DOI:
10.1039/C6RA03998G
(Paper)
RSC Adv., 2016,
6, 45219-45230
Synergistic effect of a biodegradable Mg–Zn alloy on osteogenic activity and anti-biofilm ability: an in vitro and in vivo study
Received
16th February 2016
, Accepted 19th April 2016
First published on 20th April 2016
Abstract
It is desirable for orthopaedic implants to possess good osteointegration and anti-biofilm ability simultaneously for the prevention of implant associated infections and promotion of osteointegration. In this study, both of the anti-biofilm ability and osteogenic activity of a Mg–Zn alloy as a novel magnesium alloy were investigated systematically. The in vitro findings revealed that the Mg–Zn alloy promoted osteogenic differentiation and gene expression in the rat bone mesenchymal stem cells (rBMSCs), and showed better antibacterial ability than titanium (Ti). Good antibacterial ability was also observed in vivo in a rat distal femur model; the micro-CT and histological results revealed superior osteointegration of the Mg–Zn alloy in vivo despite the presence of methicillin-resistant Staphylococcus aureus (MRSA). The alkaline pH together with magnesium and zinc ions released from the Mg–Zn alloy may have synergistic effects on the antibacterial ability and osteogenic activity of the Mg–Zn alloy. The results demonstrate that the Mg–Zn alloy possesses osteointegration ability even in the presence of MRSA.
1. Introduction
Although Ti based materials and stainless steels are the most extensively used artificial orthopaedic implants, implant failure often occurs due to insufficient osteointegration, stress shielding and implant-associated infections.1–5 Thus, alternative implant materials simultaneously possessing osteogenic activity, a low elastic modulus and antibacterial ability are highly desirable.
In recent decades, magnesium-based materials have been the focus of biodegradable medical implant research because of their good biocompatibility, desirable elastic modulus, osteogenic activity and antibacterial ability.6–9 Magnesium-based biomaterials can degrade non-toxically in the body and allow new bone formation in the implant site.10 Furthermore, magnesium ions can enhance the osteogenic activity of bone marrow stromal cells.11 Among the various magnesium alloys, a Mg–Zn alloy has been developed as a novel magnesium alloy with favourable biocompatibility and corrosion resistance.12 Zn is chosen as the alloying element due to its good biocompatibility, osteogenic activity and antibacterial ability.13–16 Recently, it has been reported that magnesium could prevent bacterial biofilm formation because of the high alkalinity at its surface.17 It has also been demonstrated that a Mg–Nd–Zn–Zr alloy could effectively enhance the antimicrobial property and corrosion resistance of Mg by alloying with the proper amount of Zn, Zr and Nd.18 Thus, we hypothesized that Mg–Zn alloys would possess the ability to stimulate new bone formation and prevent bio-film formation simultaneously.
Staphylococcus aureus is one of the leading pathogens causing implant-associated infections.19 The emergence of antibiotic-resistant bacteria strains, particularly methicillin-resistant Staphylococcus aureus (MRSA), has become a significant global healthcare issue that has also influenced trauma medicine: between 25 and 32% of infections after fracture fixation are caused by MRSA.20–23 However, there are few therapeutic methods available for implant-associated infections caused by MRSA, which actually have developed resistance to virtually all β-lactam antibiotics.24 Generally, the success of this bacterium as a pathogen is ascribed to its ability to adhere to the surfaces of implants and remain there under the protection of an extracellular matrix consisting of complex glycocalyx known as biofilm.25 To combat the formation of biofilms, orthopaedic implants with antibacterial ability against antibiotic-resistant bacteria, such as MRSA, are urgently needed.
In the current study, the in vitro osteogenic activity and antibacterial ability of a Mg–Zn alloy were evaluated systematically using rat bone marrow stromal cells (rBMSCs) and MRSA (ATCC 43300), respectively. The in vivo osteogenic activity and anti-biofilm ability of a Mg–Zn alloy and Ti implants were evaluated using a rat distal femur implantation model in the presence or absence of MRSA. Eight weeks post-operation, new bone formation around implants was evaluated using micro-CT and histological observation; the anti-biofilm ability of the Mg–Zn alloy was evaluated using rolling over plate and spread plate methods. In conclusion, a Mg–Zn alloy that possesses simultaneous antibacterial ability and osteogenic activity could be a promising candidate for the next generation of orthopaedic implants for the prevention of implant-associated infection and osteointegration promotion.
2. Materials and methods
2.1. Sample preparation and characterization
A Mg–Zn alloy was prepared using high-purity magnesium (≥99.99%) and zinc (≥99.9999%) ingots, which were provided by Shanghai Aoruiji Medical Technology Limited Company. The chemical composition (wt%) of the Mg–Zn alloy was as follows: Fe 0.0038, Si 0.0016, Ni 0.0005, Cu 0.0005, Al 0.0085, Mn 0.0004, Zn 5.6210, and Mg balance. For in vitro experiments, the Mg–Zn alloy and Ti were fabricated into Ø10 × 1 mm round plates. For in vivo experiments, the above materials were fabricated into 6-mm long, 2 mm diameter Kirschner wires. The above materials were polished up to 2000 grit and then ultrasonically cleaned in acetone for 5 min for later experiments. Before the in vitro and in vivo experiments, all samples were sterilized with 29 kGy of 60Co radiation.
2.2. Bacteria preparation and cell culture
Methicillin-resistant Staphylococcus aureus (MRSA) (ATCC 43300) was used to study the antibacterial ability of the Mg–Zn alloy. The strain was cultured on sheep blood agar (SBA) plates for 24 h at 37 °C. A single colony was collected and incubated in 5 ml of sterile Trypticase Soy Broth (TSB; BD Biosciences, Franklin Lakes, NJ) at 37 °C with agitation at 200 rpm for 24 h. For in vitro experiments, the inocula of the strain were prepared to 1 × 106 colony forming units (CFU) per ml in TSB. In vivo, MRSA was diluted to a concentration of 1 × 105 CFU ml−1.
rBMSCs were obtained from the femora and tibia of 8 week-old Sprague-Dawley rats. Briefly, marrow of the femora and tibia midshafts was flushed out and suspended in complete medium (CM) consisting of low-glucose Dulbecco's modified Eagle medium (L-DMEM, Hyclone, USA) supplemented with 10% foetal bovine serum (FBS, Hyclone), 100 U ml−1 penicillin and 100 mg l−1 streptomycin (Hyclone). Non-adherent cells were removed after 48 hours. When they reached approximately 80% confluence, cells were passaged and used for the following experiments from the second to fourth passages. Osteogenic differentiation of rBMSCs was induced by culturing in osteogenic medium (OM) consisting of CM supplemented with 50 μM ascorbic acid, 10 mM β-glycerol phosphate and 100 nM dexamethasone (all from Sigma).
