DOI:
10.1039/C6RA04255D
(Paper)
RSC Adv., 2016,
6, 42899-42910
Live diatoms facing Ag nanoparticles: surface enhanced Raman scattering of bulk cylindrotheca closterium pennate diatoms and of the single cells†
Received
17th February 2016
, Accepted 26th April 2016
First published on 26th April 2016
Abstract
A comprehensive exploratory SERS experiment in conjunction with conventional Raman spectroscopy was conducted to investigate the interface between the silver nanoparticles (AgNPs) and the lightly silicified Cylindrotheca closterium diatoms in vivo both in the bulk cells culture and at the single cell level. The SERS chemisorption mechanism was found dependent on the NPs type and size as the SERS signal showed different signature for different NPs, the common SERS feature being the absence of the conventional Raman signal of carotenoids when microalgae are excited with the 532 nm line. SERS results suggest that the (pre)resonance Raman effect observed in bulk diatoms is no longer dominant in the case of SERS in bulk solution. Detection of polyunsaturated fatty acids was achieved together with specific SERS evidence of carotenoids and chlorophylls. The results are a prerequisite for the further exploration the diatoms fluctuant contribution to the SERS outcome of natural seawater.
Introduction
Cylindrotheca closterium Reimann & Lewin (1964), an epipelic diatom is a dominating species in the micro phytoplankton community of freshly formed mucilaginous aggregates.1 Although its abundance is negligible in the seawater column,1 the mucilaginous aggregates distribution was associated with a higher salinity in the oligotrophic waters. Reviewed, documented results (ref. 1) and the references herein on central Adriatic seawater suggested a relation of the selective accumulation of C. closterium cells when oligotrophic high salinity water inflow was present. The accumulation of mucilaginous matter al large scale comprising sometimes hundreds of square kilometres could drastically hamper the aquaculture activity, affect the seawater quality, induce mortality of sedentary fauna, disturb the plankton community and affect the tourism activity.
The lightly silicified theca of Cylindrotheca fusiformis Reimann and Lewin diatom species was characterised for long.2 Extensive studies3–5 were devoted to various composition determinations in relation with the growing conditions, since the diatoms showed great potential for biodiesel but also for aquaculture farming. Concerning the chemical composition, the cultivated Cylindrotheca closterium showed the palmitoleic acid (omega-7 monounsaturated fatty acid) as the dominant fatty acid, followed by oleic acid, whereas the most abundant saturated fatty acids were pentadecanoic and palmitic acid.3 Other studies highlighted the differences in chemical composition of 60 clones of Cylindrotheca fusiformis isolated and cultivated under batch conditions.4 Isolated and purified from colony selection and subsequent dilution, Cylindrotheca closterium showed relatively high in lipid content (20.08% ± 0.67% of dry weight) and the combined amount of its 16
:
0 and 16
:
1 (n-7), suggested a significant resource of biodiesel producer, with 64% of the total fatty acids, while the amount of polyunsaturated fatty acids reached 19.96–20% of the total fatty acids.5 A comparative study of the lipid content and fatty acid profile of Cylindrotheca closterium and C. fusiformis in relation with the nutrients balance showed many similar features, being lipid rich (∼25% of the total fatty acids) with high EPA (eicosapentaenoic acid) content.6 Lipid components represented 18–27% of dry biomass in the medium with NaNO3 and among total fatty acids, polyunsaturated fatty acids constituted <40%, eicosapentaenoic acid 25%, and arachidonic acid ∼8% and ∼4% in C. fusiformis and C. closterium, respectively.16
So far, the available studies on diatoms composition rely on extraction and separation techniques. Extensive studies were focussed on the thecae or frustule of various diatoms species2,7–9 by electron microscopy or atomic force microscopy (AFM) techniques, showing that every part of the silica shell is tightly enclosed by organic material while the whole body is surrounded by mucilage material. Moreover, a first approach to understand the silver nanoparticles uptake by the Cylindrotheca diatoms employed AFM8 and found that the AgNPs penetrate the cell wall through the silica valve region as NPs embedded in organic matrix. The AgNPs caused a local damage inside the cell without destroying the cell membrane.8 Furthermore, it was suggested that the extracellular polymeric matter (EPM) secretion was stimulated by the presence of nanoparticles8 in a defense mechanism.
The chemical composition of the algal bodies and their secreted substances usually requires extraction and separation techniques with the main disadvantages of multiprocessing steps and chemicals needed, being relatively expensive and time consuming. Additionally, the inherent destructive steps required by these techniques may reflect the metabolic stress conditions and not the normal living properties.
Raman spectroscopy and its derived techniques gained increased interest for continuously expanding applications in algal research10–12 of the past decade, due to its unique capability to provide fast track in chemical composition or molecular identification. The unique vibrational molecular signature obtained in non-destructive manner is suitable for various chemical analyses, cells composition mapping or in situ applications, with great appeal for industrial biotechnology. Raman spectroscopy analysis of several microalga species like Dunaliella tertiolecta, Chlorella sorokiniana Neochloris oleoabundans, Botryococcus braunii, Botryococcus braunii Neochloris oleoabundans Chlamydomonas reinhardtii, Trachydiscus minutus, Botryococcus sudeticus, Chlamydomonas sp. were reported.13
Among Raman techniques, surface enhanced Raman scattering (SERS) using noble metal nanoparticles for the enhancement of the Raman scattering is a highly selective technique that can be successfully employed for chemical characterization and imaging of single algae cells.14 In contrast to normal Raman scattering, SERS exploits the laser light interaction with plasmonic nanoparticles working as antennas for signal enhancement. SERS reaches the sensitivity of fluorescence spectroscopy and additionally provides rich information with higher molecular specificity, arising from the close vicinity of the nanoparticles. Furthermore, SERS enables whole information of not only the native analyte, but also the surrounding live biomatrix without additional labelling, chemical processing or separation.
Live Cylindrotheca cells in their culture medium facing AgNPs may provide rich information regarding both the light harvesting complex and extracellular matrix composition and dynamics. Information regarding the nano risk associated with the presence of AgNPs in the microbial native environment is also available when SERS is applied at the interface between nanoparticles and living organisms. The prerequisite of nano risk assessment is the correct interpretation of the complex SERS data.
In this paper we investigated the interface between live marine diatoms Cylindrotheca closterium and silver nanoparticles (AgNPs) by exploiting the SERS technique both in the bulk cell culture and at single cell level, having in mind multiple purposes, (i) to relate both their carotenoids content and their mucilaginous secretion to the outcome of the normal Raman scattering and SERS, (ii) to assess diatoms spectral behaviour in the presence of nanoparticles in their native environment; (iii) to assess the lab results reproducibility with a compact, portable Raman equipment, suitable for in situ diatoms monitoring; and (iv) to further apply the results in a long term aim for seawater monitoring based on SERS sensing. The latest one requires deeper understanding of the SERS effect at the interface between noble metal nanoparticles and marine microbial community.
Experimental
Diatoms sampling
Cylindrotheca closterium diatoms were sampled during the growing phase of a batch culture at the Institute of Coastal and Marine Research of the University of Dubrovnik, Croatia.
