DOI:
10.1039/C6RA03049A
(Review Article)
RSC Adv., 2016,
6, 42196-42222
Synthesis and sensing applications of polyaniline nanocomposites: a review
Received
2nd February 2016
, Accepted 14th April 2016
First published on 22nd April 2016
Abstract
A comprehensive review on the synthesis of PANI nanocomposites and their applications as gas sensors and biosensors has been presented. The multi-functionality of PANI nanocomposites have been extensively exploited in diverse applications with impressive results. The synergistic effects between the constituents have made these materials particularly attractive as sensing elements for gases and biological agents. Not only do PANI nanocomposites allow room temperature sensing of a large number of combustible or toxic gases and pollutants with high selectivity and sensitivity, they also enable immobilization of bioreceptors such as enzymes, antigen–antibodies, and nucleic acids onto their surfaces for detection of an array of biological agents through a combination of biochemical and electrochemical reactions. Efforts are on towards understanding the working mechanism of PANI nanocomposites which will increase their potential fields of applications.
| Tanushree Sen obtained her M.Sc. in 2006 in Polymer Science. She is presently pursuing Ph.D. from University Institute of Chemical Technology, North Maharashtra University, Jalgaon, Maharashtra, India. Her areas of research are intrinsically conducting polymers, nanocomposites, gas sensors, fiber reinforced plastics, and polymeric blends and alloys. |
| Satyendra Mishra did his Ph.D. from Indian Institute of Technology, Delhi in 1989. He is presently the Director of University Institute of Chemical Technology, North Maharashtra University, Jalgaon, Maharashtra. He has been continually engaged in research activities for the last 30 years. He has over 140 published research papers in various international and national journals. His areas of research are polymer nano composites, water soluble polymers, wood polymer composites, nano-polymers, reaction engineering aspects of depolymerization reactions, nano catalysis and gas sensors. |
| Navinchandra G. Shimpi completed his Ph.D. in 2006. He is presently Associate Professor at the Department of Chemistry, University of Mumbai. He has 37 published research papers in various international and national journals. He has a research experience of 10 years on areas such as polymer nano composites, rubber composites and nano-fillers. |
1. Introduction
Synthetic polymers have been popular as electrically insulating materials for over a century. The discovery of electrically conducting polymers, therefore, heralded a new era in the domain of polymer science. The interest of the scientific community in the electrical conductivity in synthetic polymers found its impetus from the pioneering work of Shirakawa, MacDiarmid and Heeger in the field of conducting polymers, for which they received Nobel Prize in Chemistry in 2000. Since then, the field of conducting polymers has gathered momentum owing to the extensive fundamental research carried out in this arena of science, which today includes a host of conjugated polymers such as polyacetylene (PA), polyaniline (PANI), polypyrrole (PPY), poly(p-phenylene) (PPP), poly(p-phenylenevinylene) (PPV), polythiophenes (PTH) and their derivatives.1,2 These polymers have a backbone of π-conjugated chain, a sequence of alternating single and double bonds (sp2 hybridized structure), which results in delocalization of π-electrons along the entire polymer chain, consequently lending these polymers their special electrical properties.3,4 Due to the inherent ability of these polymers to conduct electricity through charge delocalization they are called intrinsically conducting polymers (ICPs). Fig. 1 shows molecular structures of some of the prominent conjugated polymers.
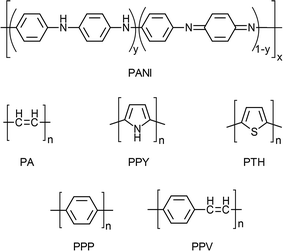 |
| Fig. 1 Molecular structures of typical intrinsically conducting polymers. | |
Amongst all the ICPs, PANI has been the subject of enormous interest to researchers due to its reversible doping/dedoping character, modifiable electrical conductivity, pH switching properties and good environmental stability.5 It also possesses the unique ability to get doped by protonic acids (proton doping) apart from the conventional redox doping. Through the virtue of its molecular self-assembly, PANI often forms supramolecular nanofibers, thereby lending itself to a variety of applications due to the radically different and new properties resulting from the high surface to volume ratio. Several nanostructures of PANI such as nanofibers, nanotubes and nanospheres have been prepared by an array of synthesis methods.6–9 Introduction of a secondary component, such as nanomaterials, into PANI further extends its functionality, offering efficient designs and enhanced performance. It has been experimentally shown that the synergy between the individual components lend the nanocomposite enhanced characteristics, thereby expanding its scope of application.4,10–12 The development of PANI nanostructures and their nanocomposites stems from the desire to explore the full potential of these materials.
The secondary component in a nanocomposite can be metallic or bimetallic nanoparticles, metal oxide nanoparticles, carbon compounds such as CNT or graphene, chalcogenides, polymers, etc.13–27 The nanoparticles within the polymer matrix do not form any coordination bonds but are stabilized by weak coulombic or van der Waal's interactions. Their introduction into PANI may lead to electronic interactions, charge transfers, morphological modifications or a combination of these effects between the constituents of the nanocomposite system.28–31 Such interactions amongst the constituents of the nanocomposite not only improve the existing properties but may also introduce interesting novel features.32 For example, depending on the secondary component the nanocomposite can be multi-functionalized, as in the case of PANI/Fe3O4 nanocomposite which exhibit both conducting and magnetic character.33 However, better dispersion of nanoparticles and an increase in interfacial interaction between the nanomaterial and PANI are integral for improvement in properties (thermal, electrical and optical). Another advantage of a nanocomposite lies in the fact that a very small amount of nanomaterial is generally sufficient to bring about the desired improvement in properties. Hence, the use of nanocomposite is also perceived as being economical.
In this review, we concentrate on the nanocomposites of PANI that have so far been prepared using different nanomaterials, and discuss the various strategies adopted for their preparation. We also focus on the potential applications of PANI nanocomposites in the area of gas sensors and biosensors. Finally, we conclude with a discussion on future research strategies for PANI nanocomposites.
2. Synthesis of PANI nanocomposites
The synthetic strategies for preparation of nanocomposites are of great importance as they impinge upon the resulting product in terms of morphology and properties, and consequently their applications. Most of the methods that are in use for nanocomposite preparation are generally based on two routes: (i) one-step redox reactions where simultaneous polymerization of aniline and formation of nanoparticle takes place or (ii) in situ polymerization where pre-synthesized nanoparticles are mixed into the monomer solution followed by chemical or electrochemical polymerization. Building on these two routes, nanocomposites have been prepared by both conventional and innovative approaches. In all the methods, however, one of the key criteria is the precise control of size and composition. Table 1 lists some of the synthesis methods employed in preparation of PANI nanocomposites, divided into categories based on nanomaterial type. These synthesis methods are discussed in detail expounding on the advantages and disadvantages of each method in the following section.
Table 1 Summary of synthesis methods for different types of PANI nanocomposites
Nanocomposite type |
Secondary component |
Synthesis method |
References |
PANI/metal |
Au |
In situ interfacial polymerization involving one-step redox reaction |
35 |
Pt |
In situ interfacial polymerization involving one-step redox reaction |
36 and 38 |
Pt |
Chemical and electrochemical synthesis by one-step redox reaction using K2PtCl6 as oxidant |
39 |
Ag |
In situ chemical polymerization yielding core–shell morphology of PANI/Ag |
40 |
Ag |
Ultrasound assisted miniemulsion method yielding Ag nanoparticles 5–10 nm in size |
41 |
Ag |
Ultrasound-assisted reduction of Ag precursor followed by in situ polymerization of PANI in presence of nanosilver |
42 |
Ni |
Aniline stabilized Ni particles prepared via ligand exchange reaction with aniline followed by polymerization |
43 |
Pd |
Electroless surface deposition of Pd nanoparticles on electro-polymerized aniline on Ti electrode |
44 |
Pd |
Sulphonated polystyrene nanofibers, used as template, coated over Pd nanoparticles followed by PANI coating and template removal |
45 |
PANI/metal oxide |
Fe3O4 |
Micelle assisted method involving in situ nanoparticle formation |
46 |
TiO2 |
Template synthesis |
48 |
γ-Fe2O3 |
In situ polymerization of aniline in presence of sonochemically prepared γ-Fe2O3 nanoparticles |
54 |
ZnO |
Chemisorptions of PANI on the surface of hydrothermally grown ZnO |
47 |
ZnO |
Physical mixture of PANI and ZnO nanoparticles |
58 and 59 |
NiO |
NiO nanoparticles embedded in PANI matrix via in situ polymerization |
52 |
Co3O4 |
In situ interfacial polymerization (in presence of Co3O4 nanoparticles) |
53 |
V2O5 |
V2O5 acts as template and oxidant for aniline polymerization yielding core–shell morphology |
55 |
V2O5 |
Hydrothermal synthesis of PANI–V2O5 nanosheets of thickness 10–20 nm via in situ intercalation polymerization |
56 |
RuO2 |
Reduction of Ru(III) to Ru(II) forming ruthenium(II)–tetraaniline complex followed by composite formation by oxidation with H2O2 |
57 |
PANI/CNT or graphene |
CNT |
Cable-like structure of PANI/CNT formed via in situ polymerization with CTAB functionalized CNT |
60 |
CNT |
Electrospinning |
65 |
CNT |
CNT functionalized by carboxylic acid followed by in situ polymerization |
68 |
Graphene |
Amination of graphene sheet followed by covalent grafting with PANI |
69 |
Graphene |
Polymerization of aniline on the surface of poly(styrenesulphonic acid) functionalized graphene with the sulphonic acid acts as dopant for PANI |
70 |
Graphene |
Polymerization of anilinium dodecylsulphate (both a surfactant and a monomer) over graphene structure |
61 |
Graphene |
Polymerization of aniline in presence of graphene oxide followed by its reduction to graphene |
73 |
Graphene oxide |
Surfactant-less method gives sandwich-like morphology |
71 |
Graphene oxide |
In situ polymerization of aniline in presence of graphene oxide sheets yielded pH dependent morphologies |
72 |
PANI/chalcogenides |
CdS |
Polymerization of DBSA doped PANI followed by synthesis of CdS nanoparticles by co-precipitation |
74 |
CdS |
In situ polymerization of aniline in presence of CdS prepared by sol–gel technique |
75 |
ZnS |
Electrochemical polymerization of aniline in presence of ZnS nanoparticles |
76 |
CdSe |
Ultrasound assisted inverse microemulsion method for nanocomposite preparation of PANI with CdSe quantum dots |
78 |
PANI/phthalocyanines or porphyrins |
Phthalocyanines |
Electrostatic LBL technique forming multilayer composite |
79 |
Phthalocyanines |
LBL films of PANI and Ni-tetrasulphonated phthalocyanines |
80 |
Porphyrins |
J-aggregates assembled from Co-porphyrin acts as template for PANI electropolymerization giving rod-like morphology |
82 |
PANI/polymers |
PVA |
APS soaked PVA hydrogel immersed into aniline hydrochloride solution leads to formation of PANI in the bulk and on the surface of PVA |
83 |
PVA |
PVA solution was spin coated on a FTO substrate followed by dip coating of PANI |
86 |
PVAc |
Electrospinning |
88 |
PMMA |
Solution casting of PANI nanofibers dispersed in PMMA/butanone solution |
87 |
PMMA |
Electrospinning of mixture of CSA doped PANI and PMMA solutions |
89 |
PANI/multicomponent |
CNT/graphene |
In situ polymerized PANI/CNT dispersed in graphene oxide followed by its reduction to graphene |
90 |
Graphene oxide/CNT |
PANI formed by anodic electropolymerization deposited on reduced graphene oxide/CNT papers |
93 |
Fe3O4/CNT |
Deposition of Fe3O4 on CNT followed by in situ polymerization of aniline |
94 |
γ-Fe2O3/CNT |
Coating of CNT and PANI/γ-Fe2O3 nanocomposite onto cotton thread |
95 |
CNT/CoFe2O4 |
In situ chemical polymerization yielding reticular branched structure |
96 |
Graphene oxide/CdSe |
Graphene oxide/PANI composite formed by in situ polymerization followed by deposition of L-cysteine modified CdSe |
97 |
SnO2–ZnO |
PANI coated solvothermally prepared SnO2–ZnO nanoparticles coated over alumina |
98 |
Pt–Pd |
In situ polymerization of aniline in presence of PVP stabilized Pt–Pd colloids |
101 |
Fe3O4–Au |
In situ chemical polymerization in presence of mercaptocarboxylic acid which acts as template |
102 |
PMMA/Ag |
Electroless coating of Ag over PMMA capped PANI |
103 |
PU–PMMA |
PANI shell over a core of PMMA/PU |
104 |
PU–PMMA |
Interpenetrating network of PANI filled PU–PMMA |
105 |
2.1. Nanocomposite of PANI with metal nanoparticles
The reversible doping/de-doping character of PANI can be used to advantage for the preparation of PANI/noble metal nanocomposites. As the standard reduction potential of most noble metal salts is higher than that of aniline, one-step redox reaction can be carried out to oxidize aniline with simultaneous reduction of the noble metal salts to give zero-valent noble metal nanoparticles. The resulting nanocomposite has the metal nanoparticles embedded into the PANI matrix. This method has been used to prepare nanocomposites of PANI with Ag, Au, Pt, Cu, etc.34–37 Cho and coworkers38 have fabricated PANI/Pt nanocomposite by interfacial polymerization in poly(styrenesulphonic acid) solution with H2PtCl6 serving as both an oxidizing agent and a Pt precursor. The microscopy study suggested a correlation between the molar ratio of Pt and aniline monomer and the nanocomposite's morphology; while an excess of aniline concentration prompted secondary growth of PANI chains, a too small amount caused non-homogenous distribution of Pt nanoparticles in the polymer matrix. An alternative to chemical polymerization is electrochemical polymerization, as reported by Kinyanjui et al.39 Their investigation revealed a more uniform particle size of Pt formed as compared with electrochemical polymerization. While the redox synthesis method for insertion of nanoparticles into polymer matrix offers the advantage of composition control it is limited in it selection of metal precursors which are required to have a reduction potential higher than that of aniline.
In situ polymerization is another route to nanocomposite fabrication. It is the most commonly employed method because it provides a better size and shape control than the one-step redox method. Jing and coworkers40 have reported an in situ chemical polymerization method for PANI/Ag nanocomposite with core–shell morphology. Barkade et al.41 too employed the in situ polymerization route for nanocomposite preparation through ultrasound assisted miniemulsion polymerization of aniline in presence of pre-synthesized Ag nanoparticles. They reported the formation of PANI/Ag nanocomposite with small sized Ag nanoparticles (5–10 nm) embedded in the PANI matrix. The in situ polymerization technique allows local interaction between nanoparticles and the amine and imine units of PANI which consequently results in remarkably different and superior properties of the nanocomposite. Our group reported the synthesis of PANI/Ag nanocomposite via in situ chemical polymerization.42 To ensure uniform dispersion of Ag nanoparticles in PANI matrix the nanoparticles were sonochemically dispersed in aniline hydrochloride solution followed by polymerization of aniline by addition of APS. The TEM micrograph for the nanocomposite is presented in Fig. 2. Microscopy studies indicated that ultrasound irradiation aided not only in uniform dispersion of nanoparticles throughout the PANI matrix but also effectively suppressed secondary growth of PANI thereby yielding nanofibers with small diameters of ∼90 nm.
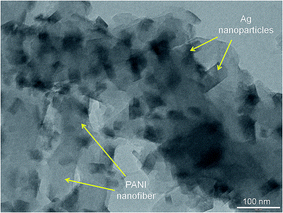 |
| Fig. 2 TEM micrograph showing uniformly dispersed Ag nanoparticles in PANI matrix. | |
Metal nanoparticles can also be synthesized on the surface of PANI. Towards this end, stabilization of the nanoparticles by monomer is an interesting way to achieve monodispersed nanoparticles. Houdayer et al.43 described a method for preparation of Ni/PANI nanocomposite for use as catalyst in Heck couplings. They first prepared zero-valent Ni particles by reduction of nickel acetate with sodium tert-butoxide activated sodium hydride in refluxing tetrahydrofuran (THF). A ligand exchange reaction with aniline gave aniline stabilized Ni particles. The composite was then prepared by polymerization of aniline with APS. Microscopy study revealed the Ni nanoparticles to be uniformly dispersed on the surface of PANI. The presence of such catalytically active metals on PANI surface makes the nanocomposite usable in catalytic applications. In yet another instance, Hosseini et al.44 demonstrated fabrication of PANI/Pd nanocomposites by electroless method in which the Pd nanoparticles were deposited on the surface of PANI. This they accomplished by electro-polymerization of aniline on the surface of Ti electrode, and then immersing the PANI/Ti electrode in electroless-plating bath containing palladium(II) chloride, a complexing agent and a reducing agent. SEM micrograph of Pd nanoparticles deposited on PANI film is shown in Fig. 3. Such uniformly dispersed nanoparticles in polymer matrix or on its surface is necessary to ensure optimum performance of the resulting nanocomposite in its field of application.