2.3. In vitro cytotoxicity evaluation of the Mg–Zn alloy
The Cell Counting Kit-8 assay (CCK-8; Dojindo Molecular Technologies, Inc., Japan) was used to determine the cytotoxicity of the extract of the Mg–Zn alloy to rBMSCs. The extract of the Mg–Zn alloy was prepared according to the International Organization for Standardization (ISO 10993-5). Briefly, the ratio of the sample to the culture medium was 100 mg ml−1. The samples were incubated in CM at 37 °C for 24 h. The pH of the Mg–Zn alloy extract was measured with a pH meter (Beckman #350, Indianapolis), and the Mg2+ and Zn2+ concentrations were measured using inductively coupled plasma-atomic emission spectroscopy (ICP-AES; Thermo Fisher #iCAP 7200).
rBMSCs (3 × 103 cells per well) were seeded in the 96-well tissue culture plate and cultured with CM in a humidified incubator containing 5% CO2 at 37 °C for 24 h. Then, the CM was replaced by the extract of the Mg–Zn alloy and incubation was continued for 1, 3 and 5 days. The control group involved the use of CM as a negative control. The culture medium was changed every 2 days. After each incubation period, the culture medium was removed and 100 μl of fresh medium with 10% CCK-8 was added to each well. After incubation for 4 h, 100 μl of culture medium was transferred to another 96-well plate for measurement. The absorbance was measured with a spectrophotometric microplate reader (Bio-Rad 680, USA) at a wavelength of 450 nm.
The cell viability of rBMSCs cultured with the extract of the Mg–Zn alloy was also observed after 1, 3 and 5 days by using a live/dead assay kit (Invitrogen) according to the manufacturer's protocol. In brief, cells were washed with phosphate buffered saline (PBS), then incubated with standard working reagents for 30 min and finally observed by fluorescence microscopy (Olympus, Japan). Viable cells combined with calcein-AM were stained green, whereas dead cells combined with ethidium homodimer-1 were stained red.
2.4. In vitro osteogenic activity of the Mg–Zn alloy
2.4.1. Extracellular matrix calcium deposition assay. rBMSCs at a density of 1 × 105 cells per well were seeded into 6-well plates in CM. After 24 h of culture, the medium was replaced with OM containing the extract of the Mg–Zn alloy. The control group involved the use of OM as a negative control. Then, the cells were cultured for 2 and 3 weeks with the medium changed twice per week. After each culture period, the cells were fixed in 10% formalin for 1 h and washed with PBS. The calcium nodules in the ECM were then stained with 0.1% Alizarin red solution (Sigma Aldrich) for 10 minutes. The cells were then rinsed with double stilled H2O and viewed with an inverted light microscope (TE2000U, Nikon, Japan).
2.4.2. Alkaline phosphatase (ALP) staining and activity assay. To determine the osteogenic differentiation of rBMSCs stimulated by the extract of the Mg–Zn alloy, rBMSCs were seeded in 6-well plates at a density of 1 × 105 cells per well and cultured in OM with the extract of the Mg–Zn alloy. ALP staining was performed according to the manufacturer's instructions (Beyotime, Jiangsu, China) at day 10. Briefly, after fixation with 4% paraformaldehyde, the cells were incubated in a mixture of nitroblue tetrazolium and 5-bromo-4-chloro-3-indolylphosphate.26Quantitative ALP activity was measured using an ALP Detection Kit (Njjc-Biotechnology, Nanjing, China) according to the manufacturer's instructions. Briefly, 1 × 105 rBMSCs were seeded in 6-well plates and cultured with CM in a humidified incubator containing 5% CO2 at 37 °C for 24 h. Then the culture medium was replaced by the OM with the extract of the Mg–Zn alloy for 4, 7 or 10 days. ALP activity was assessed according to the manufacturer's instructions and normalized to the total protein content determined by a BCA assay kit (Beyotime, Jiangsu, China).
2.4.3. Quantitative real-time PCR analysis. rBMSCs were cultured in the OM containing the extract of the Mg–Zn alloy for 7 or 14 days. Total RNA was extracted using the TRIZOL reagent (Invitrogen, Carlsbad, USA). The RNA (500 ng) was reverse-transcribed into complementary DNA (cDNA) using a PrimeScript 1st Strand cDNA Synthesis kit (Takara, Japan) according to the manufacturer's recommendations. Quantification of all of the cDNAs of osteogenesis-related genes was performed on an ABI Prism 7500 Thermal Cycler (Applied Biosystem, Australia) using a real-time PCR kit (SYBR Premix EX Taq, Takara). The gene expression level was normalized to that of the housekeeping gene GAPDH, and relative gene expression was analysed with the 2−ΔΔCt method. Each measurement was assessed in triplicate to obtain average data. The primers for the selected genes were as follows: Runx2, 5′-GTCAGCGTCCTATCAGTT-3′, 5′-CGTCAACACCATCATTCTG-3′; osteocalcin (OCN), 5′-AGTCTGACAAAGCCTTCA-3′, 5′-CAAGTCCATTGTTGAGGTAG-3′; osteopontin (OSP), 5′-TCCAAGCAACTCCAATGA-3′, 5′-TGGTGAGATTCGTCAGATT-3′.
2.5. In vitro anti-biofilm assay
Three millilitres of an as-prepared MRSA suspension (1 × 106 CFU ml−1) was added to each well in 12-well plates containing the Mg–Zn alloy and Ti samples and incubated at 37 °C for 1 or 3 days. After each incubation period, the adherent bacteria on the samples' surface were dislodged and determined by the spread plate method, confocal laser scanning microscope (CLSM, Leico) and scanning electron microscopy (FESEM, FEINOVA, NanoSEM). The planktonic bacteria in the culture medium were also determined by the spread plate method.
2.5.1. Anti-biofilm ability assay of the Mg–Zn alloy. After each incubation period, bacterial suspensions cultured with different samples were collected to analyse the viable counts of planktonic bacteria. The adherent bacteria on the samples were detached into 3 ml of TSB by ultrasonic vibration (200 W) for 10 min at 100 Hz. Then, the bacterial suspensions were serially diluted 10-fold, painted in triplicate onto SBA plates and incubated at 37 °C for 24 h. The number of CFUs of MRSA on the SBA plates was counted in accordance with the National Standard of China GB/T 4789.2 protocol. The antibacterial rates for planktonic and adhered bacteria were calculated based on the following formula: (1) Rp = (V − W)/V × 100%. Rp is the antibacterial rate for planktonic bacteria. W represents the mean number of viable bacteria in the culture medium incubated with the Mg–Zn alloy or Ti sample, and V is the mean number of viable bacteria incubated without sample (blank control). (2) Ra = (X − Y)/X × 100%. Here, Ra is the antibacterial rate for adherent bacteria. Y is the mean number of viable bacteria on the Mg–Zn alloy sample, and X is the mean number of viable bacteria on the Ti.