A lab-based small photobioreactor with stirring system was used for optimal grow of the diatoms under controlled physical parameters and light exposure. Classical enriched seawater medium (Walne's medium)15 and optimal growing conditions described elsewhere16 were applied for the cell culture. Briefly, the composition of the enriched UV-treated seawater medium for Cylindrotheca includes salts, citrate, trace metals and vitamins. The batch culture sample from bioreactor was subsampled in several glass vials of 1 ml capacity and used for Raman and SERS measurements. At the sampling moment, the cells concentration was about 1.2 × 104 cells per ml.
Ag nanoparticles synthesis and characterization
Distilled water (18.2 MΩ cm) was used for the solutions ingredients for the Ag nanoparticles synthesis according to the citrate-reduced procedure.17 Briefly, 50 ml of a 10−3 M AgNO3 aqueous solution was heated to boiling and then 1 ml of a 1% trisodium citrate (C6H5O7Na3) solution was added. The mixture was kept boiling for 1 hour and then was allowed to cool down. The resulted colloidal nanoparticles (LM-AgNPs) solution was of dark grey colour with absorbance maximum at 405 nm (FWHM of 96 nm) and size distribution centred at about 20 nm.
The hydroxylamine-reduced nanoparticles (HY-AgNPs)18 solution was prepared by dissolving 0.017 g of AgNO3 in 90 ml water. 0.021 g of NH2OH·HCl was dissolved in 5 ml water and 4.5 ml of 0.1 M sodium hydroxide was added to it. The mixture was rapidly added to AgNO3 solution and in a few seconds a grey-brown solution was obtained with absorbance maximum at 418 nm and FWHM of 140 nm. All the glass vials were cleaned using chromic solution and then rinsed many times with distilled water.
SERS sampling
Liquid SERS samples were prepared by adding 10 μl of batch culture solution to 500 μl of AgNPs and measured immediately. Sampling was repeated for 5 times for each type of AgNPs. The diatom cells concentration in 510 μl total SERS sample was estimated at 120 ± 11 cells.
Drop coating deposition samples on a hydrophobic stainless steel Teflon-coated SpectRIM™ plate19 were used for single cell characterization. Two different methodologies were applied. Firstly, the liquid SERS sample providing specific signal was further used for drop coating deposition and investigated after drying at room temperature on the SpectRIM™ plate. In 40 μl SERS sample about 9 to 12 cells were present as counted under the microscope. Secondly, a droplet of pure batch culture was deposited on the hydrophobic plate and after drying the resulted stain was coated with AgNPs droplets and further dried at room temperature for 10 min.
Equipment
Raman and SERS spectra were recorded with a Confocal Renishaw InVia Reflex Raman microscope, using the 532 nm laser line for excitation. Spectral resolution was 0.5 cm−1. For data acquisition, 10 accumulations of 1 s at progressively increased laser power from 0.1 to 1 mW were applied to avoid excessive cells heating and/or photo-bleaching.
Diatoms were alternatively Raman and SERS characterized in the 200–3500 cm−1 range with a fast, compact, dispersive DeltaNU Advantage 532 Raman spectrometer with 8 cm−1 resolution using the 532 nm laser line for excitation. Five accumulations of 5 seconds each were averaged for the final recorded signal.
For achieving non-resonant Raman conditions, batch culture solution was characterised using the FT-Raman (Bruker) system with NIR excitation at 1064 nm and 2 cm−1 resolution. 500 scans were co-added and the output laser power was set to 350 mW.
Electronic absorption spectra of AgNPs stocks before and after adding diatoms batch culture or seawater respectively, were recorded with a Jasco UV-VIS-NIR diode array double beam spectrometer ABL&E Jasco V 360 in the 200–1100 nm range using standard quartz cells at room temperature.
Results and discussions
Raman spectroscopy of the diatoms batch culture
For assessing the Raman signal of the batch culture, we employed both the visible (532 nm) and near infrared (1064 nm) excitation. A lab-based Raman system and a compact, portable one employed the 532 nm line which pre-resonantly excited the carotenoids from the cells composition. Near infrared excitation would provide in a non-resonant manner an overall Raman signature of the chemical components with different extent, depending on the specific Raman scattering cross section. Previous Raman studies on several green algal species10–13 showed that the both algal bulk solutions and single cells were dominated by the carotenoids signal superimposed with the strong fluorescence signal when the 532 nm line was used for excitation. Raman spectra of the freshly sampled diatoms batch culture collected with three different instruments are showed in the Fig. 1A. The FT-Raman instrument with NIR excitation (1064 nm) provided poor signal of Cylindrotheca batch culture, almost similar to that of natural seawater collected from the coastal water from the South Eastern Adriatic coast, in Dubrovnik. This is probably due to the longer acquisition time needed, which could lead to the slow bottom settling of the cells in the glass vial and consequently, weak Raman scattering collected from the bulk solution in the back scattered geometry of the FT-Raman instrument. The sulphate band at 978 cm−1 typical for the raw seawater FT-Raman spectrum was the only major band of the culture solution (Fig. 1A, c), the carotenoid signal hardly exceeding the noise level for NIR excitation. The Raman relative intensity ratio of sulphate to water stretching mode at 3214 cm−1 (R = I(978)/I(3214)) was calculated both for Cylindrotheca batch solution and raw seawater. The mean R value resulted from five distinct measurements on Cylindrotheca solution was 0.75, higher than for the raw seawater (0.61), thus, NIR-FT-Raman spectrum promptly showed the higher sulphate content of the diatoms batch culture compared to natural seawater. This result suggests faster aggregation of the nanoparticles by the salty culture medium than the seawater. Calculating the spectral difference between the averaged spectra of Cylindrotheca and seawater signal, several weak bands attributable to the chlorophylls and extracellular polymeric substances secreted by diatoms were weakly evidenced and highlighted in the Fig. 1B. The main fucoxanthin band at 1528 and 1160 cm−1, the Chl a/b bands in the 700–1360 range, as well as at 1555 cm−1 while fingerprint bands attributable to fatty acids were observed in the high wavenumber range. The Raman bands of the bulk diatom cells observed for visible excitation (532 nm) at 1528, 1156 and 1007 cm−1 together with their linear combinations and harmonics in the 2110–2700 cm−1 range (Fig. 1A, a) are characteristic to carotenoids.10–13,20–24 The spectral information provided by the portable equipment, although less sensitive for the spectral margins, suggests the possibility to monitor the live cell culture status concerning the carotenoids content. The conjugated C
C double bond is sensitive to the molecular structure of the carotenoid, being largely used to identify carotenoids in complex environmental pools. The Raman band of Cylindrotheca closterium at 1528 cm−1 suggests the fucoxanthin as the most dominant species.12,21,24 Resonance Raman bands of fucoxanthin have been observed at 1529, 1162 and 1013 cm−1 as the most intense bands.21 Another doublet between 1000 and 1020 cm−1 was observed for the fucoxanthin chlorophyll a/c2 proteins complex and its excitation profile was sensitive to the laser line.21 Compared to the β-carotene as carotenoid reference Raman signal, the Raman spectrum of Cylindrotheca showed an additional weak band at 1649 cm−1 (Fig. 1A, a) whose origin could be assigned both to the proteins and fatty acids but also to C
O bond from fucoxanthin. However, the identification of the carotenoid species from live microorganisms only according to the position of the C
C double bond of the polyene chain should be regarded with caution, due to the intrinsic molecular specificity of the carotene C
C mode.20 It was generally reported that diatoms contain two type of photosynthetic pigments, chlorophylls (Chl a and Chl c) and carotenoids, fucoxanthin being a dominant species responsible for light harvesting.22 Apart from Chl a and c and fucoxanthin, which play a light-harvesting function, a group of carotenoids in diatoms comprises those involved in photo protective reactions, including β-carotene, diatoxanthin, diadinoxanthin, violaxanthin, antheraxanthin and zeaxanthin.23 Moreover, the light harvesting complex (LHC) proteins named fucoxanthin-chlorophyll a/c-binding proteins (FCP) host the carotenoids species. Due to the (pre)resonance Raman effect in carotenoids when the excitation with the 532 nm laser line falls into their absorption band, the carotenoids Raman signal superimposed with the substantial fluorescence background covers the overall signal of chlorophylls and other extracellular molecular components in diatoms. Additionally, the 532 nm line excites the proteins fluorescence in the carotenoid–chlorophyll protein complex, the water soluble light harvesting antennas.