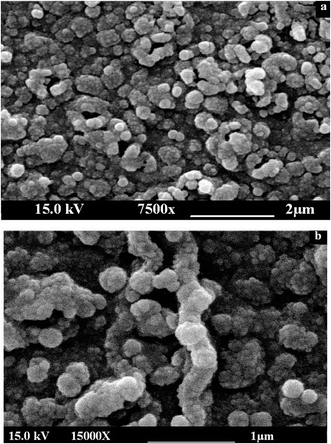 |
| Fig. 3 SEM images of Pd-NPs deposited on the PANI film with different magnifications (a and b).44 (Copyright © 2012 by John Wiley & Sons, Inc. This material is reproduced with permission of John Wiley & Sons, Inc.) | |
Template technique involving use of soft templates is yet another way to fabricate different nanostructures. Kong et al.45 have reported synthesis of PANI/Pd nanotubes using polystyrene nanofibers as templates. The Pd nanoparticles were first coated with sulphonated polystyrene nanofibers followed by PANI coating over it. The template nanofibers were then removed by dissolution in THF. The TEM images revealed that Pd nanoparticles were attached to the inner walls of PANI nanotubes. Although a facile way to prepare different nanostructures, the template technique poses problem in retaining the morphology due to the necessary template removal step.
2.2. Nanocomposite of PANI with metal oxide nanoparticles
Nanocomposites of PANI with metal oxide nanoparticles have the potential to serve as energy storage devices owing to the synergistic effect between the constituents. While the ICPs, such as PANI, serve as excellent electrode material having both electrochemical double layer capacitance and pseudocapacitance arising out of π-conjugated polymer chain, the problem of low conductance and volumetric shrinkage during ion ejection is addressed by the addition of metal oxides. Basavaiah et al.46 described the fabrication of rod-like PANI nanostructures with nanoparticles of magnetite via micelle-assisted one pot synthesis. They first added a mixture of ferric chloride and ferrous sulphate solution to DBSA-doped PANI solution and followed it with addition of ammonium hydroxide at high temperature.
In certain synthesis methods, the PANI is grown on the surface of the nanoparticles. Zhu et al.47 have reported a two-step process for synthesis of PANI/ZnO nanograss. Their method involved chemisorption of PANI on the surface of hydrothermally grown ZnO on fluorine-doped tin oxide (FTO) substrate. A schematic of synthesis procedure for nanograss formation along with their FE-SEM micrograph is presented in Fig. 4. It was observed that the deposition of PANI on ZnO nanostructure with high aspect ratio did not alter the latter's morphology thereby rendering the hybrid system suitable for solar cell performance.
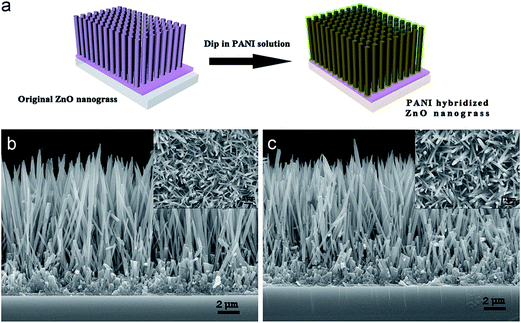 |
| Fig. 4 (a) The schematic procedure of PANI hybridizing ZnO nanograss, (b) side view FE-SEM images of ZnO nanograss obtained by hydrothermal methods, and (c) hybridized sample with 100 mg L−1 PANI. The inset images show top views (Reprinted with permission47). | |
Researchers have also used surfactants as soft templates in order to obtain composite nanostructures. Wang and coworkers48 reported the synthesis of TiO2/PANI core–shell structure using different surfactants as soft templates. They noted that the type of surfactant influenced the morphology of the nanocomposite. The SEM images revealed the formation of core–shell morphology facilitated by the surfactants which enhanced the interfacial interaction between the TiO2 surface and aniline hydrochloride. Several reports of template-free synthesis methods for fabrication of PANI nanocomposites can be found in literature.49–53 We reported the fabrication of PANI/γ-Fe2O3 nanocomposite via in situ polymerization method using a binary dopant system involving protonic acid and a surfactant.54 Our investigations demonstrated that the binary dopant system not only increased charge density in the polymer but also influenced the PANI's size and morphology. The SEM images, shown in Fig. 5, revealed that the γ-Fe2O3 nanoparticle content not only affected the diameter of the PANI nanofibers but also promoted secondary growth of PANI.
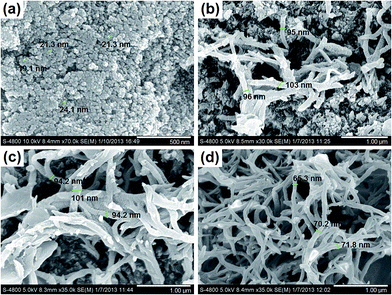 |
| Fig. 5 FE-SEM micrographs of (a) γ-Fe2O3 nanoparticles (500 nm) and PANi/γ-Fe2O3 nanocomposites at 1 wt% (b), 2 wt% (c) and 3 wt% (d) (1 μm) (Reprinted with permission54). | |
Oxidant or initiator free synthesis of PANI nanocomposite can also be found in literature. Nanobelts of PANI/V2O5 composite with core–shell morphology were prepared in which V2O5 itself acted as a template as well as oxidant for aniline polymerization.55 Typical of aniline self-assembly, the morphology was found to be influenced by pH of the solution. These same constituents, prepared under different reaction conditions, can yield different morphologies. Pang and coworkers56 reported nanocomposite formed by intercalation of PANI chains between layered V2O5 through in situ polymerization under hydrothermal conditions. Here again, the microscopic studies suggested that the reaction conditions influenced the morphology of the nanocomposite. For example, nanosheets were formed at high temperature whereas at room temperature rod-like aggregates were obtained. In this case, self-assembly of nanosheets resulted in tremella-like structure. The effect of synthesis method as well as synthesis parameters resulting in morphological variations involving the same set of constituents has been recorded by many researchers.
A somewhat different approach to nanocomposite fabrication was taken by Rao and Vijayan.57 They synthesized PANI/RuO2 nanocomposite via chemical oxidation of ruthenium(II)–tetraaniline complex by H2O2 in presence of HCl. NMR spectroscopic analysis indicated the formation of a tetraaniline complex along with reduction of Ru(III) to Ru(II). Oxidation with H2O2 resulted in simultaneous formation of PANI and RuO2 with sponge-like morphology.
Nanocomposites have also been prepared by physically mixing PANI with nanoparticles. Patil and co-workers58,59 have demonstrated that the optical property of the physical mixture of PANI/ZnO nanocomposite is much different from its constituents. As sensors, these nanocomposites also exhibited superior performance than their constituents.
2.3. Nanocomposite of PANI with CNT or graphene
Graphenes are atom thick layers of carbon exhibiting exceptional properties. Theses layers or sheets when rolled at specific chiral angles form carbon nanotubes (CNT). CNTs possess extremely high aspect ratio, and show extraordinary thermal, electrical and mechanical properties, which are dependent on the rolling angle and radius. When incorporated into PANI they improve its properties and also render it useful in various applications. Deposition of PANI layer onto 1D CNT nanostructure is the simplest method of nanocomposite preparation, and is often achieved by attaching functional groups on the CNT surface or using surfactants. Zhang et al.60 have described an in situ polymerization synthesis of PANI/CNT nanocomposite with cable-like structures using the cationic surfactant CTAB. Microscopic studies suggested that the formation of PANI took place on the surface of MWCNT and that the cable-like structures were a result of CTAB directed self-assembly of PANI. The Raman spectroscopic studies indicated a site-selective interaction of CNT with PANI moieties. Such observation has been made by several researchers in case of in situ polymerization.
Similar to CNTs, graphenes too are incorporated into PANI to obtain nanocomposite with superior electroactivity. The use of surfactant in these cases is to facilitate exfoliation of graphene sheets in the PANI matrix. Vega-Rios et al.61 demonstrated the use of anilinium dodecylsulphate both as a surfactant and a monomer; polymerization of aniline took place directly over the flake-like graphene structures. The advantage of the in situ technique lies in its adaptability to a number of methods for nanocomposite preparation, as has been demonstrated by several research groups.62–64
Electrospinning is another method for fabricating PANI/CNT nanocomposite.65,66 Highly aligned nanofibers can be obtained by controlling electrospinning parameters such as applied voltage, speed of rotating mandrel, distance between tip and collector, etc. Shin and coworkers67 reported the fabrication of nanocomposite of CNT with a blend of PANI/PEO by electrospinning. Microscopic study revealed the highly aligned nature of the nanocomposite with the MWCNTs dispersed inside the PANI matrix. The highly aligned MWCNTs inside the PANI matrix imparted the nanocomposite a significantly high conductance value. Although the process of electrospinning is cumbersome due to the large number of variables controlling the product morphology, it nevertheless remains a process of choice to produce highly aligned electrospun mats and nanofibers.
Functionalization of CNTs prior to nanocomposite formation is yet another variation in synthesis technique which ensures a strong interaction between CNT and the polymer thereby facilitating charge transfer. Kar and Choudhury68 described the effect of functionalized CNTs on the resulting nanocomposite with PANI. They prepared CNT/PANI nanocomposite by incorporating carboxylic acid functionalized MWCNT into PANI. The morphological analysis (Fig. 6) showed a well embedded CNT in PANI matrix suggesting that surface modification of MWCNT with carboxylic acid functional groups improved their dispersion in the monomer solution leading to enhanced interaction between the matrix and CNT. Furthermore, Raman spectroscopic study revealed that the strong interaction between the functionalized MWCNTs and PANI chains resulted in electron delocalization, and hence, doping of PANI by the CNTs.
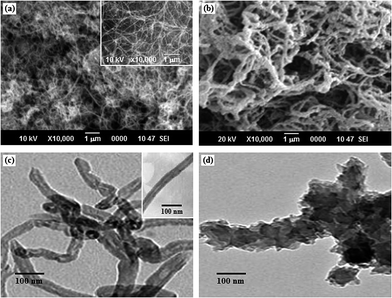 |
| Fig. 6 SEM (a) and (b) and dark field TEM (c) and (d) images of pristine MWCNT (inset), carboxyl-functionalized MWCNT and 2 wt% MWCNT-contained PANI/cMWCNT nanocomposite (Reprinted with permission68). | |
In case of graphene too, researchers have endeavoured to address the challenge of incorporation of graphene into PANI without sacrificing its conducting nature. In one method, diazotization followed by amination of graphene sheet is carried out prior to covalently grafting it with PANI in order to prevent damage of graphene sheets and their aggregation.69 While the carboxylic and hydroxyl groups on the GO surface enhance interfacial interactions with the polymer matrix, they also make it non-conducting which limits their application. The formation of amine functional groups not only increased the conductivity of graphene but also retained nucleation sites for aniline polymerization. In yet another study, nanocomposite was prepared by polymerization of aniline on the surface of poly(styrenesulphonic acid) functionalized graphene.70 Interestingly, this poly(styrenesulphonic acid) coated graphene was found to effectively dope PANI. Moreover, the resulting nanocomposite was readily dispersible in water.
Graphene oxide (GO) too have been used in PANI matrices. Sandwich-like morphology of GO/PANI has been obtained when prepared in absence of surfactants.71 In fabrication of GO/PANI nanocomposite by in situ polymerization Huang and Lin72 reported that reaction pH greatly influenced the morphology of the resulting nanocomposites – while low acidity yielded nanotubes and nanospheres, aligned nanofibers were obtained at high acidity levels. Preparation of nanocomposite by polymerization of aniline in presence of GO and further reduction by hydrazine hydrate has been described by Li et al.73 These nanocomposites were found to have high adsorption capacity of Hg(II) in aqueous solutions.
2.4. Nanocomposite of PANI with chalcogenides
Chalcogenides are compounds of sulphides, selenides and tellurides. When combined with PANI they significantly improve its optical property to a large extent. Nanocomposites of PANI and chalcogenides (such as CdS, ZnS and CdSe) have potential application in energy devices and sensors. Ameen et al.74 described the formation of CdS on the surface of PANI nanorods. They first prepared DBSA doped PANI and then synthesized CdS nanoparticles in the same polymerization reaction mixture by co-precipitation. The synthesized nanocomposite exhibited improved electrochemical behaviour. An in situ technique for CdS/PANI preparation was described by Raut et al.75 where CdS was first prepared by sol–gel technique, followed by polymerization of aniline. The nanocomposites were found to have a lower band gap than neat PANI. Similar reports of PANI/ZnS and PANI/CdSe can be found in literature.76,77 Haldorai et al.78 reported a two-step ultrasound assisted dynamic inverse microemulsion method for nanocomposite preparation of PANI with CdSe quantum dots. The TEM images revealed uniformly dispersed CdSe quantum dots of an average size of 5 nm in the PANI matrix. The nanocomposite formed had high thermal stability as compared to neat PANI. The combination of PANI and CdSe lowers the latter's luminescence lifetime.
2.5. Nanocomposite of PANI with phthalocyanines and porphyrins
Phthalocyanines are macrocyclic compounds with metal centers (coordination complex), while porphyrins are heterocyclic macrocycles containing four pyrrole subunits interconnected by methane bridges. Porphyrins, in general, exhibit good photoluminescence and electrocatalytic properties. Their incorporation into PANI decreases its conductivity but their nanocomposites find applications in fields like sensors and light emitting diodes. Synthesis of hybrids of PANI with tetrasulphonated metal phthalocyanines by LBL technique can be found in literature.79,80 Zucolotto and coworkers79 reported nanocomposites of PANI with sulphonated pthalocyanines prepared by LBL technique. The FTIR spectroscopic study indicated towards a molecular-level interaction between the metal ion containing phthalocyanines and PANI. Porphyrins too are used in PANI for sensing of compounds.81 Zhou et al.82 reported the preparation of PANI/cobalt–porphyrin nanocomposite by one-step electrochemical synthesis. Microscopy study revealed a porous structure with nanorods of Co-porphyrin. They demonstrated the formation of J-aggregates in acidic aqueous solution that served as templates for electropolymerization of aniline. The combined properties of the two components in the nanocomposite render it multifunctional for applications in diverse fields.
2.6. Nanocomposite of PANI with polymers
Polymers such as polyvinyl alcohol (PVA), polyvinyl acetate (PVAc) and polymethyl methacrylate (PMMA) have been extensively made into nanocomposites with PANI. The incorporation of these polymers induce thermal and mechanical stability as well as processability. An in situ method for composite formation using PVA hydrogel has been reported by Adhikari and Banerji.83 The method involved immersing APS soaked PVA hydrogel into aniline hydrochloride solution leading to formation of PANI in the bulk and on the surface of PVA. A similar approach was taken by Bajpai et al.84 for preparing PANI/PVA nanocomposite. Another in situ technique has been offered by Arenas and coworkers85 for formation of aqueous suspension of PANI/PVA system, which involved the use of a surfactant or an organic acid to improve solubility of the nanocomposite. Patil et al.86 too prepared a highly stable PANI/PVA film by a two-step process. First, a PVA solution was spin coated on a FTO substrate and then PANI was dip coated onto this substrate by aniline polymerization. The nanocomposite film showed excellent electrochemical stability without any loss of specific capacitance. Araujo et al.87 described a two-step method for preparation of nanocomposite of PANI with PMMA. PANI nanofibers synthesized by interfacial polymerization were sonochemically dispersed butanone and then mixed with PMMA/butanone solution. This was followed by solution casting this mixture to obtain the nanocomposite film.
Electrospinning was employed by Panthi and coworkers88 for preparation of PANI/PVAc nanocomposite mats. They, however, first individually dissolved PANI and PVAc in THF and DMF, respectively, and then mixed the two solutions and electrospun using silicon substrate as a collector. PANI/PMMA fibers were also prepared by electrospinning, as demonstrated by Veluru et al.89 They prepared PANI by chemical polymerization at low temperature, and then mixed it with CSA in chloroform in a homogenizer for several hours. This PANI solution was then mixed with PMMA and electrospun. As discussed before, the process of electrospinning allowed controlling fiber morphology to yield highly aligned fibers.