2.5.2. Antibacterial ability assay by CLSM and SEM. At each time point, the samples were transferred into another 12-well plate after being rinsed with PBS three times and then stained with 200 μl of combination dye according to the instructions of the LIVE/DEAD BacLight bacteria viability kit (L13152, Invitrogen) for 15 min. Finally, the samples were observed via CLSM. Viable bacteria with intact cell membranes were stained green, whereas dead bacteria with damaged membranes were stained red. For the SEM observation, the samples were fixed with a 2.5% glutaraldehyde solution for 2 h after being rinsed with PBS for three times and then dehydrated in a graded ethanol series (50, 70, 80, 90, and 95 v/v%) for 10 min sequentially, with the final dehydration conducted in absolute ethanol (twice). Then the samples were freeze-dried, coated with platinum, and observed by SEM.
2.5.3. Mg–Zn alloy corrosion in TSB containing MRSA. Three millilitres of TSB containing a MRSA suspension (1 × 106 CFU ml−1) was added to each well in 12-well plates containing the Mg–Zn alloy or Ti sample and incubated at 37 °C for 1 or 3 days. After each incubation period, the medium was collected and centrifuged at 10
000g for 5 min to remove the bacteria and insoluble degradation products. The Mg2+ and Zn2+ concentrations of the medium were measured using ICP-AES, and the pH of medium was measured using a pH meter.
2.6. In vivo anti-biofilm and osteointegration evaluation
2.6.1. Implant-related osteomyelitis model in rats. All experimental protocols were approved by the Animal Research Committee of Shanghai Jiaotong University School of Medicine. Thirty-two 2- month old male SD rats weighing an average of 250 g were used. The rat distal femur model was used, and the surgical procedures were conducted under sterile conditions. Briefly, after inducing general anaesthesia with 4% chloral hydrate (0.9 ml/100 g body weight) by intraperitoneal injection, each rat was immobilized and its left leg was shaved and depilated. A hole measuring 2 mm in diameter and 6 mm in depth was made with a hand drill at the lateral epicondyle using a minimally invasive approach. Subsequently, the Mg–Zn alloy or a Ti Kirschner wire was implanted into the prepared hole on the left femur of each rat. Ten microlitres of either PBS containing MRSA at a concentration of 1 × 105 CFUs ml−1 or PBS was injected into the drilled hole, followed by the insertion of a Mg–Zn alloy or Ti Kirschner wire. The wound was closed carefully. After operation, the rats were housed in ventilated rooms and given access to water and food. No antibiotic was administered. Four groups were investigated: (1) Ti + PBS, (2) Mg–Zn alloy + PBS, (3) Ti + MRSA, (4) Mg–Zn alloy + MRSA.
2.6.2. Radiographic and micro-CT scanning. At each time point (i.e., 2, 4, 6 weeks), posteroanterior radiographs of the femurs were obtained. After harvesting the femurs at 8 weeks post-operation, micro-computed tomography (Micro-CT) (Skyscan 1176, Kontich, Belgium), with scanning at a resolution of 18 μm for undecalcified specimens, was used to evaluate bone tissue responses around the implants. Three-dimensional (3D) images were reconstructed by CTVol (Skyscan Company). New bone volume and trabecular thickness were determined using the CTAn program (Skyscan Company).
2.6.3. Microbiological evaluation. At 8 weeks post-operation, three random rats in each group were sacrificed and their distal femurs with implants were retrieved aseptically. The Mg–Zn alloy and Ti wires were extracted and rolled over SBA plates for a semi-quantitative analysis of the bacteria adhered on the implant wires. Afterwards, the wires were placed in 3 ml of TSB and sonicated for 10 minutes to dislodge adhered bacteria. Finally the adhered bacteria were counted by the spread plate method.Then, the distal femurs without implants were snap frozen in liquid nitrogen and ground to powder. The powder of each distal femur was vortexed in 3 ml of TSB and centrifuged for 5 min at 10
000g. The supernatant was serially diluted 10-fold and analysed for CFU/femur using the spread plate method.17
2.6.4. Histological observation. The specimens of each group were fixed in 10% formalin for 48 h and dehydrated in ascending concentrations of alcohol from 70% to 100%. Then, the specimens were embedded in polymethylmethacrylate (PMMA). A Leica SP1600 saw microtome (Leica, Hamburg, Germany) was used to cut the embedded specimens into 200 μm thick sections, which were sanded and polished to a final thickness of approximately 50 μm with sandpaper. The sections were then stained with Van Gieson's picrofuchsin. New bone formed around the implants was stained red, and the implants were black. The bone-to-implant contact (BIC) values were calculated.
2.7. Statistical analysis
All of the in vitro experiments were independently conducted in triplicate, and the data are expressed as the means ± standard deviation. One-way analysis of variance (ANOVA) and the Student–Newman–Keuls post hoc test were used to determine the level of significance, and a P value less than 0.05 was considered to be statistically significant.
3. Results
3.1. pH value and ion concentrations of the Mg–Zn alloy extract
The pH value of the extract was 8.07. The concentrations of released magnesium and zinc ions were 17.74 mM and 4.2 μM, respectively. Of note, the concentration of Mg in normal cell culture medium (10% FBS, DMEM) is 0.94 mM according to the product information for DMEM (sigma), and in healthy adult subjects, serum Mg concentrations are maintained at stable levels between 0.75 and 0.96 mM.27
3.2. Effect of the extract of the Mg–Zn alloy on rBMSC proliferation
First, cell viability was evaluated by live/dead staining. As shown in Fig. 1A, rBMSCs cultured with the extract of the Mg–Zn alloy exhibited similar live–dead ratios with the control group at 1, 3 and 5 days. Time-dependent proliferation of rBMSCs was observed in both groups. The proliferation of rBMSCs cultured with the extract of the Mg–Zn alloy was further verified by a CCK-8 assay. As shown in Fig. 1B, the proliferation rate of rBMSCs cultured with the Mg–Zn alloy extract was not negatively impacted compared with the control group.