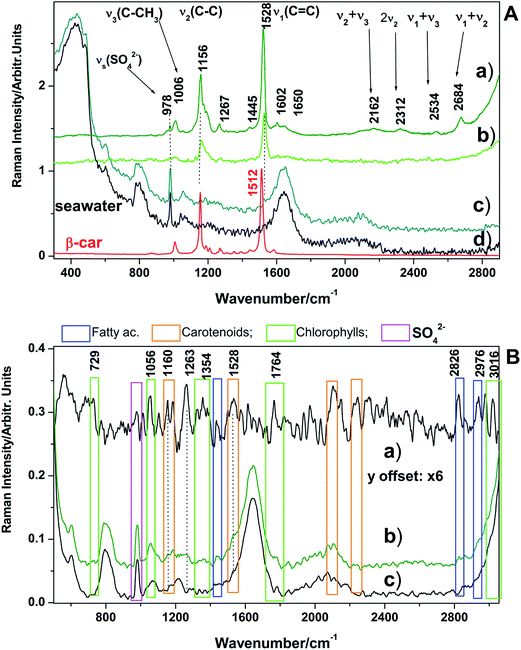 |
| Fig. 1 (A). Normalized Raman spectra of the bulk Cylindrotheca closterium culture solution recorded with a Renishaw instrument (a), a compact, portable and fast DeltaNu spectrometer (b) and with the FT-Raman system (c). FT-Raman spectra of the raw seawater (d) and solid β-carotene (red spectrum, bottom) are shown for comparison. Y offset was applied for clarity. Excitation: 532 nm (a and b) and 1064 nm (c and d). (B) Spectral difference (a) between the averaged FT-Raman spectra of bulk Cilyndrotheca (b) and seawater (c). Although weak, the difference signal shows traces characteristic to chlorophylls, carotenoids, fatty acids and sulphate ions, which are highlighted in rectangles. Y offset (6×) was applied for clarity. | |
Surface enhanced Raman scattering of diatoms batch culture
SERS spectra of the bulk diatoms solution on the HY-AgNPs and LM-AgNPs are shown in the Fig. 2A (300–1800 cm−1 range). The Fig. 2B and C highlight the low (120–330 cm−1) and high (2550–3200 cm−1) spectral range, respectively. Additionally, a three months-aged colloidal HY-AgNPs, was used for comparison. Adding small amount of 10 μl diatoms batch culture to the nanoparticles results in instant colour change from dark yellow to dark blue-grey, due to the fast aggregation in the presence of diatoms and anions from the batch culture including seawater. The nanoparticles aggregation was confirmed by the strong red shift of the AgNPs plasmon resonance and the absorption band broadening over the whole visible range. Fig. 3 illustrates such an example for the LM-AgNPs before and after adding diatoms culture or seawater. The time evolution of the electronic spectra of AgNPs in the presence of seawater over one hour is also showed (Fig. 3). Comparatively, after adding the diatoms culture, the NPs plasmon resonance broadening is stronger and much faster than for the NPs-seawater solution. The others NPs stocks showed similar feature for diatoms, indicating fast aggregation, but different broadening extent and speed for the added seawater. The carotenoid absorption range corresponds well to plasmon energies of Ag nanoparticles. Particularly, β-carotene absorbs light in the 400–500 nm range20 exhibiting three maxima at 427, 451 and 478 nm, fucoxanthin at 416, 442, and 466 nm21–23 while the nanoparticles at 405 nm (LM-AgNPs) and 418 nm (HY-AgNPs). Upon aging (three months kept in dark condition), the latter colloidal solution showed extinction maxima at 440 nm, fitting an absorption band of fucoxanthin. This observation suggests possible prospects on the resonance energy transfer between nanoparticles and diatom pigments in further studies. To date, this is the first SERS experiment on live diatoms and additional information on their molecular behaviour in vivo is scant. The nanoparticles are quickly aggregated by the seawater and culture medium salts. These nano- or micro-aggregates face the theca barrier of diatoms where the EPM gel could be potentially present. Thus, the first SERS signal upon freshly mixing NPs with bulk cells would be expected from this species. The SERS spectra recorded with the three types of NPs showed rather similar Raman modes but their slightly different SERS enhancement was observed. Prominent SERS bands were observed at 1618, 1583, 1497, 1445, 1163, 843 and 730 cm−1 for both LM- and HY-AgNPs with different enhancement extent for each colloidal nanoparticles (Fig. 2). All the three SERS cases exhibited an unexpected feature: the absence of the main Raman mode of diatoms at 1528 cm−1. The presence of the SERS band at 1497 cm−1 might be characteristic to the NPs-adsorbed carotenoid feature, however, a large downshift of 31 cm−1 is quite unusual in SERS. Such an interaction would require an instant NPs uptake through the diatoms theca and cell membrane, as the signal is observed in the first seconds after SERS sample preparation. More feasible, fucoxanthin-chlorophyll proteins would be responsible for this SERS band which is dominant also for the aged AgNPs. This hypothesis is supported by the observation of other characteristic SERS bands of chlorophylls at 730, 805, 910, 1165, 1228, 1346, 1369, 1618 and 1680 cm−1. In fact, all these bands are observed as weak to medium bands in the SERS spectra of diatoms (Fig. S1, ESI†). A typical band characteristic to fatty acids23–26 at 1445 cm−1 (CH2 scissoring mode) was observed (dominant in the HY-AgNPs). Due to the high background of the SERS spectra, other bands characteristic to fatty acids were not observed. The differences observed in the SERS spectra with three colloidal NPs indicates different adsorption mechanisms and significant chemisorption processes suggested by the typical strong SERS bands recorded in the low wavenumber range (Fig. 2B) at 236 or 222 cm−1 usually assigned to the Ag–N or Ag–O from the N- or O-containing molecular species respectively, attached to the AgNPs.27,28 Large differences between the normal Raman and SERS signals (Fig. 1A and 2) clearly indicate other species than carotenoids responsible for the diatom-AgNPs SERS interaction. This result suggests that the (pre)resonance Raman effect observed in bulk diatoms is no longer dominant in the case of SERS in bulk solution. As the cell wall is a complex arrangement of siliceous and organic substances comprising frustulins, pleuralins, silaffins and long chain polyamines,25,26 the AgNPs reaching their proximity could potentially bind to their N-terminal, thus, the observed Ag–N SERS band is fully explainable. The symmetric ν(SiO) modes in the Raman spectra usually observed in siliceous materials at 787 and 764 cm−1 for silicic acid H4SiO4 would be comprised in the SERS signal along with the typical Raman bands (430, 800, 1060, and 1200 cm−1) assigned to the fundamental vibrations of the hydrated silica glass framework.26 Very weak SERS signal at these positions was observed in the spectra of diatoms, indicating that the frustule siliceous material26 does not substantially contribute to the overall SERS.