2.7. Nanocomposite of PANI with multi-components
PANI nanocomposites with multi-components (more than one type of nanomaterial) have been prepared in order to obtain multi-functional materials which show different properties (like electrical, optical and magnetic property) within the single material, or induce significant improvement in an existing property. Such hybrid materials, consequently, project a greater scope of applications. The most widely prepared PANI multi-component systems are based on PANI/CNTs. A graphene/PANI/CNT double-layer capacitor with hierarchical structure has been fabricated by Lu et al.90 In the first stage they prepared PANI/CNT by in situ polymerization. In the next stage, the PANI/CNT dispersion was mixed with an aqueous dispersion of GO via sonication. After several hours, the flocculated mixture of GO/PANI/CNT was stabilized by adjusting its pH, and then centrifuged to obtain GO/PANI/CNT precursor. This was followed by reduction of GO to graphene by hydrazine gas. The reduced PANI was then re-doped to restore its electrical conductivity. Microscopic studies, as shown from SEM and TEM images in Fig. 7, showed uniform encapsulation of CNT by PANI forming ID core–shell nanostructures. The researchers also demonstrated that the GO sheets facilitated stable dispersion of PANI/CNT nanocomposite. Fabrication of hybrid material from graphene, PANI and CNT has been reported by other research groups as well using different synthetic techniques.91–93
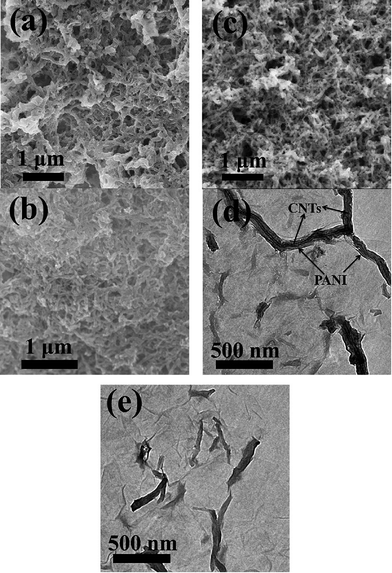 |
| Fig. 7 SEM images of PANI/CNT (a), PANI/CNT-2 (b) and PANI (c); TEM images of GO–PANI/CNT (d) and GO–PANI (e) (Reprinted with permission90). | |
Another type of hybrid composite is based on PANI/CNT with a magnetic material. Wang et al.94 prepared a PANI/Fe3O4/CNT composite for protein digestion by depositing Fe3O4 on CNTs, followed by in situ polymerization of aniline in presence of trypsin. The trypsin immobilization on the composite allows for protein analysis while the superparamagnetic behaviour, due to the presence of Fe3O4, facilitates its isolation from the digests when an external magnetic field is applied. We demonstrated the fabrication of PANI/γ-Fe2O3/CNT hybrid nanocomposite deposited on cotton fibers for sensing application. The strategic protocol described by Shimpi et al.95 for fabrication of the hybrid material involved serial immersion of cotton thread firstly in a CNT colloidal solution and then in a dispersion of PANI/γ-Fe2O3 nanocomposite under ultrasonic irradiation. Microscopic studies revealed deposition of CNT onto the cotton thread with PANI/γ-Fe2O3 uniformly distributed over them. Nanocomposites of PAN/CNT with a tertiary component of magnetic or semiconducting nature have also been reported.96,97 Depending on the synthesis conditions these nanostructures can have different morphologies, and also exhibit properties vastly different from the individual components.
Multi-component systems of PANI with metal oxides can also be found in literature. A hybrid nanocomposite based on SnO2–ZnO/PANI for NO2 sensing has been made by Xu et al.98 where the presence of both SnO2 and ZnO had implications for the response towards NO2. In this case, a solvothermal hot press route was adopted for the preparation of SnO2–ZnO porous nanoparticles. An alumina tube was then coated with a thick paste of SnO2–ZnO composite and sintered. Finally, nanocomposite was prepared by applying a paste of PANI with NMP over the SnO2–ZnO porous nanosolid thick film.
Boomi and co-workers99–101 prepared a series of multifunctional nanocomposites of PANI with different bimetallic nanoparticles by chemical method. These hybrid nanocomposites show better thermal stability (PANI/Ag–Pt) than neat PANI, as well as antibacterial effect. Fe3O4–Au/PANI multifunctional nanocomposite has been synthesized by in situ chemical polymerization in presence of mercaptocarboxylic acid, which acted as a template for the rod-like structures.102 The optical, electrical and magnetic properties exhibited by the nanocomposite were dependent on the molar ratio of Au to Fe3O4.
Lee et al.103 have reported a PMMA/PANI/Ag nanocomposite prepared by coating of PMMA spheres with PANI via in situ chemical polymerization, followed by electroless coating of Ag. The presence of PANI on the PMMA surface facilitated efficient plating of Ag due to high the activation effect. The nanocomposite showed greater thermal stability, and its electrical conductivity increased with Ag content.
Nanocomposite of polyaniline with a blend of other polymers is an economical way to achieve conductivity in polymers. Li et al.104 have prepared PANI/PMMA/PU nanocomposite with core–shell morphology through a two-step polymerization process. In the first step they prepared spheres of PU–PMMA by miniemulsion polymerization using SDS. In the second step a shell of PANI was given over the PU–PMMA core by chemical polymerization of aniline with HCl and DBSA as dopants. Variation in the concentration of dopants yielded different morphologies for PANI. Moreover, increasing concentration of DBSA was responsible for higher electrical conductivity of the nanocomposite as it facilitated better coverage of PANI over the core. Similar nanocomposite of PU–PMMA filled CSA-doped PANI in the form of interpenetrating network (IPN) has been prepared by Jeevananda and Siddaramaiah.105 The morphological analysis of the IPN system revealed two different phases pertaining to the PU and PMMA in the system.
3. Sensing applications of PANI nanocomposites
A sensor is a device which detects and measures a physical quantity and then provides a readable output for it. A sensor's response is the measure of a change in its physical parameter resulting from some chemical stimulation.106 Fig. 8 shows a general response curve for a sensor where x-axis represents ‘activity of analyte’ and the y-axis represents ‘transduction function’. The sensor response varies in the dynamic range, and then saturates beyond the saturation limit. Factors such as reliability, reversibility and selectivity are important in the context of practical applicability of a sensor. Ideally, a sensor response should be reversible, and the sensor should show high selectivity, i.e., it should respond only to one analyte even in the presence of other interfering species.
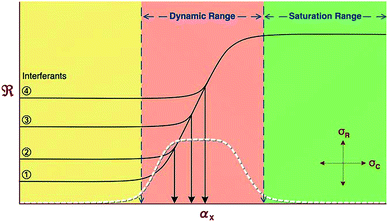 |
| Fig. 8 General response curve of a sensor106 (Reproduced from Principles of Chemical Sensors, Introduction to Sensors, 2009, page 4, Jiri Janata, ©Springer Science + Business Media, LLC 2009. With permission of Springer). | |
PANI is the most important candidate in the field of sensors. As a sensing element it allows monitoring and detection of various analytes in ambient condition (room temperature), which is a safer option compared to sensors operating at high temperatures. Its interaction with analytes influences its redox properties, leading to a change in its resistance, work function or electrochemical potential.107 Immobilization of enzyme or other biological agents on PANI or its nanocomposites is also possible which extends its ambit as a biosensor. Despite its many merits it suffers from low sensitivity and poor selectivity. This issue is often addressed by addition of a secondary component into PANI, which can induce sensitivity or selectivity in PANI, either through effective interaction with PANI or by simply acting as a catalyst. Table 2 presents a list of various PANI based nanocomposites with potential application as gas sensors or biosensors. An in-depth discussion on these PANI based sensors is provided in the following sections.
Table 2 PANI nanocomposite based gas sensors and biosensors
Sensor type |
Analyte |
Nanocomposite used |
Salient features |
References |
Gas sensor |
Methanol |
PANI/Pd |
Response to the order of ∼104 for 2000 ppm methanol; Pd act as a catalyst for reduction of imine nitrogen in PANI by methanol |
108 |
Gas sensor |
Ethanol |
PANI/Ag |
Response > 2.0; response time: 102–52 s for 2.5 mol% Ag |
109 |
Gas sensor |
Triethylamine, toluene |
PANI/Ag |
Sensor response fitted with chemisorption and diffusion model |
110 |
Gas sensor |
Chloroform |
PANI/Cu |
Adsorption–desorption phenomenon at the surface of Cu clusters |
111 |
Gas sensor |
NH3 |
PANI/TiO2 |
High selectivity; response obtained by creation of depletion layer at the heterojunction of PANI and TiO2 |
112 |
Gas sensor |
NH3 |
PANI/SnO2 |
Neat PANI shows reduced behaviour while nanocomposite shows an oxidized behaviour in gas environment |
114 |
Gas sensor |
NH3 |
PANI/SnO2 |
CSA doping increase response from 72% to 91% at 46 s response time |
115 |
Gas sensor |
NH3 |
PANI/PMMA |
Trace level detection (1 ppm) due to PANI coated highly aligned PMMA microfibers |
116 |
Gas sensor |
NH3 |
PANI/sulphonated Ni phthalocyanine |
Ni phthalocyanine catalyzed electrodeposition of PANI influence sensor response |
117 |
Gas sensor |
H2S |
PANI/CuCl2 |
Trace level H2S detection; H2S exerted oxidizing effect on PANI due to preferential binding of CuCl2 with S2− ion with evolution of HCl |
118 |
Gas sensor |
H2S |
PANI–CdS |
Response of ∼48% for 100 ppm H2S |
120 |
Gas sensor |
H2S |
PANI/Au |
Trace level H2S detection (0.1 ppb); formation of H+ ions protonates PANI |
121 |
Gas sensor |
LPG |
CdSe/PANI |
Response ∼70% for 0.08 vol% LPG |
122 |
Gas sensor |
LPG |
PANI/γ-Fe2O3 |
Response: 1.3 for 200 ppm LPG |
54 |
Gas sensor |
LPG |
PANI/TiO2 |
Response ∼63% for 0.1 vol% LPG |
123 |
Gas sensor |
LPG |
PANI/CdSe |
Response ∼80% for 1040 ppm LPG |
124 |
Gas sensor |
LPG |
PANI/ZnO |
Response ∼81% for 1040 ppm LPG |
125 |
Gas sensor |
Water vapour |
PANI/Co |
Response time: 8 s, recovery time: 1 min; water vapour adsorption modifies electrical conductivity |
127 |
Gas sensor |
Water vapour |
PANI/Ag |
Sensor's sensitivity influenced by critical quantum confinement of Ag nanoparticles |
128 |
Gas sensor |
Water vapour |
PANI/Ag–V2O5 |
Increase in conductivity with increasing humidity due to influence of humidity on macromolecular arrangement |
131 |
Gas sensor |
CH4 |
PANI/In2O3 |
Temperature dependant response to CH4 |
133 |
Gas sensor |
CO |
PANI/SnO2 |
Trace level detection of CO (1 ppm) |
134 |
Gas sensor |
CO |
PANI/SWCNT |
Highly selective towards CO in a gas mixture |
135 |
Gas sensor |
NO |
PANI/TiO2/MWCNT |
Detection occurs through decomposition of NO into HNO2, NO2 and HNO3 under UV irradiation |
136 |
Gas sensor |
NO2 |
SnO2–ZnO/PANI |
Temperature dependent response ∼368 at 35 ppm NO2 at 180 °C |
137 |
Gas sensor |
H2 |
PANI/WO3 |
Detection through formation of tungsten–dihydrogen complexes |
140 |
Gas sensor |
H2 |
PANI/PtO2 |
Catalytic oxidation of H2 gas to water decreasing resistance of PANI |
141 |
Gas sensor |
CO2 |
PANI/chloroaluminium phthalocyanine |
Sensitivity range of 0.05–7.20 |
142 |
Biosensor |
Glucose |
Au/PANI |
Sensitivity: 2.3 mA M−1 |
152 |
Detection limit: 5.0 × 10−7 mol L−1 |
Biosensor |
Glucose |
Graphene/PANI/Au |
Reagentless amperometric glucose biosensor |
153 |
Detection limit: 0.6 mM |
Biosensor |
Glucose |
PANI–TiO2 |
Sensitivity: 11.4 μA mM−1 |
157 |
Detection limit: 0.5 μM |
Biosensor |
Cholesterol |
PANI/Au/chitosan |
Sensitivity: 34.77 μA mM−1 cm−2 |
160 |
Detection limit: 1 μM |
Biosensor |
Cholesterol |
PANI/MWCNT |
Sensitivity: 6800 nA mM−1 |
162 |
Detection range: 1.29–12.93 mM |
Biosensor |
Cholesterol |
PANI/CMC |
Sensitivity: 0.14 mA mM−1 cm−2 |
163 |
Detection limit: 1.31 mM |
Biosensor |
Cholesterol |
PANI/Au/graphene |
Coupled enzyme reactions |
164 |
Sensitivity: 0.42 μA mM−1 |
Biosensor |
H2O2 |
PANI/polyester sulphonic acid |
Sensitivity: 1.33 μA μM−1 |
169 |
Detection limit: 0.185 μM |
Biosensor |
H2O2 |
PANI/chitosan |
Linear response range: 1 × 10−5 to 1.5 × 10−3 M |
170 |
Detection limit: 5 × 10−7 M |
Biosensor |
Uric acid |
Fe3O4/chitosan/PANI |
Sensitivity: 0.44 mA mM−1 |
171 |
Detection limit: 0.1 μM |
Biosensor |
Creatinine |
PANI/cMWCNTs |
Tri-enzyme system with creatinine amidohydrolase, creatine amidinohydrolase and sarcosine oxidase |
173 |
Sensitivity: 40 μA mM−1 cm2 |
Detection limit: 0.1 μM |
Biosensor |
Creatinine, urea |
Cu/PANI |
Ammonium ion-specific biosensor; sensitivity: 95 mA M−1 cm−2 (creatinine) and 91 mA M−1 cm−2 (urea) |
175 |
Biosensor |
Salbutamol |
Au nanoparticles, Prussian blue, poly(acrylic acid) and Au–graphene |
Linear response range: 0.08–1000 ng mL−1 |
176 |
Detection limit: 0.04 ng mL−1 |
Biosensor |
Interleukin-6 |
GO/PANI/CdSe |
Linear response range: 0.0005–10 ng mL−1 |
177 |
Detection limit: 0.17 pg mL−1 |
Biosensor |
Prostate-specific antigen |
PANI/Au |
Sensitivity: 1.4 μA M−1 |
178 |
Detection limit: 0.6 pg mL−1 |
Biosensor |
Chlorpyrifos |
Au/PANI/cMWCNTs/chitosan |
Linear response range: 0.1–40 × 10−6 mg mL−1 and 40 × 10−6 to 500 × 10−6 mg mL |
179 |
Detection limit: 0.06 × 10−6 mg mL |
Biosensor |
Estradiol |
Graphene/PANI |
Linear response range: 0.04–7.00 ng mL−1 |
180 |
Detection limit: 0.02 ng mL−1 |
Biosensor |
Benzo[a]pyrene |
Fe3O4/PANI/Nafion |
Linear response range: 8 pM to 2 nM |
181 |
Detection limit: 4 pM |
Biosensor |
LDL |
Au–AgCl/PANI |
Label-free biosensor |
182 |
Detection limit: 0.34 pg mL−1 |
Biosensor |
DNA hybridization |
PANI/GO |
Linear response range: 275 to 551 g mL−1 (ssDNA immobilized electrode) |
183 |
Detection limit: 29.34 g mL−1 |
Biosensor |
BCR/ABL fusion gene (in chronic myelogenous leukemia) |
Au/PANI on GCE |
Detection limit: 2.11 pM |
186 |
Biosensor |
DNA hybridization |
PPY/PANI/Au |
Label-free DNA biosensor |
187 |
Detection limit: 10−13 M |
Biosensor |
Phosphinothricin acetyltransferase gene |
PANI/MWCNT |
Linear response range: 1.0 × 10−13 to 1.0 × 10−7 mol L−1 |
190 |
Detection limit: 2.7 × 10−14 mol L−1 |
Biosensor |
DNA bases |
PANI/cMWCNTs |
Sensitivity: 1.6, 1.9, 1.5 and 2.4 μA cm−2 μM−1 |
192 |
Detection limit: 4.8, 2.9, 1.3 and 1.3 μM |
(For guanine, adenine, thymine, and cytosine, respectively) |
3.1. PANI nanocomposites as gas sensors
Commercially available gas sensors based on metal oxides generally operate at high temperatures. This causes structural changes in the sensing material resulting in instability and response variation. Another risk pertains to the detection of combustible gases or gas mixtures that might auto-ignite at high temperatures. PANI based sensors allow safer detection of a number of combustible gases in ambient conditions (room temperature). The performance of PANI sensors can further be improved by inclusion of nanoparticles which induces sensitivity and selectivity in PANI. Other criteria affecting PANI performance is the its physical form – thin films exhibit higher sensitivity to analytes as compared to pellets. Moreover, nanofibrillar network of PANI through self-assembly gives porous structure caused by features of solid-state polymerization. This structure permits penetration of gaseous molecules into the polymer film from the environment. The diffusion of gas molecules into the polymer and their adsorption onto nanoparticles modifies its electrical behaviour. This is particularly significant in nanocomposite materials whose conductivity is governed by electron transfer between nanoparticles and polymer. Hence, a strong sensor effect is observed in case of PANI nanocomposites resulting from a marked influence of low molecular weight gaseous analytes. Therefore, adsorption of analyte molecules on the sensor surface is the first step leading to their detection, and has been experimentally studied as well.107 Other factors influencing the response of nanocomposites towards analytes are morphology, size, surface area, and nanoparticle content. In the following sections we discuss PANI based nanocomposite sensor designed for different classes of gaseous analytes.