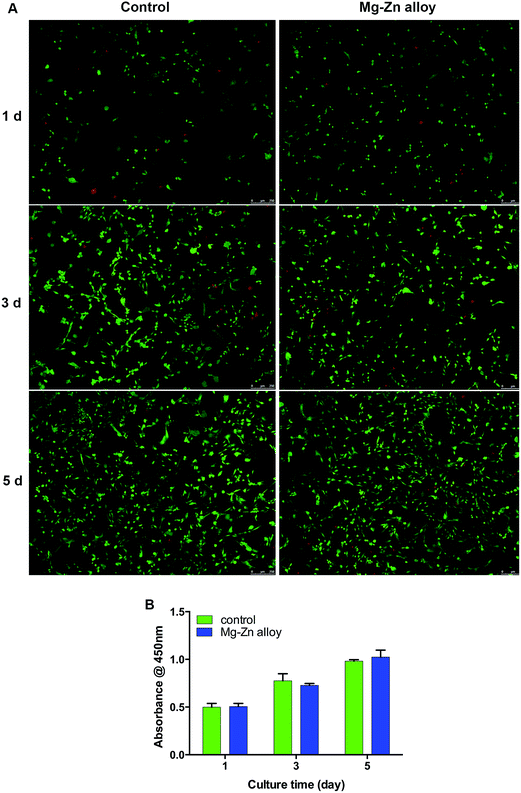 |
| Fig. 1 In vitro cytotoxicity evaluation of the Mg–Zn alloy. (A) Viability of rBMSCs using a live/dead assay at days 1, 3 and 5. Live cells were stained green and dead cells were stained red. (B) CCK-8 assay of rBMSCs cultured for 1, 3 or 5 days with CM and the Mg–Zn alloy extract. | |
3.3. Effect of the extract of the Mg–Zn alloy on osteogenic differentiation of rBMSCs
When exposed to OM containing the extract of the Mg–Zn alloy, rBMSCs underwent more osteogenic differentiation. More mineralized nodules were generated in the Mg–Zn alloy group compared to the control group at weeks 2 and 3, as demonstrated by Alizarin red S staining (Fig. 2A). As shown in Fig. 2B, the ALP staining of rBMSCs cultured in OM containing the extract of the Mg–Zn alloy was more intensive than that of the control group at day 10. Quantitative analysis revealed that the ALP activity increased over time throughout the assay period. Moreover, the Mg–Zn alloy group showed significantly higher ALP activity levels than the control group at days 4, 7 and 10 (Fig. 2C).
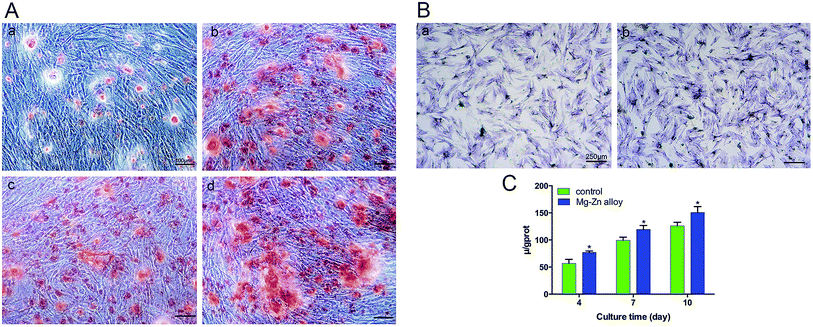 |
| Fig. 2 In vitro osteogenesis evaluation (A) Alizarin red S staining at weeks 2 and 3: (a, c) control group, (b, d) Mg–Zn alloy group. (B) ALP staining at day 10: (a) control group, (b) Mg–Zn alloy group. (C) ALP activity of rBMSCs in two groups at days 4, 7 and 10. *P < 0.05 compared to the control group. | |
3.4. Quantitative real-time PCR
The expression levels of osteogenesis related genes, including Runx2, OSP and OCN, were examined by qPCR after culturing rBMSCs in the presence of the extract of the Mg–Zn alloy for 7 or 14 days (Fig. 3). Comparing with the control group, rBMSCs cultured in OM with the Mg–Zn alloy extract showed remarkably increased expression of Runx2, OSP and OCN at day 7 (P < 0.05). However, there was no significant difference between the two groups when prolonging the culture time to 14 days.
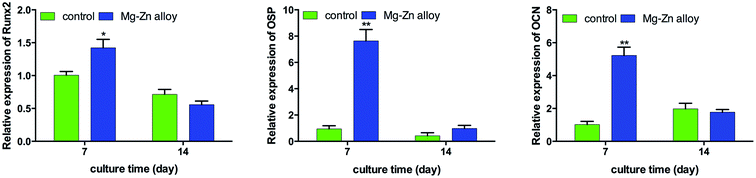 |
| Fig. 3 Osteogenic differentiation analysis for rBMSCs cultured in OM alone (control), or OM with the Mg–Zn alloy extract for 7 or 14 days. *p < 0.05, **p < 0.05. | |
3.5. In vitro anti-biofilm effect
The ability of the Mg–Zn alloy to resist MRSA colonization and biofilm formation was confirmed by CLSM and SEM observations. CLSM views of biofilm formation by MRSA are shown in Fig. 4A. At days 1 and 3, intense green fluorescence on the surface of the Ti could be observed, indicating obvious biofilm formation, whereas less intensive green fluorescence and more red fluorescence was observed on the surface of the Mg–Zn alloy, indicating less MRSA adhesion, more dead bacteria and a low level of biofilm formation.
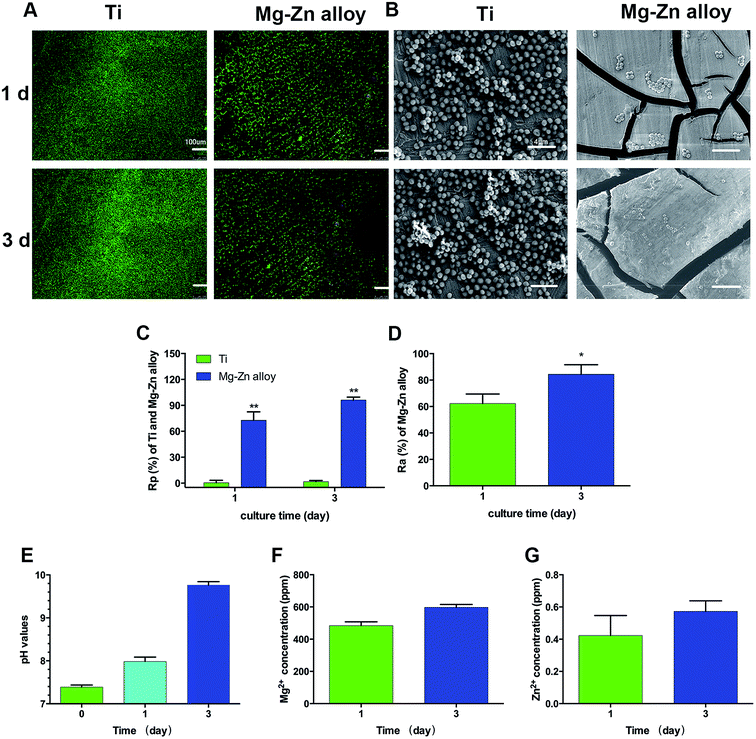 |
| Fig. 4 Anti-biofilm ability of the samples after 1 and 3 days of incubation with MRSA: (A) CLSM images of biofilm formation on Ti and Mg–Zn alloy surfaces. (B) SEM images of biofilm formation on Ti and Mg–Zn alloy surfaces. (C) Antibacterial rates of the samples against planktonic MRSA (Rp). (D) Antibacterial rates of the samples against adherent MRSA (Ra) (E–G) pH, Mg2+ and Zn2+ concentrations of TSB containing Mg–Zn alloy and MRSA at days 1 and 3. *P < 0.05, **P < 0.01. | |
SEM showed a trend similar to that with CLSM observation. As shown in Fig. 4B, larger amounts of MRSA conglomerating into grapelike colonies adhered on the surface of the Ti. In contrast, there were much fewer MRSA that were sparsely adhered on the surface of the Mg–Zn alloy, indicating that biofilm formation was inhibited.