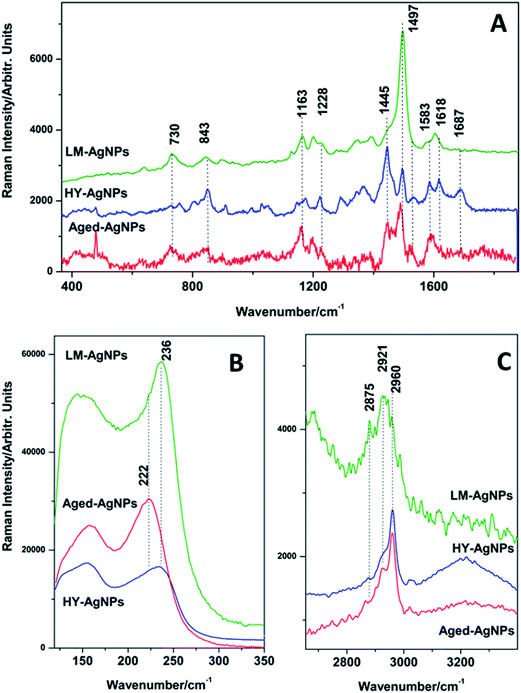 |
| Fig. 2 (A) SERS spectra of the bulk Cylindrotheca diatoms on three different AgNPs, Lee–Meisel (LM), hydroxylamine-reduced (HY) and aged HY-AgNPs respectively, as indicated on each spectrum. Details of the low (B) and high wavenumber range (C) are highlighted. Excitation: 532 nm. | |
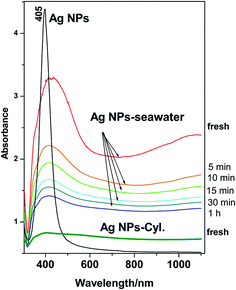 |
| Fig. 3 Extinction spectra of the LM-AgNPs before and after adding seawater or diatoms culture (Cyl). The fresh mixture of 1 ml AgNPs with 20 μl seawater showed slower decrease of peak absorbance at 405 nm than the case of diatoms adding. Spectra were measured after 5, 10, 15, 30 and 60 minutes after mixture preparation, respectively. Large extinction is due to the high density of nanoparticles. | |
Raman and SERS spectroscopy of the single diatom cells
For the immobilisation of single cells while preserving their native environment composition without any additional chemicals, we employed the drop coating deposition of cell culture on a stainless steel hydrophobic SpectRIM™ microscopic plate.19 Same support was simultaneously used for SERS analysis. The drop coating samples and their microscopic images are summarized in the graphical sketch from the Fig. 4. The dried droplets showed circle-shaped spots of crystallized salts mainly distributed on the circles margins and clearly identified by Raman spectroscopy as magnesium sulphate (Fig. S2, ESI†). The live cells were adherently immobilised in the centre and easily observed through the Raman microscope and measured individually. We believe that this diatoms migration and concentration to the middle of the drying droplet on the hydrophobic slide is a natural behaviour for survival. The microscopic image denoted M-AgNPs (Fig. 4i) shows the aggregates resulted from nanoparticles and chemicals available in the culture medium, including the diatoms secreted mucus and possible dead fragments. The microscopic view denoted AgNPs (Fig. 4j) shows the image of self-assembled pure Ag nanoaggregates (Lee–Meisel) after colloidal water evaporation. Optical views clearly showed the influence of the chemicals from the diatoms culture on the nanoparticles aggregation process, however, no microscopic evidence was found between the aggregates in the presence of diatoms culture or raw seawater.
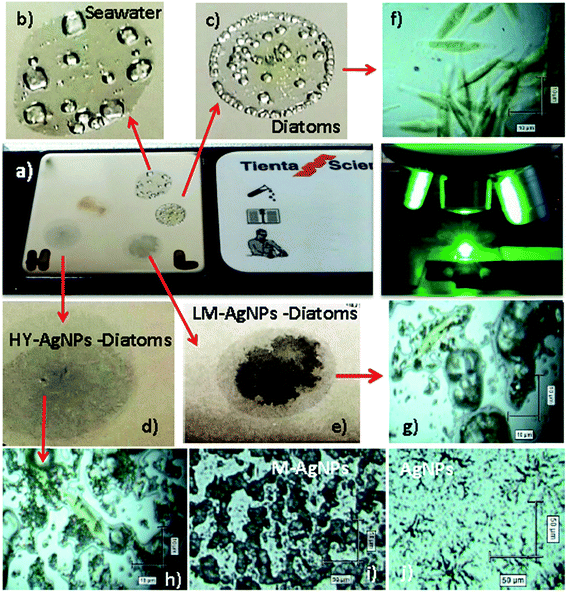 |
| Fig. 4 Graphical sketch showing the drop coating deposition of bulk droplets of diatoms culture solution, seawater and liquid SERS samples (AgNPs-diatoms), as indicated on each image (b–e), on a hydrophobic SpectRIM™ plate (a) and the corresponding images (f–i) taken with the Raman microscope during measurements. The dried droplets showed circle-shapes comprising prominent crystallized salts on the margins (b and c) while the live cells (c and f) remained immobilised in the circle centre. The unmarked droplet on the slide resulted from larger aggregates with cells collected from the bottom of the SERS vial after 2 hours from preparation and its darkness hampered optimal observation. M-AgNPs (i) indicates the mucilage aggregates with nanoparticles observed during optical exploration, while AgNPs image (j) denotes the optical microscopy view of the self-assembled pure Ag nanoaggregates (Lee–Meisel) after colloidal water evaporation. The images denoted (g) and (h) show typical optical views of the cylindrotheca in the presence of LM- (g) or HY-AgNPs (h). Scale bar 10 μm (f–h) and 50 μm (i and j). | |
Micro-Raman signal of the single diatom cells immobilised on the hydrophobic stainless steel plate after water evaporation is showed in the Fig. 5. The optical image provided by the Raman microscope through the 100× objective (Fig. 5, insertion) shows the cells employed for Raman spectra acquisition. The raw Raman signal (Fig. 5A, a and b) recorded from the cell surface comprises a strong fluorescence background and the Raman bands of carotenoids. Upon background subtraction (Fig. 5A, c and B, a–e), different cells showed carotenoids (fucoxanthin dominant) signal with different extent.
