system was further improved to 50 nM by the addition of a fluorescent tag rhodamine 6G (R6G) to this MPA/AMP-capped AuNP.43 Quaternary ammonium group terminated thiol-capped AuNPs (QA–AuNP) are used in the solar irradiation assisted detection of Hg2+ in aqueous media at room temperature. This method does not make use of any masking agents and possesses a dynamic detection range from 30 nM to 0.01 M. The principle behind this system is the abstraction of Hg2+ that brings about the aggregation of AuNPs. It is a well-known fact that Hg2+ possesses a greater affinity towards thiol groups. Thus, the thiolate groups attached on the surface of QA–AuNP are removed by the affinity of mercury thereby bringing about a destabilization of the solution finally resulting in aggregation.44
Herein, hydrophilic (11-mercapto-undecyl)-trimethyl ammonium is used as the QA-terminated thiol. The high positive charge at the surface of the QA ions renders them highly stable in acidic solution due to the electrostatic force of repulsion between the cations and the positive charge of H+ ions. Whereas there occurs a strong interaction between these cations and the OH− ions in basic solution resulting in aggregation, in the presence of Hg2+, the greater affinity of mercury to thiol groups displaces the H+ ions and brings about the chemisorption of Hg2+ onto the thiol groups thereby producing a change in the absorption spectra with a red to purple colour change. This method of detection could be used as a better platform for the lab on chip development and the LOD using this method was 30 nM. The major advantage of this method is that it does not require any sophisticated instrumentation. Adenosine triphosphate (ATP) modified gold nanoparticles (sAuNP) have been found to be stable over various pH ranges and result in an immediate aggregation when present in a Hg2+ environment. Concentrations as low as 193 nM could be detected using a sAuNP-based chemosensor.18
4.4. Nitro group
Nitrogen atoms have a significant affinity towards gold atoms. Nitrotriazole groups are used for the detection of mercury. 3-Nitro-1H-1,2,4-triazole (NTA) functionalized gold nanoparticles are used in the detection of mercury with sensitivity levels as low as 7 nM. Capping of AuNPs with NTA is achieved by the strong affinity of nitrogen atoms in the triazole ring for the gold nanoparticle surface. Coordination chemistry between the NTA moieties and Hg2+ ions enabled the development of NTA capped AuNPs as a suitable probe for the detection of heavy-metal contamination. As described previously, the sensing modality is primarily through colorimetric changes and is envisioned through the release of NTA from the AuNPs in the presence of Hg2+ ions and its binding to the same leading to the aggregation of AuNPs, thus enabling the detection of mercury. Dynamic ranges for the detection can be achieved by modifying the amounts of NTA in the NTA–AuNP probe. The assay was also used for the detection of other heavy metals in environmental samples.15
4.5. Quantum dots
Mercuric sulphide quantum dots are used in the detection of mercury. The HgS quantum dots are made to form an in situ Au@HgS core–shell nanostructure. Sulphide atoms present in the core–shell structure act as a mono dentate ligand establishing a strong link between the gold and the sulphide group. Thus, upon the addition of mercury, there was a red shift with the increase in absorption intensity. This was aided by the core–shell structure that enhanced the availability of free electrons enhancing the surface plasmon resonance oscillation. The probe which is used for both qualitative and quantitative analysis is capable of detecting mercury levels as low as 0.486 nM.23
4.6. Polymers
The major advantage of using polymers over other materials is their good biocompatibility. Chitosan, a heteropolymer, is nontoxic in nature and possesses biocompatibility, biodegradability, and antibacterial properties. The presence of reactive amino groups in chitosan enables the aggregation of metal ions in solution. Chitosan-capped gold nanoparticles (CH–AuNP) showed a blue shift in the localized surface plasmon resonance (LSPR) with the addition of Hg2+ ions. Interestingly, CH–AuNP showed a blue shift in the plasmon absorption peak while all of the other methods of detection showed a red shift upon addition of Hg2+. The sensitivity of this method was as low as 5 pM, which is the lowest value achieved using the colorimetric method for Hg2+ detection.27
4.7. Aromatic compounds
Though a large number of compounds have been used in the gold nanoparticle-based colorimetric detection of mercury, the use of the aromatic compound 4,4′-dipyridyl (DPy) follows a different mechanism of action for detection. While the other methods of detection are based on the aggregation of gold nanoparticles with the addition of Hg2+, this method follows detection based on anti-aggregation of gold nanoparticles. The three major steps upon which this probe functions rely on the size of the AuNP, the aggregation reagent DPy and upon the anti-aggregation process. Higher sensitivity and selectivity of the probe rely mainly upon the affinity of DPy for Hg2+. This method of sensing has achieved detection limits of about 15 nM and is suitable for both on-site and real-time analysis. Dynamic ranges can be achieved by modifying the amounts of anti-aggregation reagent used.16
4.8. Proteins