The spread plate method further verified the antibacterial ability of the Mg–Zn alloy against planktonic MRSA (Rp) and adherent MRSA (Ra) at days 1 and 3. As shown in Fig. 4C and D, the Mg–Zn alloy had a higher Rp value of 72.8% and 96.2% at days 1 and 3 compared with Ti. The Ra value of the Mg–Zn alloy samples was 62.3% and 84.5% at days 1 and 3.
The pH value of TSB containing the Mg–Zn alloy with MRSA increased to an average of 7.98 the first day and 9.77 at day 3 (Fig. 4E). The concentration of Mg2+ rose to 484.67 ppm and 597.67 ppm at days 1 and 3, respectively (Fig. 4F). The concentration of Zn2+ was 0.42 ppm and 0.57 ppm at days 1 and 3, respectively (Fig. 4G).
3.6. In vivo anti-biofilm and osteointegration properties
3.6.1. Radiographic assessment. Bone tissue responses around the implants were evaluated by X-ray examination. Obvious osteolysis and periosteal reaction were observed by X-ray in group III at weeks 2, 4 and 6 post-operation. Osteomyelitis developed as manifested by the progress of the periosteal reaction and exacerbation of osteolytic lesions. X rays taken from group II showed some gas accumulation around the Mg–Zn alloy implants, moderate osteolysis and periosteal reaction at week 2. Whereas at weeks 4 and 6, no osteolysis, less gas, new bone formation and a slighter periosteal reaction were observed around the implants. Encouragingly, no obvious osteolysis or periosteal reaction were found around the Mg–Zn alloy implants at weeks 2, 4 or 6 in group IV, which was similar to group II. All of the X-ray images acquired from group I showed no signs of osteolysis or periosteal reaction (Fig. 5).
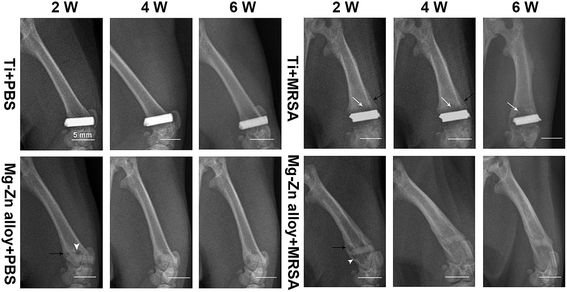 |
| Fig. 5 Radiographic images of the left femur in anteroposterior view: group I: no signs of osteolysis or periosteal reaction. Group II: small amount of gas and slight periosteal reaction emerged at 2 weeks post-operation, which gradually vanished at weeks 4 and 6. Group III: clear signs of osteolysis and periosteal reaction emerged around the implants at weeks 2, 4 and 6. Rats in group IV: similar to group II, no obvious osteolysis or periosteal reaction emerged around the implants. White arrows, black arrows and arrowheads mark osteolysis, periosteal reaction and gas, respectively. | |
3.6.2. Micro-CT evaluation. New bone formation around implant surfaces in the distal femur was evaluated by micro-CT. As shown in Fig. 6A, all of the implants showed direct contact with the newly formed bone in groups I and II; obvious osteolysis and dead bone formation were observed in group III, whereas osteolysis and dead bone formation were not obvious around implants in group IV. The bone tissue responses around implants were quantified next (Fig. 6B and C), and more new bone formation was observed around the Mg–Zn alloy implants than around the Ti implants whether MRSA were present.
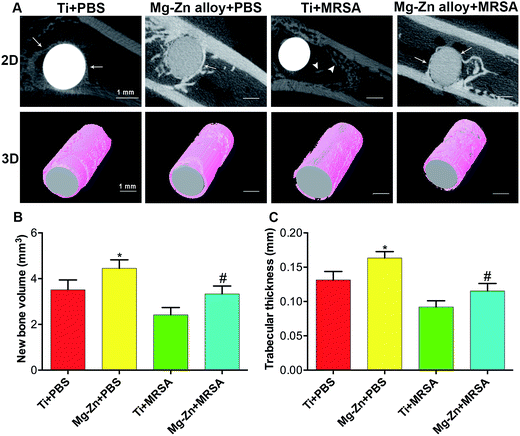 |
| Fig. 6 Characterization of implants and tissue responses by micro-CT: (A) 2D and 3D micro-CT reconstruction images of newly formed bone (white arrows) and dead bone (arrowheads) around Ti and Mg–Zn alloy implants at 8 weeks post-operation. Quantitative analysis of (B) new bone volume and (C) trabecular thickness of new bone around Ti and Mg–Zn alloy implants at 8 weeks post-operation. *p < 0.05 compared to group I, #p < 0.05 compared to group III. | |
3.6.3. Microbiological evaluation. As shown in Fig. 7A, no bacteria colonized on the surface of the Ti or Mg–Zn alloy implants in groups I and II, and much more bacteria colonized on the surface of Ti in group III than in group IV. The results were further verified by the spread plate method (Fig. 7B).
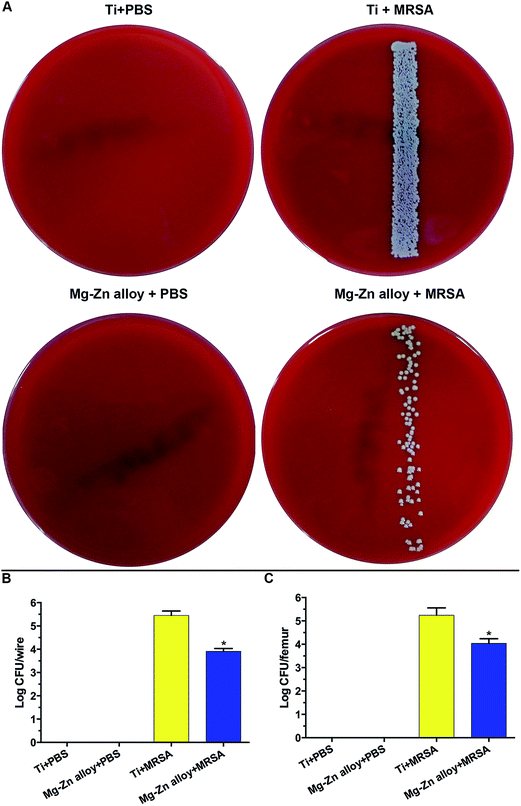 |
| Fig. 7 Cultures of implants and bone: (A) roll-over cultures of MRSA from the extracted Mg–Zn alloy and Ti Kirschner wires. (B) Counting of adherent MRSA on the surfaces of implants using the spread plate method. (C) Counting of MRSA from ground distal femur after implants extraction. *P < 0.05 compared to group III. | |
Consistent with the above results, no bacteria were cultured from the bone tissue in group I or II and the average number of CFU/femur in group IV was significantly lower than that in group III (Fig. 7C).