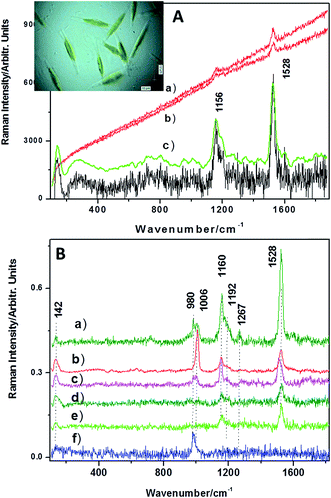 |
| Fig. 5 (A) Typical, raw micro-Raman signal (a and b) of the Cylindrotheca closterium single cells on a hydrophobic plate and the background subtracted signal (c). The smoothed signal is highlighted in green. Light microscopy image of Cylindrotheca closterium cells drop coated on the hydrophobic plate (microscope objective magnification 100×) is shown in insertion. (B) Raman spectra (background subtracted) collected from the green body of several live cells (a–e) and from the transparent terminal body (f). Excitation: 532 nm. | |
The dominant feature of the normal micro-Raman spectra collected from single diatom cells was the C
C stretching mode of fucoxanthin at 1528 cm−1 whose intensity variation was observed from one cell to another and from various points over the green body of the same cell. The intensity variation is consistent with the carotenoid distribution along the body as well as with the cell age. The fluctuant Raman scattering intensity from one diatom cell to another suggests the fucoxanthin quantification possibility during cell growing and development. The transparent siliceous terminals of the diatoms showed no signal except the weak band at 1008 cm−1 (Fig. 5B, e), which was assigned to the salt residue (magnesium sulphate) resulted after (sea)water evaporation from the medium (Fig. S2, ESI†). No other Raman signal except the sea salts signature was detectable around the diatoms body using the 100× objective. Microscope objectives with lower numerical aperture comprising extended area for Raman scattering collection could not spatially differentiate between the signal coming from the diatoms green body or surrounding medium.
The carotenoid-like Raman signal was dominant over the whole green body in various green algae species without silica shell (theca). Moreover, Grosser et al.24 showed that the carotenoid Raman signal could be recorded with a confocal micro-Raman system in a distance of 0 to 150 μm in z direction from the surface (xy stage) of the fucoxanthin-dominant brown alga Fucus vesiculosus and the β-carotene dominant green alga Ulva sp., and explained this result as a release of the non-polar metabolites involved in antifouling processes. The carotenoid intensity exponentially decreased with the z distance, losing half of the intensity in the first 20 μm from the surface. If the metabolites would be present around or on the top of the cells, we expect to observe their specific SERS signal when the NPs or nano-aggregates approached the diatom cells. It should be mentioned that around the cell the residual substances from the medium and from the colloidal solution would be also present. Therefore, the SERS measurements were strictly focussed on the green cells body. To achieve single diatom SERS, 40 μl from each liquid SERS sample (bulk SERS) described above was deposited on the hydrophobic plate and examined after water evaporation. The different AgNPs clearly revealed different aggregation in the diatom culture solution and consequently different SERS signal.
SERS spectra of single diatoms are shown in the Fig. 6, both for the LM-AgNPs (Fig. 6A) and for the HY-AgNPs (Fig. 6B), respectively. The strong band at 236 cm−1 in Fig. 6A indicates significant SERS chemisorption process of N-containing molecular species on the NPs surface as well as different lattice structure of nanoaggregates (band at 149 cm−1) while the medium band at 222 cm−1 in the B panel suggests O-containing chemisorbed species with respect to the HY-AgNPs.27,28 In both cases, carotenoids were however, dominant, but with different output. The fucoxanthin band at 1528 cm−1 was absent in both cases. In the case of HY-AgNPs the SERS signal collected from diatoms body (Fig. 6B, spectra a–c) clearly revealed strong contribution from the Ag-adsorbed β-carotene20 based on the similarity in bands positions and relative intensities. We recently showed that beta-carotene exhibit a complex SERS signal in the presence of AgNPs indicating a strong chemical interaction between the NPs and the cyclohexene ring terminals20 and this SERS feature includes, besides the conventional Raman modes at 1512 and 1156 cm−1 other prominent band at 1649 cm−1 (Fig. 6B, spectrum d). As the β-carotene is located in the light harvesting complex from cell membrane and deeper in the cell, such SERS feature would be possible only in the case of nanoparticles attaching the membrane to allow SERS chemisorption process. This hypothesis is supported by the SERS signal similarity with the β-carotene SERS feature (all the bands of adsorbed β-carotene are present in the case of Fig. 6B, a and b) and is also sustained by the reported AFM study of diatoms in the presence of AgNPs8 described in the Introduction section. An additional SERS band at 1618 cm−1 which is not related to the Ag-adsorbed carotene was observed in the SERS spectra of diatoms (Fig. 6B, spectra b and c) and its origin could be tentatively assigned to chlorophylls (similar to the bulk SERS) but also to typical marine excreted substance containing C
C conjugates and aryl groups.27,28 On the other hand, the β-carotene (and not fucoxanthin) presence in the proximity of the inner membrane for chemisorption could also suggest a natural defense mechanism of the live cells and not only an antifouling mechanism.24 Phytoplankton cells agglomeration is usually inhabited by the Cylindrotheca diatoms (Najdek et al., 2002),29 where the skeletal bodies of dead fragmented organisms are present. As long as the live cells could presumably react to the AgNPs aggression, the dead fragments or components could either attach to them to provide specific SERS feature not necessarily related to carotenoids. Another important factor potentially influencing the signal could be the spurious presence of the cyanobacteria originated from the seawater used in culture medium, although the UV treatment and filtering was conducted in the usual batch culture protocol. These contributions to the overall SERS feature upon trapping in micro-aggregates or approaching them cannot be completely excluded. The SERS variation from one diatom cell to another was expected, due to the randomly distributed nanoaggregates over the diatoms body and due to the cells age and dimension.