Protein functionalized gold nanoparticles are also employed for the detection of heavy-metal contamination. The detection system based on the protein probe depends mainly upon the pH of the system. Papain, an enzyme with seven cysteine residues, is rich in thiol groups and is used in the detection of mercury. It is capable of adhering directly onto the surface of gold nanoparticles. Papain, in the presence of mercury, is found to bring about a modulation of fluorescence. Thus, a system of detection using papain has been designed wherein it brings about a shift in the color from red to blue that could be envisioned with the naked eye. The system was found to have optimum sensitivity at pH 6 and was very stable at pH ≥ 6. Papain–gold nanoparticles (P–AuNPs) were highly stable with no significant aggregation at increasing pH whereas when the pH level reached 6, the presence of mercury brought about the aggregation of P–AuNPs of heavy metal. The system was found to be suitable for real-time analysis of heavy-metal contamination.28
4.9. Surfactants
A novel “blue-to-red” colorimetric strategy for the detection of Hg2+ is based on the ion redox-modulated chemistry of AuNPs. The minimum detectable concentration with this method was 5 nM. This method was based upon the shift in the wavelength from blue to red in contrast with other methods that relied upon the change in colour from red to purple. There are three major advantages to this method. First, it relies on the blue to red shift which could be detected with a greater sensitivity than the red to purplish transition. Second, it does not involve the need for the selection of ion chelating moieties and complicated modification techniques and finally, as mentioned above, it has a very high sensitivity which is evident in its LOD.45 Tween 20 modified citrate stabilized AuNPs are employed for the detection of Hg2+ and also other metal ions. It is found that citrate groups are present at the surface of the gold nanoparticles, and the presence of Tween 20 prevents the particles from becoming aggregated. Removal of Tween 20 resulted in the aggregation of gold nanoparticles. When Hg2+ ions are present in a solution containing Tween 20 modified citrate-capped AuNP, mercury, due to its greater affinity towards AuNPs, brings about the desorption of Tween 20 and its adsorption onto AuNP surfaces thus bringing about an aggregation and henceforth a colour change. It is to be noted that addition of Tween 20 to citrate stabilized AuNP does not bring about any displacements, and remains dispersed throughout the solution. Studies carried out based on the extinction spectra show that the sensitivity and selectivity of the method depend upon the nanoparticle concentration, surfactant chain length and also upon the ionic strength of the solution. Increased concentration of the surfactant brought about a decrease in the nanoparticle aggregation whereas increasing nanoparticle concentration caused an increase in the aggregation of NPs. At the same time, it was found that, in a solution with high ionic strength, the slight electrostatic force of repulsion between the Tween 20 moieties provided a barrier for metal ion-induced aggregation. With a fixed reaction time of 5 min, the limit of detection using this sensor was 100 nM for seawater and 200 nM for drinking water, which is very much in compliance with the minimum levels of Hg2+ in drinking water as given by WHO. It was also found that a proper choice of masking agent would enable the detection of various other metal ions with improved sensitivity.20
4.10. Chemodosimeter
A mercury-specific chemodosimeter (CHD) was developed based on mercury-induced intramolecular guanylation of thiourea attached to 1,8-naphthalimide immobilized onto the surface of gold nanoparticles. CHD with a benzoyl thiourea component was capable of detecting mercury ions with excellent specificity, but the drawback lay in its unstable nature in aqueous media. In order to overcome this hurdle, a new CHD was developed with phenylthiourea substituted in place of benzoyl thiourea. Surfactant-induced modification of the chemosensor without any modification of the basic chemical structure within the sensor molecule was found to have high efficiency and increased dispersivity in aqueous media. Furthermore, the naphthalimide signaling moiety and thiourea moiety enable the selective detection of Hg2+. The CHD-based system of detection has an LOD of 10 μM and it can be used for both qualitative and quantitative detection of not only mercury but also other metals that might be present in environmental samples. Improvements in the design of the chemodosimeter with the proper choice of nanomaterial and suitable surfactant would lead toward the development of new systems for detection with greater accuracy.46
5. Conclusion
Gold nanoparticles are excellent sensing elements in both modified and unmodified forms.47 The major advantage of a gold nanoparticle-based detection system over other conventional methods such as atomic absorption spectroscopy, atomic emission spectroscopy, inductive coupled mass spectroscopy (ICP-MS), ICP-OES, and fluorescence spectroscopy are as follows: heavy-metal contaminants can be detected with the naked eye; the AuNP-based colorimetric system does not involve the use of expensive instruments; AuNP-based systems do not require complicated sample preparation; modification of gold nanoparticles does not involve any difficult methodology and allows the simple detection of moieties, which are visible to the naked eye. Gold nanoparticles are highly biocompatible and can, therefore, be used in detection in biological environments. Among the various functionalization units studied, each unit is found to be efficient in the detection of not only mercury but also other heavy-metal contaminants in both biological and environmental samples. The easily tunable optical properties of gold nanoparticles enable not only the detection but also the quantification of Hg2+ in the sample which is impossible with other systems. Another positive aspect of AuNP-based systems is that they possess very high levels of sensitivity and selectivity, which are not found in other systems. AuNP-based colorimetric systems do not have the problems of poor solubility or cross-sensitivity, which are major drawbacks of other instrument-based methods.