3.1.1. VOCs sensor. Alcohols have a wide range of applications, from solvents and preservatives to antiseptics and fuels. However, alcohol vapour inhalation can have serious health implications as well. Extensive work has been done in detection of alcohol vapours using metal or metal oxide inclusions into PANI matrix. Athawale and co-workers108 reported detection of methanol by PANI/Pd nanocomposite. Their investigation revealed a very high response, to the order of ∼104 magnitudes, for saturated levels of methanol vapour. FTIR spectroscopic study indicated that Pd inclusions act as a catalyst for reduction of imine nitrogens in PANI by methanol. Moreover, in a mixture of VOCs, the PANI/Pd nanocomposite selectively detected methanol with an identical magnitude of response but at a longer response time. Catalytic influence of Ag nanoparticles embedded in PANI matrix in ethanol detection has been reported by Choudhury.109 He demonstrated that faster protonation–deprotonation of PANI takes place on exposure to ethanol in presence of Ag nanoparticles.More recently, PANI/Ag nanocomposite has been employed for detection of triethylamine and toluene.110 They proposed a chemisorption and diffusion model for the sensor response. While they asserted that the sensor response towards triethylamine and toluene is a result of both deprotonation of PANI and swelling of the polymer by the analyte causing a drop in its conductivity, it could not be definitely ascertained which of the two pathways was dominant.
Chloroform is a widely used solvent and an anaesthetic. It is also an irritant and can cause depression of central nervous system upon inhalation. Chloroform monitoring has been reported by Sharma et al.111 using PANI/Cu nanocomposite. They suggested an adsorption–desorption phenomenon at the surface of metal clusters as a possible mechanism. The FTIR spectroscopic study suggested interaction of chloroform with metal clusters. PANI nanocomposites with nanometal inclusions exhibit superior performance than neat PANI in detection of VOCs. These investigations, however, point towards a catalytic pathway as the underlying mechanism.
3.1.2. Sensors for reducing gases – NH3 and H2S. PANI sensor for detection of reducing gases, such as NH3 and H2S, is one of the most researched areas since the underlying sensing mechanism is straightforward, i.e., deprotonation of PANI when exposed to reducing gases through electron donation by the latter, resulting in increased resistance of PANI. Introducing a secondary component further adds to this feature advantageously as it serves to enhance the sensitivity of the sensor or improve its selectivity, or both. Detection of reducing gases by PANI/metal oxide nanocomposites has been extensively studied by various research groups. Metal oxide nanoparticles, when combined with PANI, forms p–n heterojunction with a depletion layer. Adsorption of gases brings out about a change in this depletion region which manifests itself as a change in its electrical property. Pawar et al.112 reported a highly selective NH3 sensor based on PANI/TiO2 nanocomposite. The thin film sensor exhibited gas response towards an NH3 concentration as low as 20 ppm. They proposed that the response was owing to creation of a positively charged depletion layer at the heterojunction of PANI and TiO2. Similar observations on NH3 detection were made by Dhingra et al.113 A different observation, however, was made by Deshpande and coworkers114 who studied the response of PANI/SnO2 nanocomposite towards NH3. They found that while neat PANI gets reduced in NH3 environment, the nanocomposite shows an oxidized characteristic in presence of the gas. The I–V characteristic of the nanocomposite, shown in Fig. 9(c), revealed a diode-like character which is associated with electrical conductance through hopping mechanism. The n-type SnO2 expunges the holes in PANI through formation of localized p–n heterojunction thereby making the PANI/SnO2 nanocomposite electrically more insulating in nature. However, exposure to NH3 gas caused polarization of NH3 molecules by the depletion region, which provided PANI molecules with positive charge whose mobility along the PANI chain made the nanocomposite relatively more conducting. The formation of such p–n heterojunction between PANI and nano-metal oxide has been proposed by other research groups as well.59,115
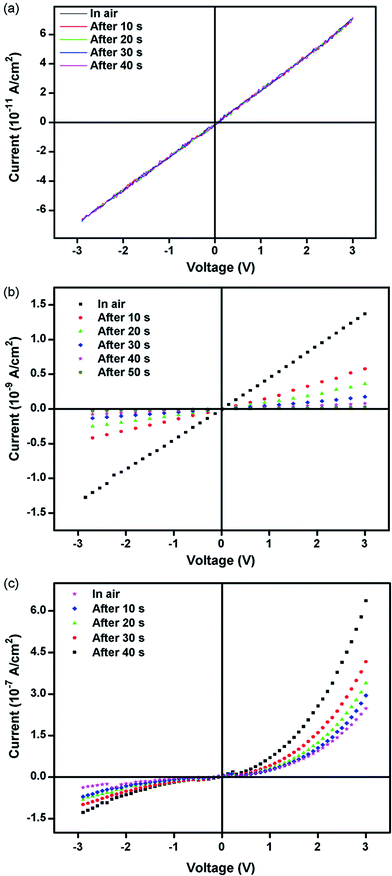 |
| Fig. 9 I–V curves (in the presence of ammonia gas) for (a) tin oxide, (b) polyaniline and (c) tin oxide/polyaniline nanocomposites (reprinted with permission114). | |
Zhang and coworkers116 reported PANI/PMMA nanocomposite for trace level NH3 detection for concentration as low as 1 ppm. The high sensitivity of the sensor was attributed to the highly aligned PMMA microfibers coated with PANI which facilitated faster diffusion of gas molecules, thereby accelerating electron transfer. Very recently, Zhihua and coworkers117 reported a porous thin film nanocomposite of PANI/sulphonated Ni phthalocyanine for NH3 sensing. They demonstrated that Ni phthalocyanine catalyzed electrodeposition of PANI films which in turn adversely affected the sensor's performance.
Detection of H2S gas using PANI nanocomposites has also been successfully attempted. Crowley and coworkers118 developed PANI/CuCl2 sensor printed on screen printed interdigitated electrodes for trace level H2S detection. A very different phenomenon was observed in their study, i.e., exposure to H2S gas resulted in protonation of PANI nanocomposite. The researchers proposed that PANI gets deprotonated by complexation with CuCl2. When exposed to H2S gas, preferential binding of CuCl2 with S2− ion resulted in evolution of HCl which protonated PANI increasing its electrical conductivity. A similar study based on PANI/CuCl2 printed on interdigitated electrodes for H2S detection was carried out by Sarfraz et al.119 as well. Raut and coworkers120 demonstrated the use of PANI–CdS nanocomposite film for room temperature detection of H2S gas wherein they achieved a maximum response of ∼48% for 100 ppm H2S concentration. They reported the sensing mechanism to be dominated by the modifications at the depletion region.
Shirsat et al.121 have proposed a mechanism involving the formation of AuS (eqn (1)) and subsequent protonation of PANI for H2S detection by PANI/Au nanocomposites.
They suggested that donation of electrons to the protons formed in the reaction (eqn (1)) led to a change in the resistance of the PANI/Au nanocomposite. Fig. 10 shows the response of PANI/Au nanocomposite and PANI as a function of time towards different concentrations of H2S gas. The nanocomposite exhibited excellent response to trace level H2S gas (∼0.1 ppb). The authors suggested that transfer of electrons from PANI to Au led to a drop in resistance of the material.
 |
| Fig. 10 (a) Response and recovery transients (solid line) of gold nanoparticles functionalized PANI nanowire network based chemiresistive sensor toward 0.1 ppb, 1 ppb, 10 ppb, 100 ppb, 500 ppb, and 1 ppm (dashed line) concentrations of H2S gas, (b) response of unfunctionalized PANI nanowire network toward 50 ppm of H2S gas ( indicates carrier gas and ↔ indicates gas analyte).121 Reproduced with permission from [M. D. Shirsat, M. A. Bangar, M. A. Deshusses, N. V. Myung, A. Mulchandani, Polyaniline nanowires–gold nanoparticles hybrid network based chemiresistive hydrogen sulfide sensor, Appl. Phys. Lett. 94 (2009) 083502-083504]. Copyright [2009], AIP Publishing LLC. | |
3.1.3. LPG sensor. LPG sensing by PANI nanocomposites is also a much investigated topic. An inflammable gas such as LPG demands detection in ambient conditions (i.e., at room temperature) for safety purposes. The use of electroactive PANI as a physical transducer for room temperature sensing provides a safer option as opposed to metal oxide based high temperature detectors. Joshi et al.122 reported the use of n-CdSe/p-PANI nanocomposite for LPG sensing wherein the response was a result of the sensor's modified depletion layer. In a similar vein, we reported the detection of LPG by PANI/γ-Fe2O3 nanocomposite at room temperature.54 Microscopic study revealed nanoscale morphology for the nanocomposites which afforded a high surface area for gas adsorption. I–V characteristic of the nanocomposite, as shown in Fig. 11, revealed the formation of a p–n heterojunction between PANI and γ-Fe2O3 nanoparticles. Based on our investigations, we proposed that the detection of LPG resulted from an increase in the depletion depth due to the adsorption of gas molecules at the depletion region of the p–n heterojunction. Moreover, the sensor's response was found to be influenced by the nanoparticle content.
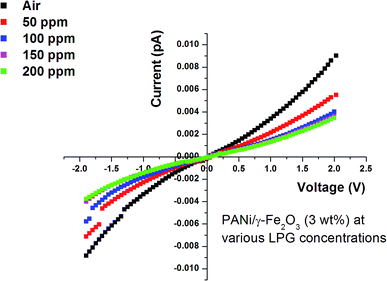 |
| Fig. 11 Current–voltage (I–V) curve of PANi/γ-Fe2O3 nanocomposite at 3 wt% γ-Fe2O3 content (F3) in air and at different LPG concentrations (Reprinted with permission54). | |
Dhawale and coworkers123–125 prepared a series of PANI based nanocomposite sensors for detection of LPG at room temperature. Their sensors exhibited significant selectivity towards LPG as compared to N2 and CO2. They too ascribed the sensor's response to a change in the barrier potential of the heterojunction.
3.1.4. Humidity sensor. Humidity (water vapour) has significant effect on electrical conductance of PANI. Its detection using PANI based sensors is another arena where extensive research has been conducted. Shukla et al.126 reported a PANI/ZnO nanocomposite based electrochemical humidity sensor. They suggested that adsorption of water molecules on the sensor surface causes efficient directional charge conduction at the heterojunction formed between PANI and ZnO. They found that charge conduction becomes more profound in case of PANI/ZnO nanocomposite as ZnO increased water adsorption capacity of PANI. Vijayan et al.127 have reported an optical fiber based PANI/Co humidity sensor which exhibited very low response and recovery times of 8 s and 1 min, respectively. In yet another case, PANI/Ag nanocomposite deposited on an optical fiber clad was used for humidity detection.128 A dramatic improvement in sensor response was observed with reduction in size of Ag nanoparticles. Such high responses towards humidity have been observed with other PANI nanocomposites as well.129,130 A fast responsive humidity sensor based on a hybrid nanocomposite structure of PANI with silver-vanadium oxide has been reported by Diggikar et al.131 Because PANI is significantly affected by humidity, detection of other analytes in the presence of humidity does not allow real time monitoring. In their recent work, Cavallo and coworkers132 have demonstrated that at high level of relative humidity (65–90%) swelling of polymer due to continuous absorption of water takes place, which increases its interchain distance thus hindering the charge hopping process and decreasing electrical conductance. It is therefore imperative that the influence of humidity on the electrical behaviour of PANI and its pathways be investigated.
3.1.5. Other gases. PANI has low sensitivity towards certain gasses, such as methane (CH4) and carbon monoxide (CO), as these do not show redox properties at room temperature. Such analytes may not undergo chemical reactions with polymers, but have weak physical interaction with the polymer matrix. The presence of metal/metal oxide nanoparticles in the PANI matrix can enhances its response towards these gases. Indium(III) oxide (In2O3) incorporated PANI nanocomposite exhibited good responses towards CH4 and CO.133 The response was higher for CH4 and was found to be temperature dependent. The theory of analyte detection via catalytic pathway has also been proposed by Ram et al.134 in their study of CO detection by PANI/SnO2 nanocomposite. PANI/SWCNT nanocomposite based sensor too demonstrated good response towards CO.135 As compared to NH3 gas which was also included in the study, the sensor showed propensity towards CO absorption. In most cases, detection of CO by PANI nanocomposite is explained using the particle electron transfer model – the stable resonance structure of +C
O− withdraws the lone pair of electron from the amine nitrogen in PANI thus creating a positive charge on it. The mobility of these positive charges generated on the amine nitrogen of PANI increases its conductivity.PANI/TiO2/MWCNT nanocomposite has been successfully used for NO detection through photocatalytic behaviour of TiO2.136 The authors reported that under UV irradiation the NO gas gets decomposed under the photocatalytic effect of TiO2 giving HNO2, NO2 and HNO3. These decomposition products get adsorbed onto the surface of PANI/MWCNT, owing to its high specific surface area and hydrophilicity of PANI. Such combination effects changes the electrical resistance of the nanocomposite, thus facilitating detection. Fig. 12 shows the sensitivities (S) of the nanocomposites towards NO under UV irradiation. PANI/TiO2/MWCNT nanocomposite (PCT) exhibited a high response towards NO, while PANI (PA) showed the least. PANI/MWCNT (PC) and PANI/TiO2 (PT) composites showed better response as compared to its individual components.
 |
| Fig. 12 NO gas sensing behaviour of various samples under UV irradiation (Reprinted with permission from136). | |
Several other gases such as NO2 and H2 were also detected by PANI nanocomposites.98,137,138 Introduction of CNT or metal oxides into PANI have shown response towards H2 gas. Srivastava et al.138 used interdigitated electrodes based on PANI/TiO2 and PANI/MWCNTs for H2 gas detection with both the materials eliciting a high response. Arsat et al.139 too reported PANI/MWCNTs deposited on lithium tantalite SAW transducers for H2 gas detection. Another SAW based PANI/WO3 sensor deposited on layered ZnO/64° YX LiNbO3 SAW transducer gave fast response with good repeatability.140 A PANI/PtO2 based thin film sensor has also been reported for H2 sensing.141 The authors reported that the PtO2 present in PANI was reduced to PtO during sensor conditioning stage. When the sensor was exposed to H2 gas, it catalytically oxidizes to water which decreases the resistance of PANI.
Azim-Araghi and Jafari142 developed an interdigitated electrode of PANI–chloroaluminium phthalocyanine (PANI/ClAlPc) for CO2 detection. They reported an optimum concentration of 10% for ClAlPc in PANI for maximum sensitivity at 300 K. They proposed the formation of a charge transfer complex between the aromatic units in PANI and phthalocyanine as donors with CO2 acceptor molecules.
The detection of a single analyte can take place through several different pathways – from nanometal catalyzed reaction to modification of barrier height. The response elicited from a nanocomposite sensor on exposure to an analyte is governed by two factors: (i) charge transfer phenomenon between the constituents of the nanocomposite, and (ii) reaction between the analyte and the nanocomposite. For example, as previously discussed, H2S can either have a reducing or an oxidizing effect on PANI depending on the interaction of PANI with the secondary component in the nanocomposite. Moreover, taking into account the humidity factor is necessary as it is known to significantly decrease the sensitivity of PANI sensors. Therefore, for future PANI based sensing materials, a deeper understanding of the sensing behaviour is required to develop high performing sensors.
3.2. PANI nanocomposites as biosensors
Updike and Hicks143 reported the first biosensor system which functioned through immobilized sensor activity. This was the beginning of modern biosensors that are widely in use today. Their system comprised of an enzyme (glucose oxidase) immobilized on a gel which measured the concentration of glucose in biological solutions. A variety of biosensors has come up since, finding applications in industry, clinical diagnostics and environment monitoring.144
A biosensor typically consists of a biorecognition element (or bioreceptor), a transducer element, and electronic components for signal processing. The schematic of a biosensor operation is shown in Fig. 13. It operates in three stages – (i) recognition of a specific analyte by the bioreceptor, (ii) transformation of biochemical reaction into transducer-type reaction, and (iii) processing of transducer signal.144
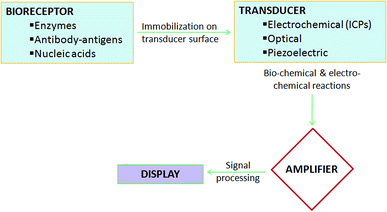 |
| Fig. 13 Schematic representation of biosensor operation. | |
PANI is particularly attractive as biosensors as it provides a conducting matrix for immobilization of bioreceptors (i.e., confined movement of bioreceptors in a defined space) onto it. Its electroactivity allows it to act as a mediator for electron transfer through a redox or enzymatic reaction. Such direct communication with the bound bioreceptors leads to a range of analytical signals which gives a measurement of the sensor activity.145 Apart from its electronic properties, PANI has shown excellent stability and strong biomolecular interactions146,147 necessary for biosensor applications. Properties of PANI such as electrical conductivity, electrochromism and pH sensitivity have been successfully employed for detection of different biological compounds.148–150 Nanocomposites of PANI provide a scope to further assess the potential of these materials as biosensors. A secondary component in the PANI matrix is often seen to increase bioreceptor binding onto PANI surface. Based on the bioreceptors, biosensors are broadly classified as enzymatic biosensors, immunosensors, and DNA/nucleic acid biosensors, which are discussed in the following sections.
3.2.1. Enzymatic biosensors. The concept of enzymatic biosensors was introduced by Clark and Lyons151 using the enzyme glucose oxidase (GOx). They developed an amperometric biosensor in which the enzyme catalyzed the oxidation of glucose on the surface of Pt electrode. Owing to the enzymatic reaction the oxygen flux to the electrode surface varied as a function of glucose concentration, thus enabling its detection. However, the enzyme activity took place in solution as opposed to the recent biosensors which have immobilized enzymes.