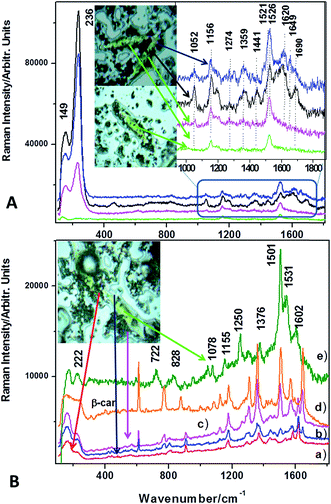 |
| Fig. 6 (A). SERS spectra of the LM-AgNPs-Cylindrotheca collected from the top points of the green cell bodies showed in inserted pictures after bulk water evaporation on the hydrophobic plate; (B) SERS spectra of the HY-AgNPs-Cylindrotheca system (a–c) collected from the dark aggregates and from the green body (e). The aggregates embedded cells are shown in the inserted micrographs. The SERS signal of pure β-carotene ethanol solution on LM-AgNPs and denoted “β-car” is shown in the (d) spectrum for comparison. The arrows indicate the SERS signal collecting points. | |
Furthermore, we noted an intriguing SERS signal (Fig. 6B, spectrum a) coming from the interface between green cell bodies and aggregated material in several diatoms. Its similarity with that of typical ASP or DSP marine toxins28,30 was remarkable, in terms of SERS bands positions and relative intensity. An example of toxin SERS signal is showed in the ESI, Fig. S3,† where the okadaic acid DSP toxin adsorbed on HY-AgNPs, revealed the same SERS spectral feature as several diatom cells. However, this behaviour was observed only in several randomly distributed cells. Two SERS bands characteristic to DSP toxins at 1618 and 1583 cm−1 (Fig. S3, ESI†), strong in Fig. 6B, a and b or superimposed with the carotenoids contribution (spectrum c), were assigned to the –C
C– conjugates specific to the toxins molecular structure.28,30 These bands are also present in SERS spectra of chlorophylls (Fig. S1, ESI†) but the overall SERS feature in the case of chlorophylls interaction with similar AgNPs is however different. To date, Cylindrotheca closterium diatoms were not associated with any harmful toxins release. The spectral match of SERS from few randomly distributed diatoms with typical toxins SERS feature is however, intriguing and additional studies would be required to understand this chemical behaviour in the presence of AgNPs. This aspect as well as the raw seawater SERS feature will be addressed elsewhere.
For the LM-AgNPs the weaker signal (Fig. 6A) indicate that the aggregates are less probably crossing silica frustule to approach to the cell membrane uptake, therefore, the SERS signal is dominated by the conventional carotenoid – like Raman feature (bands at 1156, 1521, 1526 cm−1) and not the chemisorbed ones.20 Additional bands observed at 1254, 1359, 1690 cm−1 were related to the polyunsaturated fatty acids and chlorophylls.25,26,29–33 Polysaccharides specific signal was absent and this negative result is in agreement with the previous study9 showing that in the exponential growth phase, Cylindrotheca diatoms secrets negligible extracellular polymers. In the stationary phase of growth extracellular polymers were observed in more than 25% of the diatom cells.9
Even larger AgNPs aggregates (extinction maximum at 444 nm) available from three months aged HY-AgNPs colloidal solution were probed for the SERS response (Fig. 7). The SERS spectral feature clearly revealed the enhancement of the fatty acid bands together with the conventional signal from carotenoids upon 532 nm laser excitation. The fatty acids characteristic bands32–38 at 1269, 1429, 1445 cm−1 highlighted in blue (Fig. 7) and the carotenoids bands highlighted in light orange at 1156, 1504 and 1521 cm−1 were dominant in the diatoms SERS signal. Additionally, the very strong SERS band at 225 cm−1 supports the supposition of O-adsorption through the carboxylic groups of fatty acids on the Ag colloidal surface. The SERS result with aged AgNPs of greater size highlighting the fatty acids strong contribution is also fully explainable considering outer diatoms gel matrix attachment to greater size aggregates and consequently difficult to penetrate through the frustule to inner cell wall. The EPS represents a subtle network of polysaccharide fibrils cross-linked in fibrils junctions and fibril-globule interconnections with globules connecting two or more fibrils.9,25 Glucose units characteristic to polysaccharides exhibit Raman bands at 405(s), 440(m), 542(s), 650(mw), 772(mw), 841(ms), 914(ms), 1002(mw), 1022(m), 1054(m), 1075(ms), 1120(ms), 1149(m), 1272(m), 1296(ms), 1459(m).34 Due to the chemisorption processes, their identical band position and relative intensity was not expected on passing from Raman to SERS. Even more, the complexity of the SERS signal arising from the surface of live cells in contact with the AgNPs aggregates would be judged taking into account the resonance Raman effect in conjunction with SERS, or surface enhanced resonance Raman (SERRS) of carotenoids, superimposed with the SERS of other secreted substances and chlorophylls.
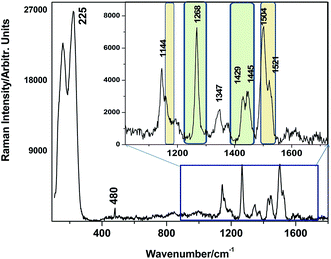 |
| Fig. 7 SERS signal of the C. closterium diatoms with aged hydroxylamine-reduced AgNPs. The 900–1700 cm−1 range is shown in insertion for clarity. The fatty acid bands are highlighted in blue while the carotenoids signal in light orange. Excitation: 532 nm. | |
The mucilage matter excreted by the diatoms through the raphes may provide the means for cell attachment to the AgNPs. In the case of such an event, we expected to record SERS signal comprising the polysaccharides bands. Due to the complexity of the signal, their presence may be covered by the dominant SERS feature of other components.
A novel phenomenon of self-orientation of gold nanorods at oleic acid–water immiscible interface was described36 to create a substrate-free interfacial liquid-state SERS. The vertical alignment of the nanorods at the interface allowed higher Raman enhancement than in the case of randomly oriented nanorods with three to four order of magnitude. The SERS outcome showed typical bands of fatty acid.36 Such an effect is perfectly plausible for nanoaggregates that are instantly formed when the AgNPs get in contact with the salty environment and further the nanoaggregates attached to the mucilage. This hypothesis could perfectly explain the SERS feature of the bulk C. closterium showed in the Fig. 7 which was observed with aged HY-AgNPs exhibiting absorption maxima at 440 nm.
Although the plasmon resonance of the aggregates shifted to NIR suggested the possibility to probe NIR-SERS, the NIR excitation of SERS samples was unable to provide any signal, probably due to the insufficient energy for Raman excitation which was completely covered by the fluorescence emission only.
SERS of the immobilised diatom cells by AgNPs coating
The drop coated deposition of pure batch culture on the hydrophobic microscopic plate was further kept for micro-SERS investigation after coating with LM-AgNPs. We examined 12 cells concerning their SERS response after the colloidal water evaporation. There were two cases, as shown in the Fig. 8A and B. First case (dominant) showed SERS signal consistent with the enhanced Raman scattering of carotenoids modes, with similar bands position with the normal Raman modes of single cells. Typical SERS spectra collected from the green body of diatoms using 20× objective (spectra numbered 1–4) or 100× objective (spectra 5–8) revealed the characteristic carotenoid bands superimposed with the fluorescence background. The main carotenoid SERS band position ranged from 1521 to 1528 cm−1, suggesting that after drying process the diatoms interaction with the LM-AgNPs is changed. Normalised, background subtracted spectra collected from individual cells from Fig. 8 is shown in the Fig. S4, ESI material.† The pictures captured with the Raman microscope in the Fig. 8 highlight the dried algal bodies coated with LM-AgNPs drops. The signal numbered from 1 to 12 was collected from individual diatoms body from the corresponding micrographs. As the cells were stressed due to the aqueous medium evaporation, they could presumably release metabolic substances. In the second case, the SERS signal (9–12) collected from the black dots (aggregates) randomly distributed on few diatoms body and highlighted with circles in inserted micrographs, was related with this metabolic release process (Fig. 8). Spectral comparison suggests that the released substances vary from one cell to another. Raman signal (1) of single diatom before coating with LM-AgNPs drops is shown for comparison.