3.6.4. Histological evaluation. As shown in Fig. 8, more new bone formation and enhanced bone-to-implant contact were observed around the Mg–Zn alloy implants in group II than the Ti implants in group I. Obvious osteolysis and sequestra were observed around the Ti implants in the presence of MRSA in group III. However, there was still new bone formation around the Mg–Zn alloy implants in the presence of MRSA in group IV even though slight osteolysis and a few sequestra were observed.
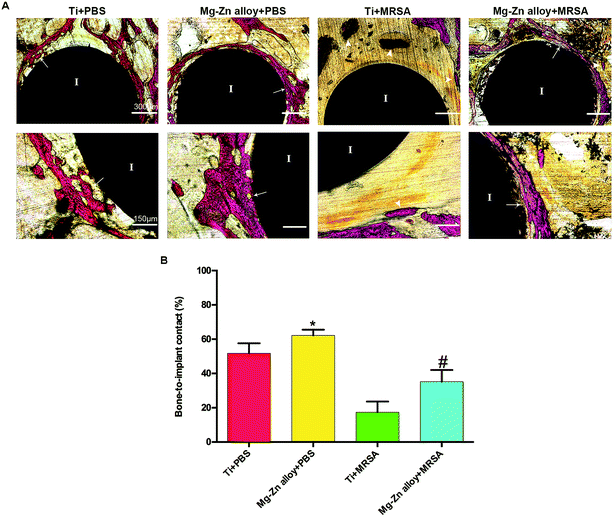 |
| Fig. 8 (A) Histological photographs of Van Gieson's stained bone tissue around the implants. ‘I’ represents the implant location, white arrows show the location of newly formed bone, and arrowheads represent the sequestrum. (B) The bone-to-implant contact values 8 weeks post-implantation in the distal femur. *p < 0.05 compared to group I, #p < 0.05 compared to group III. | |
4. Discussion
Poor integration of implants and risk of implant-associated infection are the two major obstacles to the enduring performance of orthopedic implanted materials.28 Orthopaedic implants possessing both osteogenic activity and antibacterial ability are desirable for better clinical performance. In recent years, much progress has been made in the development of biodegradable magnesium alloys in biomedical applications. The osteogenic activity and antibacterial ability of magnesium alloys have each been confirmed.7,17 In this study, we investigated the osteogenic activity and antibacterial ability of a Mg–Zn alloy simultaneously for the first time.
First, we focused our study on the cytocompatibility and osteogenic activity of the extract of the Mg–Zn alloy. Our data showed that cell proliferation was not inhibited in the presence of the Mg–Zn alloy extract, which was consistent with the literature,12 indicating that the Mg–Zn alloy is safe as an implantable material. The pH and magnesium ion concentration of the extract were 8.07 and 17.74 mM, respectively. Monfoulet et al. demonstrated that the pH within the range (specifically, 7.9–8.27) do not inhibit the proliferation of hBMSCs.29 Moreover, it was reported that magnesium ions within the range (0–70 mM) do not adversely affect the proliferation of osteoblasts.30 Furthermore, the osteogenic activity of the Mg–Zn alloy was investigated by ALP assays, Alizarin red staining and real-time qPCR. Both ALP expression and ECM mineralization of rBMSCs cultured in the extract were enhanced compared with the control group. Higher expression of osteogenesis-related genes including Runx2, OSP and OCN was observed at day 7 in the Mg–Zn alloy extract group in comparison with the control. The above results demonstrated enhanced osteogenic activity of rBMSCs in the presence of the Mg–Zn alloy extract. It was assumed that the enhanced osteogenic activity of rBMSCs was attributed to the synergistic effects of the alkalinity, Mg and Zn ions from the Mg–Zn alloy. Yoshizawa et al. reported that Mg2+ stimulates the osteogenesis of BMSCs possibly via hypoxia-inducible factor (HIF)-2α in undifferentiated BMSCs and via peroxisome proliferator-activated receptor gamma coactivator (PGC)-1α in osteogenic cells.11 The osteogenic activity of the Mg–Zn alloy can be partly ascribed to the Zn ions, which can stimulate bone formation and increase osteogenic function in osteoblasts by stimulating cell proliferation, alkaline phosphatase activity, collagen synthesis and osteoblast marker gene expression.31–33 Jin et al. showed that the implantation of Zn using plasma immersion ion implantation technology enhanced the osteogenic activity of titanium alloys and that Zn ions at approximately 0.26 ppm were able to promote the osteogenesis of rBMSCs.34 In this work, the Zn ion concentration of the extract was 4.2 μM (0.27 ppm), which is in good agreement with the above research results. However, it was reported that there is a pH range (specifically, 7.9–8.27) at which the osteogenic differentiation of human BMSCs is adversely affected.29 The pH value of the extract of the Mg–Zn alloy was 8.07, which is within the above range. The relatively high pH of the Mg–Zn alloy extract might be correlated with the drop of the cDNA expression on day 14.
Further, we demonstrated that the Mg–Zn alloy decreased the viability of MRSA and reduced the colony forming units over 1- and 3-day incubation periods compared with Ti, and its antibacterial effect increased with extended incubation time. We speculated that the antibacterial ability of the Mg–Zn alloy could be ascribed to the synergistic effects of high alkalinity and Zn ions. The antibacterial rate at day 3 was significantly higher than that at day 1. The higher pH and higher Zn ion concentration in the solution might account for the result. Qin et al. demonstrated that pure magnesium prevented bacteria biofilm formation by high alkalinity (pH ≥ 9) at its surface rather than by the high Mg2+ concentration.18 Mg corrosion results in a rapid increase in pH, which produces toxic effects on microorganisms. On one hand, high alkalinity inhibits the adhesion of bacteria by decreasing bacterial surface hydrophobicity,35 on the other hand, high alkalinity disrupts the proton electro-chemical gradient in the intermembrane space of bacteria and reduces the synthesis of ATP by excessive consumption of H+.18
It has been reported that bacterial adhesion and growth can be inhibited by reactive oxygen species (ROS) generated by Zn,36 and Zn ions are able to inhibit transmembrane proton translocation, glycolysis and acid tolerance in bacteria.37 Zinc ions at an appropriate concentration possess both antibacterial and osteogenic activity without introducing undesired side effects.36,38,39 Therefore, Zn on the surface of the Mg–Zn alloy and the Zn ions released with degradation of the Mg–Zn alloy may contribute to enhancing its antibacterial properties. In this study, the Zn ion concentration of the solution was 0.42 and 0.57 ppm at days 1 and 3, respectively. It was reported that Zn ions at a concentration of approximately 0.26 ppm presented partly antibacterial effects on both Escherichia coli and Staphylococcus aureus.34 Although Zn is effective against biofilms, its potency is significantly reduced due to the high biomass concentration in such films,37 which may explain why the Ra value was lower than the Rp value in this work. As far as we know, the Zn content of the Mg–Zn alloy is highest among all of the magnesium alloys, which may strengthen the antibacterial ability of the Mg–Zn alloy. Whether the Mg–Zn alloy has better antibacterial ability than pure Mg or other Mg alloys needs further investigation.