3.2.1.1. Glucose biosensor. Since it was first introduced, glucose biosensors have undergone significant modifications as regards to its detection technique and development of newer materials for enzyme immobilization. Xian et al.152 have reported a glucose biosensor based on Au/PANI nanocomposite. GOx and Nafion were immobilized on the nanocomposite surface, and quantification of glucose was done by electrochemical detection (oxidation) of enzymatically released H2O2. A much higher anodic current was observed by the Au/PANI nanocomposite as compared to PANI, indicating a better response, possibly as a result of electron transfer between electrode and H2O2 facilitated by Au in PANI matrix. Xu et al.153 prepared a graphene/PANI/Au nanocomposite modified GCE† as glucose biosensor. This nanocomposite was found to be more biocompatible and facilitated efficient electron transfer between GOx and the electrode.Nanocomposites of PANI/MWCNTs have also been employed as biosensors for glucose detection.154–156 The biosensor developed by Le et al.156 had PANI/MWCNTs deposited on interdigitated planar Pt-film electrode over which the GOx was immobilized via glutaraldehyde. Fig. 14 presents the graph showing rapid amperometric response of PANI/MWCNTs to changing glucose concentration. The porous nature of the nanocomposite facilitated stronger binding to GOx, and the nanocomposite proved to be an efficient transducer with a response time of 5 s. More recently, Zhu and coworkers157 reported a GOx immobilized PANI–TiO2 nanotube composite as electrochemical biosensor. The performance studies revealed that the nanoscaled tub-like morphology facilitated direct electron transfer of GOx, giving a sensitivity of 11.4 μA mM−1 at a low detection range of 0.5 μM at a high signal-to-noise ratio.
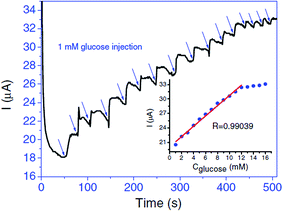 |
| Fig. 14 Amperometric response of GOx/PANI-MWCNT/IDμE upon increasing the glucose concentration in steps of 1 mM at +0.6 V (versus SCE) in PBS. Inset shows the calibration plot156 (Reproduced with permission from IOPscience). | |
Though most biosensors utilize immobilized GOx for glucose detection, other enzymes can also be used for this purpose. Ozdemir et al.158 have reported a biosensor based on pyranose oxidase immobilized Au/PANI/AgCl/gelatin nanocomposite for glucose detection, wherein sensing was facilitated by amperometric detection of consumed O2 during the enzymatic reaction. The importance of glucose biosensors lies in simplistic and accurate monitoring of blood glucose levels, which might help in controlling the growing issue of diabetes around the world.
3.2.1.2. Cholesterol biosensor. The rise in heart diseases and other illness related to high cholesterol levels in blood has prompted researchers to develop suitable devices for efficient and accurate monitoring blood cholesterol levels. Towards this end, a number of publications cite PANI nanocomposites as a material conducive to cholesterol detection. For instance, Srivastava et al.159 reported a cholesterol oxidase (ChOx) immobilized PANI/Au/chitosan nanocomposite for cholesterol detection. The enzyme-substrate kinetics for the nanocomposite biosensor showed that it facilitates enzymatic reaction and activity. PANI nanocomposites with CNTs and/or graphene are also extensively used for cholesterol detection. A ChOx immobilized graphene/PVP/PANI modified paper-based electrode has shown a low detection limit of 1 μM‡.160 The presence of PVP was reported to enhance the sensitivity of the biosensor.It has often been observed that these biosensors show a high shelf life when stored at low temperature. PANI/Ag nanocomposite too was employed as cholesterol sensing.161 The biosensor gave a fast response towards cholesterol and showed uniform activity for up to 50 days when stored at low temperatures. Dhand et al.162 have described a PANI/MWCNT coated ITO electrode over which ChOx was immobilized via N-ethyl-N′-(3-dimethylaminopropyl)carbodiimide and N-hydroxysuccinimide. Storage at 4 °C gave this biosensor a shelf life of about 12 weeks. Similarly, a PANI/carboxymethyl cellulose (CMC) nanocomposite deposited on ITO coated glass using glutaraldehyde as cross-linker showed a shelf life of 10 weeks when stored at 4 °C.163
Saini et al.164 reported a bienzymatic cholesterol biosensor based on PANI/Au/graphene nanocomposite. Both ChOx and horseradish peroxidase (HRP) were immobilized on the surface of the nanocomposite. Studies reveal that this bienzyme immobilized bioelectrode facilitates efficient electron transfer between the bienzyme and the electrode, thereby exhibiting better performance over monoenzymatic biosensor.
3.2.1.3. Peroxide biosensors. Hydrogen peroxide (H2O2) is a byproduct of a wide range of biological processes, and plays an important role in various cellular activities of mammalian cells.165–167 H2O2 has also been found to be toxic to cells – together with the superoxide anion and the hydroxyl radical it forms the reactive oxygen species in the body, which is capable of causing macromolecular damage to cells.168 Due to its varying cellular functions, detection and quantification of H2O2 is necessary to understand the underlying mechanism in its production and function. Generally, horseradish peroxidase (HRP) is employed for peroxide detection. HRP immobilized on PANI/polyester sulphonic acid (PESA) nanocomposite has been reported to detect H2O2 with a detection limit of 0.185 μM.169 A PANI/chitosan nanocomposite film with HRP immobilized on its surface was used by Du et al.170 for H2O2 detection. While the enzyme catalyzed the reduction of H2O2, the nanocomposite enabled rapid electron transfer between the active centers of the enzyme and electrode.
3.2.1.4. Other enzymatic biosensors. Several other enzymes have been immobilized on PANI nanocomposites enabling detection of an array of compounds. One of these is uricase which is used for sensing uric acid. Devi and Pundir171 reported a covalently immobilized uricase on Fe3O4/chitosan/PANI nanocomposite electrode. The hybrid composition of the electrode facilitated charge transfer resulting in a appreciable sensitivity of 0.44 mA mM−1 at a detection limit of 0.1 μM in under 1 s. A nanocomposite of Prussian blue nanoparticles, carboxylated MWCNTs (cMWCNTs) and PANI has also exhibited good sensitivity towards uric acid.172 The enzyme uricase was immobilized onto the nanocomposite surface via chitosan–glutaraldehyde cross-linking. Similarly, creatinine can also be detected using enzyme immobilized PANI nanocomposites. PANI/cMWCNTs with enzymes creatinine amidohydrolase, creatine amidinohydrolase, and sarcosine oxidase immobilized onto it via N-ethyl-N′-(3-dimethylaminopropyl)carbodiimide and N-hydroxy succinimide has shown promising results.173 This tri-enzyme system was also utilized by Yadav et al.174 for creatinine detection wherein the enzymes were immobilized on Fe3O4/chitosan/PANI nanocomposite electrode which gave a fast response with a detection limit of 1 μM.Very recently, Zhybak and coworkers175 demonstrated a creatinine deaminase and urease immobilized ammonium ion-specific Cu/PANI nanocomposite for creatinine and urea detection. The biosensors were characterized by high selectivity, sensitivity and fast response. Fig. 15 shows the amperometric response of creatinine and urea biosensors. The addition of glucose prevented loss of enzyme activity providing better homogeneity to the biosensor membrane. The creatinine and urea sensors exhibited excellent sensitivity of ∼95 and 91 mA M−1 cm−2.
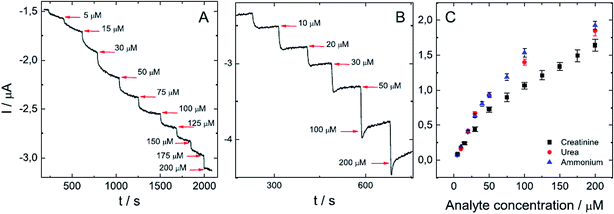 |
| Fig. 15 (A) An example of the amperometric response of the creatinine biosensor to successive additions of creatinine (−0.35 V, PBS pH 7.4). (B) An example of the amperometric response of the urea biosensor to successive additions of urea (−0.35 V, PBS pH 7.4). (C) Calibration curve for creatinine, urea and ammonium ion detection obtained at creatinine and urea biosensors and PANI–Nafion–Cu-modified SPE (Reprinted with permission175). | |
3.2.2. Immunosensors. The combination of antigen–antibody specificity and transducer forms the basis of an immunosensor. Huang et al.176 described a multicomponent system for detection of salbutamol. They prepared a nanocomposite of PANI with Au nanoparticles, Prussian blue, poly(acrylic acid) and Au–graphene. A label of chitosan coated graphene with nano-Au shell was attached to immobilize HRP-anti-SAL antibody on this multicomponent nanocomposite. The immunosensor showed good catalytic activity for hydrogen reduction on the electrode. Liu and coworkers177 prepared GO/PANI/CdSe nanocomposite for detection of interleukin-6. The electrochemiluminescence of CdSe was greatly improved by combining with graphene oxide/PANI. The nanocomposite showed high specificity, long term stability and reproducibility with detection limit as low as 0.17 pg mL−1.§PANI/Au nanocomposite has been used for detection of prostate-specific antigen (PSA).178 The sensor performance was measured using differential pulse voltammetry (DPV) which studied the electrochemical changes resulting from the biochemical reactions on the surface. The nanocomposite provided a high surface area for immobilization of anti-PSA, and resulted in increased electron transfer which improved the performance of the immunosensor. Fig. 16 shows the electrochemical response studies of the immunosensor to different concentrations of PSA. The sensor exhibited good linearity, high sensitivity and excellent response at very low concentrations. The immunosensor showed stability for up to 5 weeks after which a decrease in response current was observed.
 |
| Fig. 16 (A) Electrochemical response studies of the BSA/anti-PSA/AuNP-PSA/Au immunoelectrode as a function of PSA (1 pg mL−1 to 100 ng mL−1) in PBS (10 mM, pH 7 containing 0.9% NaCl) using DPV technique. Inset (a) calibration curve between magnitude of electrochemical response current vs. logarithm of PSA concentration and inset (b) shelf-life studies of BSA/anti-PSA/AuNP-PSA/Au immunoelectrode. (B) Interference studies of BSA/anti-PSA/AuNP-PSA/Au immunoelectrode using BSA (10 ng mL−1) and cortisol (10 ng mL−1) with respect to PSA (10 ng mL−1). Reproduced from178 with permission from The Royal Society of Chemistry. | |
A multilayer nanocomposite of Au/PANI/cMWCNTs/chitosan has been used for detection of chlorpyrifos, an organophosphate insecticide.179 The Au nanoparticle on the surface of the nanocomposite provided an excellent platform for immobilization of anti-chlorpyrifos antibody. The immunosensor showed superior sensitivity and specific immunoreactions even when used for analysis of real samples. Like other biosensors, storage at low temperatures affords the sensor a longer shelf life. Detection of estradiol by graphene/PANI nanocomposite based immunosensor has been reported by Li et al.180 Carboxylated graphene oxide was used as the carrier for HRP-antibody immobilization on the sensor surface, which improved the catalytic activity of the hydrogen reduction of electrode. The immunosensor showed a wide range of linearity with a low detection limit of 0.02 ng mL−1.¶ Lin et al.181 reported the detection of benzo[a]pyrene (BaP) using a multi-enzyme antibody of HRP-HCS-secondary antibody immobilized on Fe3O4/PANI/Nafion sensor. The nanocomposite provided an efficient electron transfer pathway for reduction of H2O2.
A label-free immunosensor has been developed by Yan et al.182 for detection of low density lipoproteins (LDL). Apolipoprotein B-100 antibody was immobilized on the surface of Au–AgCl/PANI nanocomposite, and the sensor performance was measured by electrochemical impedance spectroscopy (EIS). Label-free biosensors enable monitoring of real time biochemical reactions while providing a scope for exploring modulation factors. The poor conductivity of LDL and the negative charges carried by it brings about a change in electron transfer resistance which enabled its detection. The immunosensor gave a very low detection limit of 0.34 pg mL−1 at an optimized incubation time of 50 min and incubation temperature of 37 °C. PANI based hybrid materials provide an efficient transducer to which the immunochemical reactions can be coupled. The ease of fabrication and tunability of PANI properties by combination of nanomaterials allows facile label-free detection of antigens.
3.2.3. DNA biosensors. Biosensors aimed at DNA detection are so fabricated as to provide high sensitivity and accurate and rapid detection. The development of DNA biosensors stems from its applicability in the fields of forensics, gene analysis, biological warfare detection and DNA diagnostics.145 A single-stranded DNA (ssDNA) probe immobilized on the transducer surface recognizes its complementary DNA target by hybridization. Wu and coworkers183 reported a PANI/graphite oxide nanocomposite over carbon paste electrode (CPE) for monitoring DNA hybridization. Their results indicated that both ssDNA and dsDNA (double-strand DNA) change the redox characteristics of the nanocomposite electrode. Square wave voltammetry (SWV), used to monitor hybridization and detect the complementary ssDNA, revealed good stability and reproducibility for the sensor, and showed that its responses were influenced by factors like pH and incubation time. Other researchers184,185 too used nanocomposites of graphene and PANI for DNA sensing. Wang and coworkers186 designed a layered biosensor by fabricating graphene sheets on GCE followed by electropolymerization of PANI and electro-deposition of Au nanoparticles for detection of BCR/ABL fusion gene in chronic myelogenous leukemia (CML). The ssDNA probe was dually labeled at 5′ and 3′ with –SH and biotin, respectively. Following hybridization, the 3′-biotin site face moved away from the electrode surface, binding the streptavidin–alkaline phosphatase. The hydrolysis of 1-naphthyl phosphate to 1-naphthol was monitored via DPV. The biosensor showed high selectivity with a detection limit of 2.11 pM.||A label-free electrochemical DNA sensor based on PPY/PANI/Au nanocomposite used ssDNA labeled with 6-mercapto-1-hexane for hybridization.187 The biopolymer enhanced the hybridization efficiency of the ssDNA as compared to the lone Au nanoparticle based transducer. The biosensor was highly sensitive towards the complementary ssDNA with a low detection limit of 10−13 M. Forster and coworkers188,189 employed a PANI nanocomposite with surface deposited Au nanoparticles as DNA biosensor. In one of their reports,188 thiolated ssDNA, which is complementary to a sequence of Staphylococcus aureus, was immobilized onto the nanocomposite surface. HRP labeled probe strand was hybridized to an unbound section of the capture strand. Target concentration was quantified by current measurements during reduction of benzoquinone through HRP.
Yang et al.190 reported a PANI/MWCNT nanocomposite for detection of phosphinothricin acetyltransferase gene (PAT), which is one of the screening detection genes for the transgenic plants. An ssDNA probe was immobilized onto the nanocomposite and hybridization was detected through EIS. The authors conducted the test on a real sample of genetically modified soybean. PAT has also been detected using PANI/ZrO2 nanocomposites with polytyrosine.191 The nanocomposite aided in ssDNA immobilization and hybridization, which were studied by CV and EIS. The electron transfer resistance was found to increase with concentration of target DNA. It should however be noted that both these nanocomposites, i.e., PANI/MWCNT and PANI/ZrO2, exhibited the same detection limit. Another study reports the detection of DNA base (guanine, adenine, thymine, and cytosine) using PANI/MnO2 nanocomposite.192 Radhakrishnan et al.193 described a PPY/PANI nanocomposite with ssDNA immobilized onto it via glutaraldehyde, and using methyl blue as electrochemical indicator. The biosensor exhibited a high sensitivity with a detection limit of 50 fM.** DNA biosensors have also been employed for pesticide detection. For example, a nucleic acid based biosensor for pesticide detection has been reported by Prabhakar et al.194 The calf thymus dsDNA immobilized on PANI/polyvinyl sulphonate (PVS) nanocomposite exhibited high stability for up to 6 months. In DNA biosensors, the immobilization of nucleic acid probe sequence onto a transducer while maintaining its activity is crucial to the device's performance. Hybridization with the target DNA changes the doping level of PANI, and therefore its conductivity.
4. Conclusion and future challenges
PANI nanocomposites has shown much promise as a sensing element, as reflected by its multitude of applications. However, their commercialization still remains a challenge for scientists across the world due to the fact that PANI itself suffers from a number of drawbacks. First of them is regarding the synthesis of PANI. To avoid secondary growth and retain its nanostructure, controlled synthesis of PANI at optimized pH and temperature is necessary. Dopant also plays a very important role in formation of PANI, as well as its electronic properties. Depending on the type of dopant, PANI can be highly conducting, self-doped, and/or soluble in water or different organic solvents. PANI in doped form can have a relatively short shelf life. A secondary component in PANI can have a synergistic effect in improving its shelf life in doped form, and various other properties, and thus applicability. Hence, fabrication of PANI nanocomposites with an extended life in doped form is essential for its long service life.
Nanocomposites of PANI show better properties as compared to neat PANI. However, in many cases the secondary component also tends to reduce its electrical conductivity. Nevertheless, PANI nanocomposites offer safer detection of number combustible and toxic gases at room temperature by inducing selectivity and higher sensitivity into the material. Room temperature sensing not only prevents response variation resulting from structural changes in the sensing material at high temperatures, but also avoids sensor instability thus extending its shelf life. However, the sensor response of a large number of these nanocomposites is influenced by humidity which may cause false responses in the material. Hence, developing PANI nanocomposites impervious to humidity is one of the great challenges. An increased electron transfer capability of PANI nanocomposites is also desirable for detection of biological agents. Immobilization of biological agents onto PANI transducers becomes more feasible when a secondary component has been combined with PANI. A better interaction between the components of the nanocomposite will enhance the efficiency and performance of the sensor manifold.