In summary, gold nanoparticle-based colorimetric sensing of heavy-metal ions is an important tool for the detection of environmental contamination. Further modifications of available systems would provide a significant environmental tool without requiring any complex instrumentation. In addition, another noble metal, silver, in its nano form, possesses similar tunable optical properties and is employed in the detection of heavy-metal contamination.48 Under certain conditions, sensor systems based on metallic nanoparticles have stability issues and can become aggregated over time. Silica-capped gold nanoparticles are also used in the stabilisation of the system and are sensitive to low doses of mercury.49 A number of colorimetric sensors have been developed for the detection of heavy-metal contamination in water and other sources. Gold nanoparticles have proved to be a very effective tool for this purpose as the synthesis methods are simple and easy functionalization is achieved.50 Tunable optical properties add to the increased sensitivity of the system.
Acknowledgements
The author, Miss Velswamy Poornima would like to acknowledge the support from Department of Science and Technology (DST), Ministry of India for providing financial assistance in the form of woman scientist scheme-A under grant SR/WOS-A/LS-1211/2014.
References
- L. C. Rai, J. P. Gaur and H. D. Kumar, Biol. Rev., 1981, 56, 99–151 CrossRef CAS.
- S. Shribman, A. Davis, N. Vella and G. Giovannoni, J. Neurol., Neurosurg. Psychiatry, 2013, 84, e2 CrossRef.
- P. C. Nagajyoti, K. D. Lee and T. V. M. Sreekanth, Environ. Chem. Lett., 2010, 8, 199–216 CrossRef CAS.
- M. Patra and A. Sharma, Bot. Rev., 2000, 66, 379–422 CrossRef.
- Z. S. Zhou, S. J. Wang and Z. M. Yang, Chemosphere, 2008, 70, 1500–1509 CrossRef CAS PubMed.
- F. Zahir, S. J. Rizwi, S. K. Haq and R. H. Khan, Environ. Toxicol. Pharmacol., 2005, 20, 351–360 CrossRef CAS PubMed.
- K. a. Graeme and C. V. Pollack, J. Emerg. Med., 1998, 16, 45–56 CrossRef CAS PubMed.
- J. O. Duruibe, M. O. C. Ogwuegbu and J. N. Egwurugwu, Int. J. Phys. Sci., 2007, 2, 112–118 Search PubMed.
- G. Guzzi and C. A. M. La Porta, Toxicology, 2008, 244, 1–12 CrossRef CAS PubMed.
- a. Léonard, P. Jacquet and R. R. Lauwerys, Mutat. Res., 1983, 114, 1–18 Search PubMed.
- S. Nabi, Bull. Environ., Pharmacol. Life Sci., 2014, 3, 272–285 Search PubMed.
- X. Xue, F. Wang and X. Liu, J. Am. Chem. Soc., 2008, 130, 3244–3245 CrossRef CAS PubMed.
- X. Liu, X. Cheng, T. Bing, C. Fang and D. Shangguan, Anal. Sci., 2010, 26, 1169–1172 CrossRef CAS PubMed.
- Y.-R. Kim, R. K. Mahajan, J. S. Kim and H. Kim, ACS Appl. Mater. Interfaces, 2010, 2, 292–295 CAS.
- X. Chen, Y. Zu, H. Xie, A. M. Kemas and Z. Gao, Analyst, 2011, 136, 1690–1696 RSC.
- Z. Jiang, G. Wen, Y. Fan, C. Jiang, Q. Liu, Z. Huang and A. Liang, Talanta, 2010, 80, 1287–1291 CrossRef CAS PubMed.
- H. D. Song, I. Choi, Y. I. Yang, S. Hong, S. Lee, T. Kang and J. Yi, Nanotechnology, 2010, 21, 145501 CrossRef PubMed.
- S. Kim, N. H. Lee, S. H. Seo, M. S. Eom, S. Ahn and M. S. Han, Chem.–Asian J., 2010, 5, 2463–2466 CrossRef CAS PubMed.
- N. Ding, H. Zhao, W. Peng, Y. He, Y. Zhou, L. Yuan and Y. Zhang, Colloids Surf., A, 2012, 395, 161–167 CrossRef CAS.