Finally, the in vivo osteogenic activity and antibacterial ability of the Mg–Zn alloy were evaluated using an implant-related femur osteomyelitis model in rats. Tissue responses around implants were evaluated by X-ray photography, micro-CT and histological observation. Little gas was observed around the Mg–Zn alloy implants at week 2 by the X-ray examination, which indicated that the Mg–Zn alloy had good corrosion resistance. Excessive hydrogen gas formation (Mg + 2H2O → Mg(OH)2 + H2)40 may impede the process of osteosynthesis.41 The formation of a fairly stable corrosion product layer on the implant surface leads to a delayed degradation rate with the extension of implantation time,7 which may explain why much less gas was observed at weeks 4 and 6 post-operation. As shown in Fig. 8, better bone-implant integration was observed around the Mg–Zn alloy implants compared with Ti in the absence of MRSA, and significantly obvious osteolysis was observed around the Ti implants compared with the Mg–Zn alloy in the presence of MRSA. It is rational to speculate that better bone-implant integration of the Mg–Zn alloy is mainly attributed to the synergistic effects of Mg, Zn ions and alkalinity on the osteogenic activity and antibacterial ability. Magnesium ions released from the implant are able to enhance osteogenic activity and generate a stimulatory effect on the growth of new bone tissue.7,42,43 Moreover, Janning et al. reported that the major corrosion product, Mg(OH)2, from any magnesium alloy was the major origin of the enhanced bone growth in vivo.44 Encouragingly, there was new bone formation around the Mg–Zn alloy even though the presence of MRSA. Mg–Zn alloy might create the conditions beneficial for the bone-implant integration by inhibiting bacterial infection. Implant-associated infections caused by adhesion and colonization of bacteria may led to osteolysis, implant loosening, inadequate osteointegration and eventual failure of implant.45 The antibacterial ability of the Mg–Zn alloy was further confirmed by bacterial cultures from the implanted wires and bone powder, a significant reduction of bacteria was observed in group IV compared with group III, which was consistent with the in vitro results. The above results indicate that the Mg–Zn alloy could effectively reduce bacterial adhesion and restrain bacterial growth, which bodes well for its clinical application. The antibacterial ability of the Mg–Zn alloy reduces the risk of perioperative infection and fosters normal wound healing in the early stage. Then, with the extension of implantation time, the corrosion product layer deposited on the surface of the Mg–Zn alloy implant retards the corrosion of the implant, which makes the microenvironment more favourable for the establishment of osteointegration.
Based on the in vitro and in vivo studies, the newly developed Mg–Zn alloy exhibited good biocompatibility, osteogenic activity and antibacterial ability. Thus, the Mg–Zn alloy probably has immense potential in orthopaedics and other biomedical applications, especially for promoting osteointegration and preventing implant-associated infections. Although the most commonly used orthopaedic implants are still Ti (alloy) and stainless steel, the mismatch in mechanical properties, insufficient osteointegration and implant-associated infections increase the risk of implant failure. Therefore, the Mg–Zn alloy may be a potential candidate for the next generation of orthopaedic implants.
5. Conclusion
The anti-biofilm ability and osteogenic activity of a Mg–Zn alloy were investigated in vitro and in vivo systematically. The Mg–Zn alloy exhibited synergistic effects in simultaneous anti-biofilm ability and osteogenic activity. New bone formed around the Mg–Zn implants even in the presence of MRSA. The anti-biofilm ability of the Mg–Zn alloy might be associated with increased alkalinity and Zn ions from its degradation; the osteogenic activity of the Mg–Zn alloy might be ascribed to the released magnesium and zinc ions. This research clarified the anti-biofilm and osteogenic properties of the Mg–Zn alloy, which bodes well for potential biomedical applications as the next generation of orthopaedic implants for the prevention of implant-associated infections and osteointegration promotion.
Author contributions
The manuscript was written through contributions of all authors. All authors have given approval to the final version of the manuscript.
Conflict of interest
The authors declare no competing financial interest.
Acknowledgements
Financial support from the National Natural Science Foundation of China (81271961, 81271998), Shanghai Committee of Science and Technology, China (15ZR1431900) are acknowledged.
References
- K. G. Neoh, X. Hu, D. Zheng and E. T. Kang, Biomaterials, 2012, 33, 2813–2822 CrossRef CAS PubMed.
- Y. Zhao, M. I. Jamesh, W. Li, G. Wu, C. Wang, Y. Zheng, K. W. Yeung and P. K. Chu, Acta Biomater., 2014, 10, 544–556 CrossRef CAS PubMed.
- L. Zhao, P. K. Chu, Y. Zhang and Z. Wu, J. Biomed. Mater. Res., Part B, 2009, 91, 470–480 CrossRef PubMed.
- G. Jin, H. Qin, H. Cao, S. Qian, Y. Zhao, X. Peng, X. Zhang, X. Liu and P. K. Chu, Biomaterials, 2014, 35, 7699–7713 CrossRef CAS PubMed.
- J. Nagels, M. Stokdijk and P. M. Rozing, J. Shoulder Elbow Surg., 2003, 12, 35–39 CrossRef PubMed.
- F. Witte, Acta Biomater., 2010, 6, 1680–1692 CrossRef CAS PubMed.
- F. Witte, V. Kaese, H. Haferkamp, E. Switzer, A. Meyer-Lindenberg, C. J. Wirth and H. Windhagen, Biomaterials, 2005, 26, 3557–3563 CrossRef CAS PubMed.
- D. A. Robinson, R. W. Griffith, D. Shechtman, R. B. Evans and M. G. Conzemius, Acta Biomater., 2010, 6, 1869–1877 CrossRef CAS PubMed.
- J. Y. Lock, M. Draganov, A. Whall, S. Dhillon, S. Upadhyayula, V. I. Vulley and H. Liu, Conf. Proc. IEEE Eng. Med. Biol. Soc., 2012, 2012, 1378–1381 Search PubMed.
- F. Witte, N. Hort, C. Vogt, S. Cohen, K. Kainer, R. Willumeit and F. Feyerabend, Curr. Opin. Solid State Mater. Sci., 2008, 12, 63–72 CrossRef CAS.
- S. Yoshizawa, A. Brown, A. Barchowsky and C. Sfeir, Acta Biomater., 2014, 10, 2834–2842 CrossRef CAS PubMed.
- S. Zhang, X. Zhang, C. Zhao, J. Li, Y. Song, C. Xie, H. Tao, Y. Zhang, Y. He, Y. Jiang and Y. Bian, Acta Biomater., 2010, 6, 626–640 CrossRef CAS PubMed.