Finally, new strategies need to be devised in order to fully understand the underlying mechanism involved in analyte sensing by PANI and its nanocomposites, which will enable us in fabrication and commercialization of highly selective sensors for specific agents.
Acknowledgements
One of the authors (T. Sen) is thankful to the University Grants Commission, New Delhi, India for providing financial support under the Non-SAP RFSMS scheme.
References
- M. Wan, Conducting polymers with micro or nanometer structure, Tsinghua University Press, Beijing and Springer-Verlag GmbH, Berlin, Heidelberg, 2008, p. 3 Search PubMed.
- G. G. Wallace, G. M. Spinks, L. A. P. Kane-Maguire and P. R. Teasdale, Conductive electroactive polymers, Taylor & Francis Group, LLC, Florida, 2009, p. 137 Search PubMed.
- N. Gospodinova and L. Terlemezyan, Conducting polymers prepared by oxidative polymerization: polyaniline, Prog. Polym. Sci., 1998, 23, 1443–1484 CrossRef CAS.
- X. Lua, W. Zhanga, C. Wanga, T.-C. Wenb and Y. Wei, One-dimensional conducting polymer nanocomposites: synthesis, properties and applications, Prog. Polym. Sci., 2011, 36, 671–712 CrossRef.
- E. T. Kang, K. G. Neoha and K. L. Tan, Polyaniline: A Polymer with Many Interesting Intrinsic Redox States, Prog. Polym. Sci., 1998, 23, 277–324 CrossRef CAS.
- Y.-Z. Longa, M.-M. Li, C. Gub, M. Wan, J.-L. Duvaild, Z. Liue and Z. Fan, Recent advances in synthesis, physical properties and applications of conducting polymer nanotubes and nanofibers, Prog. Polym. Sci., 2011, 36, 1415–1442 CrossRef.
- A. Rahy and D. J. Yang, Synthesis of highly conductive polyaniline nanofibers, Mater. Lett., 2008, 62, 4311–4314 CrossRef CAS.
- Z. J. Gu, J. R. Ye, W. Song and Q. Shen, Synthesis of polyaniline nanotubes with controlled rectangular or square pore shape, Mater. Lett., 2014, 121, 12–14 CrossRef CAS.
- M. Ayad, G. El-Hefnawy and S. Zaghlol, Facile synthesis of polyaniline nanoparticles; its adsorption behavior, Chem. Eng. J., 2013, 217, 460–465 CrossRef CAS.
- Y. F. Kuang, C. P. Fu and H. H. Zhu, Polyaniline Nanocomposites, in Polymer Nanocomposite Research Advances, ed. S. Thomas and G. E. Zaikov, Nova Science, New York, 2008, p. 314 Search PubMed.
- J. Jang, J. Ha and K. Kim, Organic light-emitting diode with polyaniline-poly(styrene sulfonate) as a hole injection layer, Thin Solid Films, 2008, 516, 3152–3156 CrossRef CAS.
- Z. Zhou, X. Zhang, C. Lu, L. Lan and G. Yuan, Polyaniline-decorated cellulose aerogel nanocomposite with strong interfacial adhesion and enhanced photocatalytic activity, RSC Adv., 2014, 4, 8966–8972 RSC.
- A. A. Khan and L. Paquiza, Characterization and ion-exchange behavior of thermally stable nano-composite polyaniline zirconium titanium phosphate: its analytical application in separation of toxic metals, Desalination, 2011, 265, 242–254 CrossRef CAS.
- P. Bober, J. Stejskal, M. Trchova and J. Prokes, In situ prepared polyaniline–silver composites: single- and two-step strategies, Electrochim. Acta, 2014, 122, 259–266 CrossRef CAS.
- L. Li, G. Yan, J. Wu, X. Yu and Q. Guo, Preparation of polyaniline–metal composite nanospheres by in situ microemulsion polymerization, J. Colloid Interface Sci., 2008, 326, 72–75 CrossRef CAS PubMed.
- P. Boomi and H. G. Prabu, Synthesis, characterization and antibacterial analysis of polyaniline/Au–Pd nanocomposite, Colloids Surf., A, 2013, 429, 51–59 CrossRef CAS.
- P. Boomi, H. G. Prabu and J. Mathiyarasu, Synthesis and characterization of polyaniline/Ag–Pt nanocomposite for improved antibacterial activity, Colloids Surf., B, 2013, 103, 9–14 CrossRef CAS PubMed.
- H. Guleryuz, C. Filiatre, M. Euvrard, C. Buron and B. Lakard, Novel strategy to prepare polyaniline-modified SiO2/TiO2 composite particles, Synth. Met., 2013, 181, 104–109 CrossRef CAS.
- Q. Xiao, X. Tan, L. Ji and J. Xue, Preparation and characterization of polyaniline/nano-Fe3O4 composites via a novel pickering emulsion route, Synth. Met., 2007, 157, 784–791 CrossRef CAS.
- F. Huang, E. Vanhaecke and D. Chen, In situ polymerization and characterizations of polyaniline on MWCNT powders and aligned MWCNT films, Catal. Today, 2010, 150, 71–76 CrossRef CAS.
- O.-K. Park, T. Jeevananda, N. H. Kim, S. Il Kim and J. H. Lee, Effects of surface modification on the dispersion and electrical conductivity of carbon nanotube/polyaniline composites, Scr. Mater., 2009, 60, 551–554 CrossRef CAS.
- Y.-C. Lin, F.-H. Hsu and T.-M. Wu, Enhanced conductivity and thermal stability of conductive polyaniline/graphene composite synthesized by in situ chemical oxidation polymerization with sodium dodecyl sulfate, Synth. Met., 2013, 184, 29–34 CrossRef CAS.
- Q. Zhang, Y. Li, Y. Feng and W. Feng, Electropolymerization of graphene oxide/polyaniline composite for high-performance supercapacitor, Electrochim. Acta, 2013, 90, 95–100 CrossRef CAS.
- S. V. Bhat and S. R. C. Vivekchand, Optical spectroscopic studies of composites of conducting PANI with CdSe and ZnO nanocrystals, Chem. Phys. Lett., 2006, 433, 154–158 CrossRef CAS.
- S. Zhang, Q. Chen, D. Jing, Y. Wang and L. Guo, Visible photoactivity and antiphotocorrosion performance of PdS–CdS photocatalysts modified by polyaniline, Int. J. Hydrogen Energy, 2012, 37, 791–796 CrossRef CAS.
- B. H. F. Moura, R. H. B. Assis, P. I. B. M. Franco, N. R. Antoniosi Filho and D. Rabelo, Synthesis and characterization of composites based on polyaniline and styrene-divinylbenzene copolymer using benzoyl peroxide as oxidant agent, React. Funct. Polym., 2013, 73, 1255–1261 CrossRef CAS.
- Z. Ben Othmen, A. Fattoum and M. Arous, Dielectric study of polyaniline/poly(methylmethacrylate) composite films below the percolation threshold, J. Electrost., 2013, 71, 999–1004 CrossRef CAS.
- D. N. Huyen, Carbon Nanotubes and Semiconducting Polymer Nanocomposites, in Carbon Nanotubes – Synthesis, Characterization, Applications, ed. S. Yellampalli, In Tech, Croatia, 2011, pp. 469–486 Search PubMed.
- K. Gupta, P. C. Jana and A. K. Meikap, Optical and Electrical Transport Properties of Polyaniline–Silver Nano-composite, Synth. Met., 2010, 160, 1566–1573 CrossRef CAS.
- Z.-A. Hu, Y.-L. Xie, Y.-X. Wang, L.-P. Mo, Y.-Y. Yang and Z.-Y. Zhang, Polyaniline/SnO2 nanocomposite for supercapacitor applications, Mater. Chem. Phys., 2009, 114, 990–995 CrossRef CAS.
- A. Olad and R. Nosrati, Preparation, characterization, and photocatalytic activity of polyaniline/ZnO nanocomposite, Res. Chem. Intermed., 2012, 38, 323–336 CrossRef CAS.
- P. Kannusamy and T. Sivalingam, Chitosan–ZnO/polyaniline hybrid composites: polymerization of aniline with chitosan–ZnO for better thermal and electrical property, Polym. Degrad. Stab., 2013, 98, 988–996 CrossRef CAS.
- G. Qiu, Q. Wang and M. Nie, Polyaniline/Fe3O4 magnetic nanocomposite prepared by ultrasonic irradiation, J. Appl. Polym. Sci., 2006, 102, 2107–2111 CrossRef CAS.
- M. D. Bedre, S. Basavaraja, B. D. Salwe, V. Shivakumar, L. Arunkumar and A. Venkataraman, Preparation and characterization of PANI and PANI–Ag nanocomposites via interfacial polymerization, Polym. Compos., 2009, 30, 1668–1677 CrossRef CAS.
- B. Zhang, B. Zhao, S. Huang, R. Zhang, P. Xu and H.-L. Wang, One-pot interfacial synthesis of Au nanoparticles and Au–polyaniline nanocomposites for catalytic applications, CrystEngComm, 2012, 14, 1542 RSC.
- F.-J. Liu, L.-M. Huang, T.-C. Wen, A. Gopalan and J.-S. Hung, Interfacial synthesis of platinum loaded polyaniline nanowires in poly(styrenesulfonic acid), Mater. Lett., 2007, 61, 4400–4405 CrossRef CAS.
- V. Divya and M. V. Sangaranarayanan, A facile synthetic strategy for mesoporous crystalline copper–polyaniline nanocomposites, Eur. Polym. J., 2012, 48, 560–568 CrossRef CAS.
- W. Cho, S.-J. Park and S. Kim, Effect of monomer concentration on interfacial synthesis of platinum loaded polyaniline nanocomplex using poly(styrenesulfonic acid), Synth. Met., 2011, 161, 2446–2450 CrossRef CAS.
- J. M. Kinyanjui, N. R. Wijeratne, J. Hanks and D. W. Hatchett, Chemical and electrochemical synthesis of polyaniline/platinum composites, Electrochim. Acta, 2006, 51, 2825–2835 CrossRef CAS.
- S. Jing, S. Xing, L. Yu, Y. Wu and C. Zhao, Synthesis and Characterization of Ag/polyaniline core-shell nanocomposites based on silver nanoparticles colloids, Mater. Lett., 2007, 61, 2794–2797 CrossRef CAS.
- S. S. Barkade, J. B. Naik and S. H. Sonawane, Ultrasound assisted miniemulsion synthesis of polyaniline/Ag nanocomposite and its application for ethanol vapor sensing, Colloids Surf., A, 2011, 378, 94–98 CrossRef CAS.
- S. Mishra, N. G. Shimpi and T. Sen, The effect of PEG encapsulated silver nanoparticles on the thermal and electrical property of sonochemically synthesized polyaniline/silver nanocomposite, J. Polym. Res., 2013, 20, 49–58 CrossRef.
- A. Houdayer, R. Schneider, D. Billaud, J. Ghanbaja and J. Lambert, New polyaniline/Ni(0) nanocomposites: synthesis, characterization and evaluation of their catalytic activity in Heck couplings, Synth. Met., 2005, 151, 165–174 CrossRef CAS.
- M. G. Hosseini, S. Zeynali, M. M. Momeni and R. Najjar, Polyaniline nanofibers supported on titanium as templates for immobilization of Pd nanoparticles: a new electro-catalyst for hydrazine oxidation, J. Appl. Polym. Sci., 2012, 124, 4671–4677 CAS.
- L. Kong, X. Lu, E. Jin, S. Jiang, C. Wang and W. Zhang, Templated synthesis of polyaniline nanotubes with Pd nanoparticles attached onto their inner walls and its catalytic activity on the reduction of p-nitroanilinum, Compos. Sci. Technol., 2009, 69, 561–566 CrossRef CAS.
- K. Basavaiah, Y. Pavan Kumar and A. V. Prasada Rao, A facile one-pot synthesis of polyaniline/magnetite nanocomposites by micelles-assisted method, Appl. Nanosci., 2013, 3, 409–415 CrossRef CAS.
- S. Zhu, W. Wei, X. Chen, M. Jiang and Z. Zhou, Hybrid structure of polyaniline/ZnO nanograss and its application in dye-sensitized solar cell with performance improvement, J. Solid State Chem., 2012, 190, 174–179 CrossRef CAS.
- B. Wang, C. Liu, Y. Yin, S. Yu, K. Chen, P. Liu and B. Liang, Double template assisting synthesized core–shell structured titania/polyaniline nanocomposite and its smart electrorheological response, Compos. Sci. Technol., 2013, 86, 89–100 CrossRef CAS.
- Z. Wang, H. Bi, J. Liu, T. Sun and X. Wu, Magnetic and microwave absorbing properties of polyaniline/γ-Fe2O3 nanocomposite, J. Magn. Magn. Mater., 2008, 320, 2132–2139 CrossRef CAS.
- S. Sathiyanarayanan, S. S. Azim and G. Venkatachari, Preparation of polyaniline–Fe2O3 composite and its anticorrosion performance, Synth. Met., 2007, 157, 751–757 CrossRef CAS.
- A. Mostafaei and A. Zolriasatein, Synthesis and characterization of conducting polyaniline nanocomposites containing ZnO nanorods, Prog. Nat. Sci., 2012, 22, 273–280 CrossRef.
- B. I. Nandapure, S. B. Kondawar, M. Y. Salunkhe and A. I. Nandapure, Magnetic and transport properties of conducting polyaniline/nickel oxide nanocomposites, Adv. Mater. Lett., 2013, 4, 134–140 CAS.
- M. D. Bedre, R. Deshpande, B. Salimath and V. Abbaraju, Preparation and Characterization of Polyaniline-Co3O4 Nanocomposites via Interfacial Polymerization, Am. J. Mater. Sci., 2012, 2, 39–43 CrossRef.
- T. Sen, N. G. Shimpi, S. Mishra and R. P. Sharma, Polyaniline/γ-Fe2O3 nanocomposite for room temperature LPG sensing, Sens. Actuators, B, 2014, 190, 120–126 CrossRef CAS.
- G. Li, C. Zhang, H. Peng and K. Chen, One-dimensional V2O5@polyaniline core/shell nanobelts synthesized by an in situ polymerization method, Macromol. Rapid Commun., 2009, 30, 1841–1845 CrossRef CAS PubMed.
- S. Pang, G. Li and Z. Zhang, Synthesis of Polyaniline–Vanadium Oxide Nanocomposite Nanosheets, Macromol. Rapid Commun., 2005, 26, 1262–1265 CrossRef CAS.
- C. R. K. Rao and M. Vijayan, Ruthenium(II)-mediated synthesis of conducting polyaniline (PANI): a novel route for PANI–RuO2 composite, Synth. Met., 2008, 158, 516–519 CrossRef CAS.
- S. L. Patil, S. G. Pawar, M. A. Chougule, B. T. Raut, P. R. Godse, S. Sen and V. B. Patil, Structural, Morphological, Optical, and Electrical Properties of PANI-ZnO Nanocomposites, Int. J. Polym. Mater. Polym. Biomater., 2012, 61, 809–820 CrossRef CAS.
- S. L. Patil, M. A. Chougule, S. G. Pawar, S. Sen, A. V. Moholkar, J. H. Kim and V. B. Patil, Fabrication of Polyaniline–ZnO Nanocomposite Gas Sensor, Sens. Transducers J., 2011, 134, 120–131 CAS.
- X. Zhang, J. Zhang, R. Wang and Z. Liu, Cationic surfactant directed polyaniline/CNT nanocables: synthesis, characterization, and enhanced electrical properties, Carbon, 2004, 42, 1455–1461 CrossRef CAS.
- A. Vega-Rios, F. Y. Renteria-Baltierrez, C. A. Hernandez-Escobar and E. A. Zaragoza-Contreras, A new route toward graphene nanosheet/polyaniline composites using a reactive surfactant as polyaniline precursor, Synth. Met., 2013, 184, 52–60 CrossRef CAS.
- S. Srivastava, S. S. Sharma, S. Agrawal, S. Kumar, M. Singh and Y. K. Vijay, Study of chemiresistor type CNT doped polyaniline gas sensor, Synth. Met., 2010, 160, 529–534 CrossRef CAS.
- C. Oueiny, S. Berlioz and F.-X. Perrin, Carbon nanotube–polyaniline composites, Prog. Polym. Sci., 2014, 39, 707–748 CrossRef CAS.
- F. Huang, E. Vanhaecke and D. Chen, In situ polymerization and characterizations of polyaniline on MWCNT powders and aligned MWCNT films, Catal. Today, 2010, 150, 71–76 CrossRef CAS.
- Q. Jiang, G. Fu, D. Xie, S. Jiang, Z. Chen, B. Huang and Y. Zhao, Preparation of carbon nanotube/polyaniline nanofiber by electrospinning, Procedia Eng., 2012, 27, 72–76 CrossRef CAS.