- C. Y. Lin, C. J. Yu, Y. H. Lin and W. L. Tseng, Anal. Chem., 2010, 82, 6830–6837 CrossRef CAS PubMed.
- G. K. Darbha, a. K. Singh, U. S. Rai, E. Yu, H. T. Yu and P. C. Ray, J. Am. Chem. Soc., 2008, 130, 8038–8043 CrossRef CAS PubMed.
- Y. Li, P. Wu, H. Xu, Z. Zhang and X. Zhong, Talanta, 2011, 84, 508–512 CrossRef CAS PubMed.
- F. Zhang, L. Zeng, C. Yang, J. Xin, H. Wang and A. Wu, Analyst, 2011, 136, 2825–2830 RSC.
- J. S. Lee, M. S. Han and C. a. Mirkin, Angew. Chem., Int. Ed., 2007, 46, 4093–4096 CrossRef CAS PubMed.
- M. R. Knecht and M. Sethi, Anal. Bioanal. Chem., 2009, 394, 33–46 CrossRef CAS PubMed.
- S. Si, A. Kotal and T. K. Mandal, J. Phys. Chem. C, 2007, 111, 1248–1255 CAS.
- C. Radhakumary and K. Sreenivasan, Analyst, 2011, 136, 2959–2962 RSC.
- Y. Guo, Z. Wang, W. Qu, H. Shao and X. Jiang, Biosens. Bioelectron., 2011, 26, 4064–4069 CrossRef CAS PubMed.
- H. N. Daghestani and B. W. Day, Sensors, 2010, 10, 9630–9646 CrossRef CAS PubMed.
- Y. Cho, S. S. Lee and J. H. Jung, Analyst, 2010, 135, 1551–1555 RSC.
- J. M. Walker, Biosensors and Biodetection, 2009, vol. 504 Search PubMed.
- X. Ma, Z. Sheng and L. Jiang, Analyst, 2014, 139, 3365–3368 RSC.
- T. Senapati, D. Senapati, A. K. Singh, Z. Fan, R. Kanchanapally and P. C. Ray, Chem. Commun., 2011, 47, 10326–10328 RSC.
- K. S. Phillips and Q. Cheng, Anal. Bioanal. Chem., 2007, 387, 1831–1840 CrossRef CAS PubMed.
- K. Hamaguchi, H. Kawasaki and R. Arakawa, Colloids Surf., A, 2010, 367, 167–173 CrossRef CAS.
- J. Wu, L. Li, D. Zhu, P. He, Y. Fang and G. Cheng, Anal. Chim. Acta, 2011, 694, 115–119 CrossRef CAS PubMed.
- D. Li, A. Wieckowska and I. Willner, Angew. Chem., 2008, 120, 3991–3995 CrossRef.
- C.-J. Yu, T.-L. Cheng and W.-L. Tseng, Biosens. Bioelectron., 2009, 25, 204–210 CrossRef CAS PubMed.
- Y. Wang, F. Yang and X. Yang, Biosens. Bioelectron., 2010, 25, 1994–1998 CrossRef CAS PubMed.
- L. Chen, T. Lou, C. Yu, Q. Kang and L. Chen, Analyst, 2011, 136, 4770–4773 RSC.
- F. Chai, C. Wang, T. Wang, Z. Ma and Z. Su, Nanotechnology, 2010, 21, 025501 CrossRef PubMed.
- C.-C. Huang and H.-T. Chang, Chem. Commun., 2007, 1215–1217 RSC.
- C. Yu and W. Tseng, Langmuir, 2008, 12717–12722 CrossRef CAS PubMed.
- D. Liu, W. Qu, W. Chen, W. Zhang, Z. Wang and X. Jiang, Anal. Chem., 2010, 82, 9606–9610 CrossRef CAS PubMed.
- T. Lou, Z. Chen, Y. Wang and L. Chen, ACS Appl. Mater. Interfaces, 2011, 3, 1568–1573 CAS.
- B. Leng, L. Zou, J. Jiang and H. Tian, Sens. Actuators, B, 2009, 140, 162–169 CrossRef CAS.
- L. Chen, J. Li and L. Chen, ACS Appl. Mater. Interfaces, 2014, 6, 15897–15904 CAS.
- L. Chen, L. Chen, X. Fu and W. Lu, ACS Appl. Mater. Interfaces, 2013, 5, 284–290 CAS.
- G. Wang, Z. Chen, W. Wang, B. Yan and L. Chen, Analyst, 2011, 136, 174–178 RSC.
- X. Han, Y. Liu and Y. Yin, Nano Lett., 2014, 14, 2466–2470 CrossRef CAS PubMed.
Footnote |
† Both the authors have contributed equally. |
|
This journal is © The Royal Society of Chemistry 2016 |