- D. Yin, E. Zhang and S. Zeng, Trans. Nonferrous Met. Soc. China, 2008, 18, 763–768 CrossRef CAS.
- E. Zhang, L. Yang, J. Xu and H. Chen, Acta Biomater., 2010, 6, 1756–1762 CrossRef CAS PubMed.
- G. Jin, H. Qin, H. Cao, Y. Qiao, Y. Zhao, X. Peng, X. Zhang, X. Liu and P. K. Chu, Biomaterials, 2015, 65, 22–31 CrossRef CAS PubMed.
- M. Yamaguchi, M. Goto, S. Uchiyama and T. Nakagawa, Mol. Cell. Biochem., 2008, 312, 157–166 CrossRef CAS PubMed.
- H. Qin, Y. Zhao, M. Cheng, Q. Wang, Q. Wang, J. Wang, Y. Jiang, Z. An and X. Zhang, RSC Adv., 2015, 5, 21434–21444 RSC.
- H. Qin, Y. Zhao, Z. An, M. Chen, Q. Wang, T. Cheng, Q. Wang, J. Wang, Y. Jiang, X. Zhang and G. Yuan, Biomaterials, 2015, 53, 211–220 CrossRef CAS PubMed.
- A. K. John, D. Baldoni, M. Haschke, K. Rentsch, P. Schaerli, W. Zimmerli and A. Trampuz, Antimicrob. Agents Chemother., 2009, 53, 2719–2724 CrossRef CAS PubMed.
- D. C. Oliveira, A. Tomasz and H. de Lencastre, Lancet Infect. Dis., 2002, 2, 180–189 CrossRef CAS PubMed.
- L. Helbig, H. G. Simank, H. Lorenz, C. Putz, C. Wolfl, A. J. Suda, A. Moghaddam, G. Schmidmaier and T. Guehring, Int. Orthop., 2014, 38, 891–897 CrossRef PubMed.
- A. F. Chen, V. M. Schreiber, W. Washington, N. Rao and A. R. Evans, Clin. Orthop. Relat. Res., 2013, 471, 3135–3140 CrossRef PubMed.
- J. T. Torbert, M. Joshi, A. Moraff, P. E. Matuszewski, A. Holmes, A. N. Pollak and R. V. O'Toole, J. Orthop. Trauma, 2015, 29, 7–17 CrossRef PubMed.
- H. Grundmann, M. Aires-de-Sousa, J. Boyce and E. Tiemersma, Lancet, 2006, 368, 874–885 CrossRef.
- S. Bhattacharyya, A. Agrawal, C. Knabe and P. Ducheyen, Biomaterials, 2014, 35, 509–517 CrossRef CAS PubMed.
- L. Xia, Z. Zhang, L. Chen, W. Zhang, D. Zeng, X. Zhang, J. Chang and X. Jiang, Eur. Cells Mater., 2011, 15, 68–82 Search PubMed.
- C. Yang, G. Yuan, J. Zhang, Z. Tang, X. Zhang and K. Dai, Biomed. Mater., 2010, 5, 045005 CrossRef PubMed.
- J. W. Costerton, P. S. Stewart and E. P. Greenberg, Science, 1999, 284, 1318–1322 CrossRef CAS PubMed.
- L. E. Monfoulet, P. Becquart, D. Marchat, K. Vandamme, M. Bourguignon, E. Pacard, V. Viateau, H. Petite and D. Logeart-Avramoglou, Tissue Eng., Part A, 2014, 20, 1827–1840 CrossRef CAS PubMed.
- Y. Lu, P. Han, W. Ji and Y. Chin, J. Orthop. Trauma, 2013, 15, 1065–1070 CAS , in chinese.
- H. J. Seo, Y. E. Cho, T. Kim, H. I. Shin and I. S. Kwun, Nutr. Res. Pract., 2010, 4, 356–361 CrossRef CAS PubMed.
- X. Wang, A. Ito, Y. Sogo, X. Li and A. Oyane, Acta Biomater., 2010, 6, 962–968 CrossRef CAS PubMed.
- K. Yusa, O. Yamamoto, M. Fukuda, S. Koyota, Y. Koizumi and T. Sugiyama, Biochem. Biophys. Res. Commun., 2011, 412, 273–278 CrossRef CAS PubMed.
- G. Jin, H. Cao, Y. Qiao, F. Meng, H. Zhu and X. Liu, Colloids Surf., B, 2014, 117, 158–165 CrossRef CAS PubMed.
- A. Nostro, L. Cellini, M. Di Giulio, M. D'Arrigo, A. Marino, A. R. Blanco, A. Favaloro, G. Cutroneo and G. Bisignano, APMIS, 2012, 120, 733–742 CrossRef CAS PubMed.
- H. Hu, W. Zhang, Y. Qiao, X. Jiang, X. Liu and C. Ding, Acta Biomater., 2012, 8, 904–915 CrossRef CAS PubMed.
- T. N. Phan, T. Buckner, J. Shen, J. D. Baldeck and R. E. Marquis, Oral Microbiol. Immunol., 2004, 19, 31–38 CrossRef CAS PubMed.
- A. Tarushi, G. Psomas, C. P. Raptopoulou and D. P. Kssissoglou, J. Inorg. Biochem., 2009, 103, 898–905 CrossRef CAS PubMed.
- J. Xu, G. Ding, J. Li, S. Yang, B. Fang, H. Sun and Y. Zhou, Appl. Surf. Sci., 2010, 256, 7540–7544 CrossRef CAS.
- M. Pourbaix, Houston texas: National Association of Corrosion Engineers 1974 Search PubMed.
- T. Kraus, S. F. Fischcerauer, A. C. Hanzi, P. J. Uggowitzer, J. F. Loffler and A. M. Weinberg, Acta Biomater., 2012, 8, 1230–1238 CrossRef CAS PubMed.
- H. Zreiqat, C. R. Howlett, A. Zannettino, P. Evans, G. S. Tranzil, C. Knabe and M. Shakibaei, J. Biomed. Mater. Res., 2002, 62, 175–184 CrossRef CAS PubMed.
- A. Brown, S. Zaky, H. Ray Jr and C. Sfeir, Acta Biomater., 2015, 11, 543–553 CrossRef CAS PubMed.
- C. Janning, E. Willbold, C. Vogt, J. Nellesen, A. Meyer-Lindenberg, H. Windhagen, F. Thorey and F. Witte, Acta Biomater., 2010, 6, 1861–1868 CrossRef CAS PubMed.
- Y. Huang, G. Zha, Q. Luo, J. Zhang, F. Zhang, X. Li, S. Zhao, W. Zhu and X. Li, Sci. Rep., 2014, 4, 6172 CrossRef CAS PubMed.
Footnote |
† These authors contributed equally to this work. |
|
This journal is © The Royal Society of Chemistry 2016 |