- M. Hezarjaribi, M. Jahanshahi, A. Rahimpour and M. Yaldagard, Gas diffusion electrode based on electrospun PANI/CNF nanofibers hybrid for proton exchange membrane fuel cells (PEMFC) applications, Appl. Surf. Sci., 2014, 295, 144–149 CrossRef CAS.
- M. K. Shin, Y. J. Kim, S. I. Kim, S.-K. Kim, H. Lee, G. M. Spinks and S. J. Kim, Enhanced conductivity of aligned PANI/PEO/MWNT nanofibers by electrospinning, Sens. Actuators, B, 2008, 134, 122–126 CrossRef CAS.
- P. Kar and A. Choudhury, Carboxylic acid functionalized multi-walled carbon nanotube doped polyaniline for chloroform sensors, Sens. Actuators, B, 2013, 183, 25–33 CrossRef CAS.
- M. Kumar, K. Singh, S. K. Dhawan, K. Tharanikkarasu, J. S. Chung, B.-S. Kong, E. J. Kim and S. H. Hur, Synthesis and characterization of covalently-grafted graphene–polyaniline nanocomposites and its use in a supercapacitor, Chem. Eng. J., 2013, 231, 397–405 CrossRef CAS.
- J. Luo, S. Jiang, R. Liu, Y. Zhang and X. Liu, Synthesis of water dispersible polyaniline/poly(styrenesulfonic acid) modified graphene composite and its electrochemical properties, Electrochim. Acta, 2013, 96, 103–109 CrossRef CAS.
- Y. Li, H. Peng, G. Li and K. Chen, Synthesis and electrochemical performance of sandwich-like polyaniline/graphene composite nanosheets, Eur. Polym. J., 2012, 48, 1406–1412 CrossRef CAS.
- Y. F. Huang and C. W. Lin, Facile synthesis and morphology control of graphene oxide/polyaniline nanocomposites via in situ polymerization process, Polymer, 2012, 53, 2574–2582 CrossRef CAS.
- R. Li, L. Liu and F. Yang, Preparation of polyaniline/reduced graphene oxide nanocomposite
and its application in adsorption of aqueous Hg(II), Chem. Eng. J., 2013, 229, 460–468 CrossRef CAS.
- S. Ameen, M. S. Akhtar, Y. S. Kim and H. S. Shin, Synthesis and electrochemical impedance properties of CdS nanoparticles decorated polyaniline nanorods, Chem. Eng. J., 2012, 181–182, 806–812 CrossRef CAS.
- B. T. Raut, P. R. Godse, S. G. Pawar, M. A. Chougule, D. K. Bandgar, S. Sen and V. B. Patil, New process for fabrication of polyaniline–CdS nanocomposites: Structural, morphological and optoelectronic investigations, J. Phys. Chem. Solids, 2013, 74, 236–244 CrossRef CAS.
- E. Tuncer and E. Turac, Synthesis and Characterization of Polyaniline/Zinc Sulfite Composite Films and Investigation of Properties, Adv. Polym. Technol., 2013, 32 DOI:10.1002/adv.21373.
- H. Zhuang, J.-B. Liu, Q.-H. Zeng and X.-G. Kong, Effect of Polyaniline as Hole-Acceptor and Energy-Acceptor on the Photoluminescence of CdSe, Chem. Res. Chin. Univ., 2007, 23, 131–134 CrossRef CAS.
- Y. Haldorai, V. H. Nguyen and J.-J. Shim, Synthesis of polyaniline/Q-CdSe composite via ultrasonically assisted dynamic inverse emulsion polymerization, Colloid Polym. Sci., 2011, 289, 849–854 CAS.
- V. Zucolotto, M. Ferreira, M. R. Cordeiro, C. J. L. Constantino, W. C. Moreira and O. N. Oliveira Jr, Nanoscale processing of polyaniline and phthalocyanines for sensing applications, Sens. Actuators, B, 2006, 113, 809–815 CrossRef CAS.
- J. C. B. Santos, L. G. Paterno, E. A. T. Dirani, F. J. Fonseca and A. M. de Andrade, Influence of polyaniline and phthalocyanine hole-transport layers on the electrical performance of light-emitting diodes using MEH-PPV as emissive material, Thin Solid Films, 2008, 516, 3184–3188 CrossRef CAS.
- X. Lu, D. Shan, J. Yang, B. Huang and X. Zhou, Determination of m-dinitrobenzene based on novel type of sensor using thiol-porphyrin mixed monolayer-tethered polyaniline with intercalating fullerenols, Talanta, 2013, 115, 457–461 CrossRef CAS PubMed.
- Q. Zhou, C. Li, J. Li and J. Lu, Electrocatalysis of template electrosynthesized cobalt-porphyrin/polyaniline nanocomposite for oxygen reduction, J. Phys. Chem. C, 2008, 112, 18578–18583 CAS.
- S. Adhikari and P. Banerji, Polyaniline composite by in situ polymerization on a swollen PVA gel, Synth. Met., 2009, 159, 2519–2524 CrossRef CAS.
- A. K. Bajpai, J. Bajpai and S. N. Soni, Designing Polyaniline (PANI) and Polyvinyl Alcohol (PVA) Based Electrically Conductive Nanocomposites: Preparation, Characterization and Blood Compatible Study, J. Macromol. Sci., Pure Appl. Chem., 2009, 46, 774–782 CrossRef CAS.
- M. C. Arenas, G. Sanchez, O. Martinez-Alvarez and V. M. Castano, Electrical and morphological properties of polyaniline–polyvinyl alcohol in situ nanocomposites, Composites, Part B, 2014, 56, 857–861 CrossRef CAS.
- D. S. Patil, J. S. Shaikh, D. S. Dalavi, S. S. Kalagi and P. S. Patil, Chemical synthesis of highly stable PVA/PANI films for supercapacitor application, Mater. Chem. Phys., 2011, 128, 449–455 CrossRef CAS.
- P. L. B. Araujo, E. S. Araujo, R. F. S. Santos and A. P. L. Pacheco, Synthesis and morphological characterization of PMMA/polyaniline nanofiber composites, Microelectron. J., 2005, 36, 1055–1057 CrossRef CAS.
- G. Panthi, N. A. Barakat, A. M. Hamza, A. R. Unnithan, M. Motlak, K. A. Khalil, Y.-S. Shin and H. Y. Kim, Polyaniline-Poly(vinyl acetate) Electrospun Nanofiber Mats as Novel Organic Semiconductor Material, Sci. Adv. Mater., 2012, 4, 1118–1126 CrossRef CAS.
- J. B. Veluru, K. K. Satheesh, D. C. Trivedi, V. R. Murthy and N. T. Srinivasan, Electrical properties of electrospun fibers of PANI–PMMA composites, J. Eng. Fibers Fabr., 2007, 2, 25–31 CAS.
- X. Lu, H. Dou, S. Yang, L. Hao, L. Zhang, L. Shen, F. Zhang and X. Zhang, Fabrication and electrochemical capacitance of hierarchical graphene/polyaniline/carbon nanotube ternary composite film, Electrochim. Acta, 2011, 56, 9224–9232 CrossRef CAS.
- Q. Cheng, J. Tang, N. Shinya and L.-C. Qin, Polyaniline modified graphene and carbon nanotube composite electrode for asymmetric supercapacitors of high energy density, J. Power Sources, 2013, 241, 423–428 CrossRef CAS.
- J. Yan, T. Wei, Z. Fan, W. Qian, M. Zhang, X. Shen and F. Wei, Preparation of graphene nanosheet/carbon nanotube/polyaniline composite as electrode material for supercapacitors, J. Power Sources, 2010, 195, 3041–3045 CrossRef CAS.
- Z.-D. Huang, R. Liang, B. Zhang, Y.-B. He and J.-K. Kim, Evolution of flexible 3D graphene oxide/carbon nanotube/polyaniline composite papers and their supercapacitive performance, Compos. Sci. Technol., 2013, 88, 126–133 CrossRef CAS.
- S. Wang, H. Bao, P. Yang and G. Chen, Immobilization of trypsin in polyaniline-coated nano-Fe3O4/carbon nanotube composite for protein digestion, Anal. Chim. Acta, 2008, 612, 182–189 CrossRef CAS PubMed.
- N. G. Shimpi, D. P. Hansora, R. Yadava and S. Mishra, Performance of hybrid nanostructured conductive cotton threads as LPG sensor at ambient temperature: preparation and analysis, RSC Adv., 2015, 5, 99253–99269 RSC.
- J. Zhao, Y. Xie, C. Yu, Z. Le, R. Zhong, Y. Qin, J. Pan and F. Liu, Preparation and characterization of the graphene–carbon nanotube/CoFe2O4/polyaniline composite with reticular branch structures, Mater. Chem. Phys., 2013, 142, 395–402 CrossRef CAS.
- X.-W. Hu, C.-J. Mao, J.-M. Song, H.-L. Niu, S.-Y. Zhang and H.-P. Huang, Fabrication of GO/PANI/CdSe nanocomposites for sensitive electrochemiluminescence biosensor, Biosens. Bioelectron., 2013, 41, 372–378 CrossRef CAS PubMed.
- H. Xu, X. Chen, J. Zhang, J. Wang, B. Cao and D. Cui, NO2 gas sensing with SnO2–ZnO/PANI composite thick film fabricated from porous nanosolid, Sens. Actuators, B, 2013, 176, 166–173 CrossRef CAS.
- P. Boomi and H. G. Prabu, Synthesis, characterization and antibacterial analysis of polyaniline/Au–Pd nanocomposite, Colloids Surf., A, 2013, 429, 51–59 CrossRef CAS.
- P. Boomi, H. G. Prabu and J. Mathiyarasu, Synthesis and characterization of polyaniline/Ag–Pt nanocomposite for improved antibacterial activity, Colloids Surf., B, 2013, 103, 9–14 CrossRef CAS PubMed.
- P. Boomi, H. G. Prabu and J. Mathiyarasu, Synthesis, characterization and antibacterial activity of polyaniline/Pt–Pd nanocomposite, Eur. J. Med. Chem., 2014, 72, 18–25 CrossRef CAS PubMed.
- Q. Yu, M. Shi, Y. Cheng, M. Wang and H.-Z. Chen, Fe3O4@Au/polyaniline multifunctional nanocomposites: their preparation and optical, electrical and magnetic properties, Nanotechnology, 2008, 19, 265702, DOI:10.1088/0957-4484/19/26/265702.
- Y. Lee, E. Kim, K. Kim, B. H. Lee and S. Choe, Polyaniline effect on the conductivity of the PMMA/Ag hybrid composite, Colloids Surf., A, 2012, 396, 195–202 CrossRef CAS.
- C.-Y. Li, W.-Y. Chiu and T.-M. Don, Polyurethane/Polyaniline and Polyurethane-Poly(methylmethacrylate)/Polyaniline Conductive Core-Shell Particles: Preparation, Morphology, and Conductivity, J. Polym. Sci., Part A: Polym. Chem., 2007, 45, 3902–3911 CrossRef CAS.
- T. Jeevananda and Siddaramaiah, Synthesis and characterization of polyaniline filled PU/PMMA interpenetrating polymer networks, Eur. Polym. J., 2003, 39, 569–578 CrossRef CAS.
- J. Janata, Principles of Chemical Sensors, Springer, New York, 4th edn, 2009 Search PubMed.
- H. Bai and G. Shi, Gas Sensors Based on Conducting Polymers, Sensors, 2007, 7, 267–307 CrossRef CAS.
- A. A. Athawale, S. V. Bhagwat and P. P. Katre, Nanocomposite of Pd-polyaniline as a selective methanol sensor, Sens. Actuators, B, 2006, 114, 263–267 CrossRef CAS.
- A. Choudhury, Polyaniline/silver nanocomposites. Dielectric properties and ethanol vapour sensitivity, Sens. Actuators, B, 2009, 138, 318–325 CrossRef CAS.
- Z.-F. Li, F. D. Blum, M. F. Bertino and C.-S. Kim, Understanding the response of nanostructured polyaniline gas sensors, Sens. Actuators, B, 2013, 183, 419–427 CrossRef CAS.
- S. Sharma, C. Nirkhe, S. Pethkar and A. A. Athawale, Chloroform vapour sensor based on copper/polyaniline nanocomposite, Sens. Actuators, B, 2002, 85, 131–136 CrossRef CAS.
- S. G. Pawar, S. L. Patil, M. A. Chougule, P. R. Godse, D. K. Bandgar and V. B. Patil, Fabrication of Polyaniline/TiO2 Nanocomposite Ammonia Vapor Sensor, J. Nano- Electron. Phys., 2011, 3, 1056–1063 Search PubMed.
- M. Dhingra, L. Kumar, S. Shrivastava, P. Senthil Kumar and S. Annapoorni, Impact of interfacial interactions on optical and ammonia sensing in zinc oxide/polyaniline nanostructures, Bull. Mater. Sci., 2013, 36, 647–652 CrossRef CAS.
- N. G. Deshpande, Y. G. Gudage, R. P. Sharma, J. C. Vyas, J. B. Kim and Y. P. Lee, Studies on tin oxide-intercalated polyaniline nanocomposite for ammonia gas sensing applications, Sens. Actuators, B, 2009, 138, 76–84 CrossRef CAS.
- G. D. Khuspe, S. T. Navale, M. A. Chougule and V. B. Patil, Ammonia gas sensing properties of CSA doped PANI-SnO2 nanohybrid thin films, Synth. Met., 2013, 185–186, 1–8 CAS.
- H.-D. Zhang, C.-C. Tang, Y.-Z. Long, J.-C. Zhang, R. Huanga, J.-J. Li and C.-Z. Gu, High-sensitivity gas sensors based on arranged polyaniline/PMMAcomposite fibers, Sens. Actuators, A, 2014, 219, 123–127 CrossRef CAS.
- L. Zhihua, Z. Xucheng, S. Jiyong, Z. Xiaobo, H. Xiaowei, H. E. Tahir and M. Holmes, Fast response ammonia sensor based on porous thin film of polyaniline/sulfonated nickel phthalocyanine composites, Sens. Actuators, B, 2016, 226, 553–562 CrossRef.
- K. Crowley, A. Morrin, R. L. Shepherd, M. in het Panhuis, G. G. Wallace, M. R. Smyth and A. J. Killard, Fabrication of Polyaniline-Based Gas Sensors Using Piezoelectric Inkjet and Screen Printing for the Detection of Hydrogen Sulfide, IEEE Sens. J., 2010, 10, 1419–1426 CrossRef CAS.
- J. Sarfraz, P. Ihalainen, A. Määttänen, J. Peltonen and M. Lindén, Printed hydrogen sulfide gas sensor on paper substrate based on polyaniline composite, Thin Solid Films, 2013, 534, 621–628 CrossRef CAS.
- B. T. Raut, P. R. Godse, S. G. Pawar, M. A. Chougule, D. K. Bandgar and V. B. Patil, Novel method for fabrication of polyaniline–CdS sensor for H2S gas detection, Measurement, 2012, 45, 94–100 CrossRef.
- M. D. Shirsat, M. A. Bangar, M. A. Deshusses, N. V. Myung and A. Mulchandani, Polyaniline nanowires-gold nanoparticles hybrid network based chemiresistive hydrogen sulfide sensor, Appl. Phys. Lett., 2009, 94, 083502–083504 CrossRef.
- S. S. Joshi, C. D. Lokhande and S.-H. Han, A room temperature liquefied petroleum gas sensor based on all-electrodeposited n-CdSe/p-polyaniline junction, Sens. Actuators, B, 2007, 123, 240–245 CrossRef CAS.
- D. S. Dhawale, R. R. Salunkhe, U. M. Patil, K. V. Gurav, A. M. More and C. D. Lokhande, Room temperature liquefied petroleum gas (LPG) sensor based on p-polyaniline/n-TiO2 heterojunction, Sens. Actuators, B, 2008, 134, 988–992 CrossRef CAS.
- D. S. Dhawale, D. P. Dubal, V. S. Jamadade, R. R. Salunkhe, S. S. Joshi and C. D. Lokhande, Room temperature LPG sensor based on n-CdS/p-polyaniline heterojunction, Sens. Actuators, B, 2010, 145, 205–210 CrossRef CAS.
- D. S. Dhawale, D. P. Dubal, A. M. More, T. P. Gujar and C. D. Lokhande, Room temperature liquefied petroleum gas (LPG) sensor, Sens. Actuators, B, 2010, 147, 488–494 CrossRef CAS.
- S. K. Shukla, M. Vamakshi, A. Bharadavaja, A. Shekhar and A. Tiwari, Fabrication of electro-chemical humidity sensor based on zinc oxide/polyaniline nanocomposites, Adv. Mater. Lett., 2012, 3(5), 421–425 CAS.
- A. Vijayan, M. Fuke, R. Hawaldar, M. Kulkarni, D. Amalnerkar and R. C. Aiyer, Optical fibre based humidity sensor using Co-polyaniline clad, Sens. Actuators, B, 2008, 129, 106–112 CrossRef CAS.