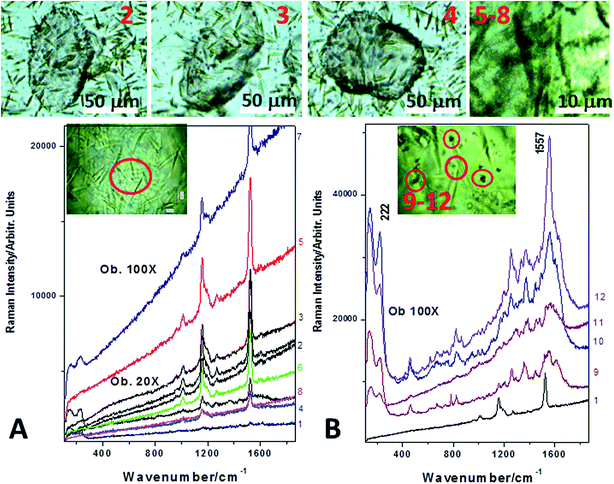 |
| Fig. 8 (A) Typical SERS spectra collected from the green body of diatoms using 20× objective (spectra 1–4) or 100× objective (spectra 5–8) revealing the carotenoid bands superimposed with the fluorescence background. The above pictures captured with the Raman microscope highlight the dried algal bodies drop coated with LM-AgNPs. Scale bar: 50 μm (for 20× objective) and 10 μm (100×). (B) SERS signal (9–12) collected from the black dots (aggregates) on the diatoms body highlighted with circles in inserted picture. Raman signal (1) is shown for comparison. | |
Concerning the metabolite release, EPA (eicosapentaenoic acid – omega-3 fatty acid) is of high interest for economical purpose. Raman spectrum of EPA used for constructing a calibration curve for iodine value38 estimation, showed bands at 1267, 1445 δ (
CH2) and 1656 cm−1 ν(C
C). While the latter band is observed as shoulder in most studied cases, some bands in the SERS spectra of diatoms showed common feature, while other bands (i.e. band at 1557 cm−1, Fig. 8B) were enhanced with different extent from one cell to another, depending on the local adsorbed plasmonic nanoparticles on their body. A characteristic SERS band was uniformly observed in all the SERS spectra (Fig. 8) at 222 cm−1 being attributable to the Ag–O bond resulted from chemisorbed fatty acids species on the AgNPs.
In Raman scattering, the intensity being proportional with the analyte concentration, it is possible to determine the balance between the unsaturated to saturated fatty acids in a mixture using the iodine value38 based on the fact that the band at 1656 cm−1 corresponds to the cis –C
C stretching mode proportional to the amount of unsaturated C
C bonds; and the band at 1445 cm−1 is associated with the CH2 scissoring mode, whose intensity is proportional to the amount of saturated C–C bonds, as saturated fat indicator. However, assuming that a biomatrix is uniformly incubated with nanoparticles (which is less feasible in real experiments), this approach must be evaluated with much caution in SERS, due to the localised surface plasmon resonance effects and the surface selection rule modification upon chemisorption.
Conclusions and outlook
Raman spectra of live diatoms excited with the 532 nm laser line provided typical signature of fucoxanthin on fluorescence background both for the bulk culture and single cells, while the SERS signal of the bulk diatoms showed different feature for the citrate- and hydroxylamine-reduced AgNPs, indicating different interaction mechanisms. Additionally, the SERS signal of single cells with adsorbed AgNPs showed different signature to that of the bulk, allowing evidence of the extracellular polymeric substances. Typical bands assigned to the polyunsaturated fatty acids were observed together with specific SERS contribution of carotenoids and chlorophylls.
The three types of AgNPs used in the present study showed distinct interaction with the diatoms cells. The common SERS feature with the three types of NPs is the presence of the carotenoid specific signal while the distinct feature is the presence of additional bands whose origin could be associated with the extracellular substances. The size and optical properties of the AgNPs clearly influence the SERS output of both the bulk diatoms and single cells.
The compact, portable Raman equipment provided good reproducibility for assessing the carotenoid content in diatoms batch culture by normal Raman scattering, while SERS of the bulk diatoms provide valuable, robust and fast tool for evaluation of carotenoids or the extracellular metabolites in live diatoms cultures.
The characteristic SERS feature of the bulk diatoms further suggest that the diatoms contribution would be relevant in the overall SERS signalling of seawater in the blooming alga periods when the diatoms content is increased. However, additional SERS studies will be necessary on various diatoms species for monitoring applications purpose. Although extensively exploited to detect polycyclic aromatic hydrocarbons from environmental waters, SERS technique still has limited in situ applications due to the difficulty to correctly interpret and exploit the complex information comprised in the SERS spectra. The SERS signal generated by the natural seawater still requires extensive experimental data to be able to extract systematic information for monitoring purpose. As the seawater comprises rich microbial community (plankton) as well as dissolved organic and inorganic compounds, beyond the so-called sea snow (detritus), to understand the SERS signal outcome requires understanding the complex nanoparticles aggregation processes induces by anions in conjunction with the specific interaction of microorganisms with the noble metal nanoparticles. The rapid concentration increase or accumulation of certain microscopic algae species generates drastic physic-chemical and biological changes of the real aqueous ecosystems and these changes are expected to be measurable exploiting the plasmonic nanoparticles and SERS technique. Therefore, the individual species contribution in the overall SERS signal is essential. To date, there are no SERS studies devoted to this aspect in natural seawaters. If the diatoms, dinoflagellates or cyanobacteria that populates environmental waters could be used as effective biological indicators of the presence of nanoparticles in aquatic system based on their grow rate and photosynthetic capability, their SERS signal should be a valuable and effective tool for long run monitoring purpose.
Acknowledgements
This work was possible due to the NEWFELPRO Grant No. 5/2014 of the Government of the Republic of Croatia and the Ministry of Science, Education and Sport (MSES) co-financed through the Marie Curie FP7-PEOPLE-2011-COFUND. Cs. M acknowledges the Sectorial Operational Program for Human Resources Development 2007-2013, co-financed by the European Social Fund, project no. POSDRU/159/1.5/S/132400. The Babeş-Bolyai University Research Infrastructure Project financed by the Romanian Government, PN II-Capacities, Integrated Network for Interdisciplinary Research (INIR) is highly acknowledged. Special thanks to Professor Nenad Jasprica from the Institute for Marine and Coastal Research, Univ. Dubrovnik, Croatia, for the valuable discussions and critical review on the presented topic.
References
- M. Najdek, M. Blažina, T. Djakovac and R. Kraus, J. Plankton Res., 2005, 27, 851–862, DOI:10.1093/plankt/fbi057.
- B. E. F. Reimann, J. C. Lewin and B. E. Volcani, J. Cell Biol., 1965, 24, 39–55 CrossRef CAS PubMed.
- Z. Demirel, E. Imamoglu and M. Conk Dalay, J. Chem. Technol. Biotechnol., 2015, 90, 2290–2296, DOI:10.1002/jctb.4687.
- Y. Liang, K. Mai and S. Sun, J. Appl. Phycol., 2005, 17(1), 61–65 CrossRef CAS.
- S. Wang, L. Zhang, G. Yang, B. Zhu and K. Pa, J. Ocean Univ. China, 2015, 14, 357–361 CrossRef CAS.
- K. Suman, T. Kiran, U. Koduru Devi and N. S. Sarma, Bot. Mar., 2012, 55, 289–299, DOI:10.1515/bot-2011-0076.
- M. Hildebrand, G. Holton, D. C. Joy, M. J. Doktycz and D. P. Allison, J. Microsc., 2009, 235, 172–187, DOI:10.1111/j.1365-2818.2009.03198.x.
- G. Pletikapić, V. Žutić, I. Vinković Vrček and V. Svetličić, J. Mol. Recognit., 2012, 25, 309–317, DOI:10.1002/jmr.2177.
- V. Svetličić, V. Žutić, G. Pletikapić and T. Radić, Int. J. Mol. Sci., 2013, 14, 20064–20078 CrossRef PubMed.
- N. D. T. Parab and V. Tomar, J. Nanomed. Nanotechnol., 2012, 3(131), 5–7, DOI:10.4172/2157-7439.1000131.
- Y. Y. Huang, C. M. Beal, W. W. Cai, R. S. Ruoff and E. M. Terentjev, Biotechnol. Bioeng., 2010, 105, 889–898, DOI:10.1002/bit.22617.
- J. Jehlicka, G. M. E. Howell and A. Oren, Appl. Environ. Microbiol., 2014, 80, 3286–3295 CrossRef PubMed.
- P. Pořízka, P. Prochazková, D. Prochazka, L. Sládková, J. Novotný, M. Petrilak, M. Brada, O. Samek, Z. Pilát, P. Zemánek, V. Adam, R. Kizek, K. Novotný and J. Kaiser, Sensors, 2014, 14, 17725–17752, DOI:10.3390/s140917725.
- M. Josefson, A. Walsh and K. Abrahamsson, Methods Mol Biol., ed. D. B. Stengel and E. Connan, 1308, Springer, New-York, 2015, pp. 365–74. DOI:10.1007/978-1-4939-2684-8_23.
- R. A. Anderson, Algal Culturing Techniques, Elsevier Academic Press, Burlington, Massachusetts. 2005 Search PubMed.
- M. B. Kingston, J. N. C. Acad. Sci., 2009, 125, 138–142 Search PubMed.
- P. C. Lee and D. J. Meisel, J. Chem. Phys., 1982, 86, 3391–3396 CrossRef CAS.
- N. Leopold and B. Lendl, J. Phys. Chem. B, 2003, 107, 5723–5727 CrossRef CAS.
- S. Cinta Pinzaru, N. Peica, U. Schmidt and W. Kiefer, J. Optoelectron. Adv. Mater., 2007, 9, 768–771 Search PubMed.
- S. Cintă Pinzaru, C. Müller, S. Tomšić, M. M. Venter, B. I. Cozar and B. Glamuzina, J. Raman Spectrosc., 2015, 46, 597–604, DOI:10.1002/jrs.4713.
- L. Premvardhan, L. Bordes, A. Beer, C. Büchel and B. Robert, J. Phys. Chem. B, 2009, 113(37), 12565–12574, DOI:10.1021/jp903029g.
- J. L. Stauber and S. W. Jeffrey, J. Phycol., 1988, 24, 158–172, DOI:10.1111/j.1529-8817.1988.tb04230.x.
- P. Kuczynska, M. Jemiola-Rzeminska and K. Strzalka, Mar. Drugs, 2015, 13, 5847–5881, DOI:10.3390/md13095847.
- K. Grosser, L. Zedler, M. Schmitt, B. Dietzek, J. Popp and G. Pohnert, Biofouling, 2012, 28, 687–696, DOI:10.1080/08927014.2012.700306.
- G. Pletikapić, T. M. Radić, A. H. Zimmermann, V. Svetličić, M. Pfannkuchen, D. Marić, J. Godrijan and V. Zutić, J. Mol. Recognit., 2011, 24, 436–445, DOI:10.1002/jmr.1114.
- M. Sumper and N. Kröger, J. Mater. Chem., 2004, 14, 2059–2065 RSC.
- S. Cinta Pînzaru and I. Pavel, SERS and Pharmaceuticals, in Surface Enhanced Raman Spectroscopy: Analytical, Biophysical and Life Science Applications, ed. S. Schlücker, Wiley-VCH Verlag GmbH & Co. KGaA, Weinheim, Germany, 2010, pp. 129–154, DOI:10.1002/9783527632756.ch6.
- S. Cinta Pinzaru, C. Müller, S. Tomšić, I. Ujević, A. Bratoš Cetinić, B. I. Cozar, R. Stiufiuc, M. M. Venter and B. Glamuzina, Noble Metal Nanoparticles in Seawater: Nano Risk Towards Aquatic Organisms Assessed by SERS, Proc. ICAVS 8, ed. B. Lendl, C. Koch, M. Kraft, J. Ofner and G. Ramer, Vienna, 2015, pp. 106–107, http://www.icavs.org/icavs8/images/program/program_web.pdf Search PubMed.
- M. Najdek, D. Debobbis, D. Mioković and I. Ivančić, J. Plankton Res., 2002, 24, 429–441, DOI:10.1093/plankt/24.5.429.
- C. Müller, B. Glamuzina, I. Poznjak, D. Cialla, J. Popp and S. Cinta Pinzaru, Talanta, 2014, 130, 108–115 CrossRef PubMed.
- I. W. Schie, L. Nolte, T. L. Pedersen, Z. Smith, J. Wu, I. Yahiatène, J. W. Newman and T. Huser, Analyst, 2013, 138, 6662–6670 RSC.
- P. Meksiarun, N. Spegazzini, H. Matsui, K. Nakajima, Y. Matsuda and H. Sato, Appl. Spectrosc., 2015, 69, 45–51, DOI:10.1366/14-07598.
- K. Suman, T. Kiran, U. Koduru Devi and N. S. Sarma, Botanica Marina, Walter de Gruyter, Berlin, Boston, 2012, DOI:10.1515/bot-2011-0076.
- O. Samek, A. Jonáš, Z. Pilát, P. Zemánek, L. Nedbal, J. Tříska, P. Kotas and M. Trtílek, Sensors, 2010, 10, 8635–8651, DOI:10.3390/s100908635.
- J. De Gelder, K. De Gussem, P. Vandenabeele and L. Moens, J. Raman Spectrosc., 2007, 38, 1133–1147, DOI:10.1002/jrs.1734.
- K. Kim, H. Soo Han, I. Choi, C. Lee, S. G. Hong, S.-H. Suh, L. P. Lee and T. Kang, Nat. Commun., 2013, 4, 2182, DOI:10.1038/ncomms3182.
- I. W. Schie, L. Nolte, T. L. Pedersen, Z. Smith, J. Wu, I. Yahiatène, J. W. Newman and T. Huser, Analyst, 2013, 138, 6662–6670 RSC.
- O. Samek, P. Zemánek, A. Jonáš and H. H. Telle, Laser Phys. Lett., 2011, 10, 701–709 CrossRef.
Footnote |
† Electronic supplementary information (ESI) available. See DOI: 10.1039/c6ra04255d |
|
This journal is © The Royal Society of Chemistry 2016 |