- M. V. Fuke, P. Kanitkar, M. Kulkarni, B. B. Kale and R. C. Aiyer, Effect of particle size variation of Ag nanoparticles in Polyaniline composite on humidity sensing, Talanta, 2010, 81, 320–326 CrossRef CAS PubMed.
- N. Parvatikar, S. Jain, S. Khasim, M. Revansiddappa, S. V. Bhoraskar and M. V. N. Ambika Prasad, Electrical and humidity sensing properties of polyaniline/WO3 composites, Sens. Actuators, B, 2006, 114, 599–603 CrossRef CAS.
- K. C. Sajjan, M. Faisal, S. C. Vijaya Kumari, Y. T. Ravikiran and S. Khasim, Proceeding of International Conference on Recent Trends in Applied Physics and Material Science: RAM 2013, AIP Conf. Proc., 2013, 1536, 289–290, DOI:10.1063/1.4810214.
- R. S. Diggikar, M. V. Kulkarni, G. M. Kale and B. B. Kale, Formation of multifunctional nanocomposites with ultrathin layers of polyaniline (PANI) on silver vanadium oxide (SVO) nanospheres by in situ polymerization, J. Mater. Chem. A, 2013, 1, 3992–4001 CAS.
- P. Cavallo, D. Acevedo, M. C. Fuertes, G. J. A. A. Soler-Illia and C. Barbero, Understanding the sensing mechanism of polyaniline resistive sensors. Effect of humidity on sensing of organic volatiles, Sens. Actuators, B, 2015, 210, 574–580 CrossRef CAS.
- X. Yan, G. Xie, X. Du, H. Tai and Y. Jiang, Preparation and characterization of polyaniline/indium(III) oxide (PANI/In2O3) nanocomposite thin film, Proc. SPIE 7282, 4th International Symposium on Advanced Optical Manufacturing and Testing Technologies: Advanced Optical Manufacturing Technologies, 72823C, 21 May 2009, DOI:10.1117/12.831026.
- M. K. Ram, O. Yavuz, V. Lahsangah and M. Aldissi, CO gas sensing from ultrathin nanocomposite conducting polymer film, Sens. Actuators, B, 2005, 106, 750–757 CrossRef CAS.
- I. Kim, K.-Y. Dong, B.-K. Ju and H. H. Choi, Gas sensor for CO and NH3 using polyaniline/CNTs composite at room temperature, Nanotechnology (IEEE-NANO), 2010 10th IEEE Conference, Seoul, 2010, pp. 466–469, DOI:10.1109/nano.2010.5697782.
- J. Yun, S. Jeon and H.-I. Kim, Improvement of NO Gas Sensing Properties of Polyaniline/MWCNT Composite by Photocatalytic Effect of TiO2, J. Nanomater., 2013, 2013, 184345, DOI:10.1155/2013/184345.
- H. Xu, X. Chen, J. Zhang, J. Wang, B. Cao and D. Cui, NO2 gas sensing with SnO2–ZnO/PANI composite thick film fabricated from porous nanosolid, Sens. Actuators, B, 2013, 176, 166–173 CrossRef CAS.
- S. Srivastava, S. Kumar, S. Agrawal, A. Saxena, B. L. Choudhary, S. Mathur, M. Singh and Y. K. Vijay, TiO2/PANI and MWNT/PANI Composites Thin Films for Hydrogen Gas Sensing, International Conference on Physics of Emerging Functional Materials (PEFM-2010), ed. D. K. Aswal and A. K. Debnath, Mumbai, India, 2010, vol. 1313, ISBN: 978-0-7354-0868-5 Search PubMed.
- R. Arsat, X. He, P. Spizzirri, M. Shafiei, M. Arsat and W. Wlodarski, Hydrogen Gas Sensor Based on Highly Ordered Polyaniline/Multiwall Carbon Nanotubes Composite, Sens. Lett., 2011, 9(2), 940–943 CrossRef CAS.
- A. Sadek, W. Wlodarski, K. Shin, R. Kaner and K. Kalantar-Zadeh, A polyaniline/WO3 nanofiber composite-based ZnO/64° YX LiNbO(3)SAW hydrogen gas sensor, Synth. Met., 2008, 158, 29–32 CrossRef CAS.
- C. Conn, S. Sestak, A. T. Baker and J. Unsworth, A Polyaniline-Based Selective Hydrogen Sensor, Electroanalysis, 1998, 10(16), 1137–1141 CrossRef CAS.
- M. E. Azim-Araghi and M. J. Jafari, Electrical and gas sensing properties of polyaniline–chloroaluminium phthalocyanine composite thin films, Eur. Phys. J.: Appl. Phys., 2010, 52, 10402–10407 CrossRef.
- S. J. Updike and G. P. Hicks, The Enzyme Electrode, Nature, 1976, 214, 986–988 CrossRef.
- A. A. Karyakin, Biosensors, in Sensors for Environment, Health and Security, ed. M. I. Baraton, Springer, The Netherlands, 2009, pp. 255–262 Search PubMed.
- C. Dhanda, M. Dasa, M. Datta and B. D. Malhotra, Recent advances in polyaniline based biosensors, Biosens. Bioelectron., 2011, 26, 2811–2821 CrossRef PubMed.
- D. Wei and A. Ivaska, Electrochemical Biosensors Based on Polyaniline, Chem. Anal., 2006, 51, 839–852 CAS.
- M. D. Imisides, R. John and G. G. Wallace, Microsensors based on conducting polymers, CHEMTECH, 1996, 261, 9–25 Search PubMed.
- Z. Muhammad-Tahir and E. C. Alocilja, A conductometric biosensor for biosecurity, Biosens. Bioelectron., 2003, 18, 813 CrossRef CAS PubMed.
- A. Malinauskas, R. Garjonyte, R. Mazeikiene and I. Jureviciute, Electrochemical response of ascorbic acid at conducting and electrogenerated polymer modified electrodes for electroanalytical applications: a review, Talanta, 2004, 64, 121–129 CrossRef CAS PubMed.
- D. T. Hoa, T. N. Suresh Kunar, N. S. Punekar, R. S. Srinivasa, R. Lal and A. Q. Contractor, A biosensor based on conducting polymers, Anal. Chem., 1992, 64, 2645–2646 CrossRef CAS.
- L. C. Clark and C. Lyons, Electrode systems for continuous monitoring in cardiovascular surgery, Ann. N. Y. Acad. Sci., 1962, 102, 29–45 CrossRef CAS PubMed.
- Y. Xian, Y. Hu, F. Liu, Y. Xiang, H. Wang and L. Jin, Glucose baiosensor based on Au nanoparticles-conductive polyaniline nanocomposite, Biosens. Bioelectron., 2006, 21, 1996–2000 CrossRef CAS PubMed.
- Q. Xu, S.-X. Gu, L. Jin, Y. E. Zhou, Z. Yang, W. Wang and X. Hu, Graphene/polyaniline/gold nanoparticles nanocomposite for the direct electron transfer of glucose oxidase and glucose biosensing, Sens. Actuators, B, 2014, 190, 562–569 CrossRef CAS.
- A. I. Gopalan, K. P. Lee, D. Ragupathy, S. H. Lee and J. W. Lee, An electrochemical glucose biosensor exploiting a polyaniline grafted multiwalled carbon nanotube/perfluorosulfonate ionomer–silica nanocomposite, Biomaterials, 2009, 30, 5999–6005 CrossRef CAS PubMed.
- H. Zhong, R. Yuan, Y. Chai, W. Li, X. Zhong and Y. Zhang, In situ chemo-synthesized multi-wall carbon nanotube-conductive polyaniline nanocomposites: characterization and application for a glucose amperometric biosensor, Talanta, 2011, 85(1), 104–111 CrossRef CAS PubMed.
- T. H. Le, N. T. Trinh, L. H. Nguyen, H. B. Nguyen, V. A. Nguyen, D. L. Tran and T. D. Nguyen, Electrosynthesis of polyaniline–multiwalled carbon nanotubes nanocomposite films in the presence of sodium dodecyl sulfate for glucose biosensing, Adv. Nat. Sci.: Nanosci. Nanotechnol., 2013, 4, 025014, DOI:10.1088/2043-6262/4/2/025014.
- J. Zhu, X. Liu, X. Wang, X. Huo and R. Yan, Preparation of polyaniline-TiO2 nanotube composite for the development of electrochemical biosensors, Sens. Actuators, B, 2015, 221, 450–457 CrossRef CAS.
- C. Ozdemir, F. Yeni, D. Odaci and S. Timur, Electrochemical glucose biosensing by pyranose oxidase immobilized in gold nanoparticle-polyaniline/AgCl/gelatin nanocomposite matrix, Food Chem., 2010, 119, 380–385 CrossRef CAS.
- M. Srivastava, S. K. Srivastava, N. R. Nirala and R. Prakash, Chitosan-based Polyaniline-Au Nanocomposite Biosensor for Determination of Cholesterol, Anal. Methods, 2014, 6, 817–824 RSC.
- N. Ruecha, R. Rangkupan, N. Rodthongkum and O. Chailapakul, Novel paper-based cholesterol biosensor using graphene/polyvinylpyrrolidone/polyaniline nanocomposite, Biosens. Bioelectron., 2014, 52, 13–19 CrossRef CAS PubMed.
- R. K. Basniwal, R. P. Singh Chauhan, S. Parvez and V. K. Jain, Development of a Cholesterol Biosensor by Chronoamperometric Deposition of Polyaniline–Ag Nanocomposites, Int. J. Polym. Mater. Polym. Biomater., 2013, 62, 493–498 CrossRef CAS.
- C. Dhand, S. K. Arya, M. Datta and B. D. Malhotra, Polyaniline–carbon nanotube composite film for cholesterol biosensor, Anal. Biochem., 2008, 383, 194–199 CrossRef CAS PubMed.
- A. Barik, P. R. Solanki, A. Kaushik, A. Ali, M. K. Pandey, C. G. Kim and B. D. Malhotra, Polyaniline–Carboxymethyl Cellulose Nanocomposite for Cholesterol Detection, J. Nanosci. Nanotechnol., 2010, 10, 6479–6488 CrossRef CAS PubMed.
- D. Saini, R. Chauhan, P. R. Solanki and T. Basu, Gold-Nanoparticle Decorated Graphene-Nanostructured Polyaniline Nanocomposite-Based Bienzymatic Platform for Cholesterol Sensing, ISRN Nanotechnol., 2012 DOI:10.5402/2012/102543.
- B. D'Autreaux and M. B. Toledano, ROS as signaling molecules: mechanisms that generate specificity in ROS homeostasis, Nat. Rev. Mol. Cell Biol., 2007, 8, 813–824 CrossRef PubMed.
- M. Giorgio, M. Trinei, E. Migliaccio and P. G. Pelicci, Hydrogen peroxide: a metabolic by-product or a common mediator of ageing signals?, Nat. Rev. Mol. Cell Biol., 2007, 8, 722–728 CrossRef CAS PubMed.
- S. G. Rhee, Cell signaling. H2O2, a necessary evil for cell signaling, Science, 2006, 312, 1882–1883 CrossRef PubMed.
- S. G. Rhee, T.-S. Chang, W. Jeong and D. Kang, Methods for Detection and Measurement of Hydrogen Peroxide Inside and Outside of Cells, Mol. Cells, 2010, 29, 539–549 CrossRef CAS PubMed.
- A. Al-Ahmed, P. M. Ndangili, N. Jahed, P. G. L. Baker and E. I. Iwuoha, Polyester Sulphonic Acid Interstitial Nanocomposite Platform for Peroxide Biosensor, Sensors, 2009, 9(12), 9965–9976 CrossRef CAS PubMed.
- Z. Du, C. Li, L. Li, M. Zhang, S. Xu and T. Wang, Simple fabrication of a sensitive hydrogen peroxide biosensor using enzymes immobilized in processable polyaniline nanofibers/chitosan film, Mater. Sci. Eng., C, 2009, 29, 1794–1797 CrossRef CAS.
- R. Devi and C. S. Pundir, Construction and application of an amperometric uric acid biosensor based on covalent immobilization of uricase on iron oxide nanoparticles/chitosan-g-polyaniline composite film electrodeposited on Pt electrode, Sens. Actuators, B, 2014, 193, 608–615 CrossRef CAS.
- R. Rawal, S. Chawla, N. Chauhan, T. Dahiya and C. S. Pundir, Construction of amperometric uric acid biosensor based on uricase immobilized on PBNPs/cMWCNT/PANI/Au composite, Int. J. Biol. Macromol., 2012, 50(1), 112–118 CrossRef CAS PubMed.
- S. Yadav, A. Kumar and C. S. Pundir, Amperometric creatinine biosensor based on covalently coimmobilized enzymes onto carboxylated multiwalled carbon nanotubes/polyaniline composite film, Anal. Biochem., 2011, 419, 277–283 CrossRef CAS PubMed.
- S. Yadav, R. Devi, P. Bhar, S. Singhla and C. S. Pundir, Immobilization of creatininase, creatinase and sarcosine oxidase on iron oxide nanoparticles/chitosan-g-polyaniline modified Pt electrode for detection of creatinine, Enzyme Microb. Technol., 2012, 50, 247–254 CrossRef CAS PubMed.
- M. Zhybak, V. Beni, M. Y. Vagin, E. Dempsey, A. P. F. Turner and Y. Korpan, Creatinine and urea biosensors based on a novel ammonium ion-selective copper-polyaniline nano-composite, Biosens. Bioelectron., 2016, 77, 505–511 CrossRef CAS PubMed.
- J. Huang, Q. Lin, X. Zhang, X. He, X. Xing, W. Lian, M. Zuo and Q. Zhang, Electrochemical immunosensor based on polyaniline/poly(acrylic acid) and Au-hybrid graphene nanocomposite for sensitivity enhanced detection of salbutamol, Food Res. Int., 2011, 44, 92–97 CrossRef CAS.
- P.-Z. Liu, X.-W. Hu, C.-J. Mao, H.-L. Niu, J.-M. Song, B.-K. Jin and S.-Y. Zhang, Electrochemiluminescence immunosensor based on graphene oxide nanosheets/polyaniline nanowires/CdSe quantum dots nanocomposites for ultrasensitive determination of human interleukin-6, Electrochim. Acta, 2013, 113, 176–180 CrossRef CAS.
- A. Dey, A. Kaushik, S. K. Arya and S. Bhansali, Mediator free highly sensitive polyaniline–gold hybrid nanocomposite based immunosensor for prostate-specific antigen (PSA) detection, J. Mater. Chem., 2012, 22, 14763–14772 RSC.
- X. Sun, L. Qiao and X. Wang, A Novel Immunosensor Based on Au Nanoparticles and Polyaniline/Multiwall Carbon Nanotubes/Chitosan Nanocomposite Film Functionalized Interface, Nano-Micro Lett., 2013, 5(3), 191–201 CrossRef CAS.
- J. Li, S. Liu, J. Yu, W. Lian, M. Cui, W. Xu and J. Huang, Electrochemical immunosensor based on graphene–polyaniline composites and carboxylated graphene oxide for estradiol detection, Sens. Actuators, B, 2013, 188, 99–105 CrossRef CAS.
- M. Lin, Y. Liu, Z. Sun, S. Zhang, Z. Yang and C. Ni, Electrochemical immunoassay of benzo[a]pyrene based on dual amplification strategy of electron-accelerated Fe3O4/polyaniline platform and multi-enzyme-functionalized carbon sphere label, Anal. Chim. Acta, 2012, 722, 100–106 CrossRef CAS PubMed.
- W. Yan, X. Chen, X. Li, X. Feng and J.-J. Zhu, Fabrication of a Label-Free Electrochemical Immunosensor of Low-Density Lipoprotein, J. Phys. Chem. B, 2008, 112, 1275–1281 CrossRef CAS PubMed.
- J. Wu, Y. Zou, X. Li, H. Liu, G. Shen and R. Yu, A biosensor monitoring DNA hybridization based on polyaniline intercalated graphite oxide nanocomposite, Sens. Actuators, B, 2005, 104, 43–49 CrossRef CAS.
- Y. Bo, H. Yang, Y. Hu, T. Yao and S. Huang, A novel electrochemical DNA biosensor based on graphene and polyaniline nanowires, Electrochim. Acta, 2011, 56, 2676–2681 CrossRef CAS.
- M. Du, T. Yang, X. Li and K. Jiao, Fabrication of DNA/graphene/polyaniline nanocomplex for label-free voltammetric detection of DNA hybridization, Talanta, 2012, 88, 439–444 CrossRef CAS PubMed.
- L. Wang, E. Hua, M. Liang, C. Ma, Z. Liu, S. Sheng, M. Liu, G. Xie and W. Feng, Graphene sheets, polyaniline and AuNPs based DNA sensor for electrochemical determination of BCR/ABL fusion gene with functional hairpin probe, Biosens. Bioelectron., 2014, 51, 201–207 CrossRef CAS PubMed.
- J. Wilson, S. Radhakrishnan, C. Sumathi and V. Dharuman, Polypyrrole–polyaniline–Au (PPy–PANi–Au) nano composite films for label-free electrochemical DNA sensing, Sens. Actuators, B, 2012, 171–172, 216–222 CrossRef CAS.
- E. Spain, T. E. Keyes and R. J. Forster, Vapour phase polymerised polyaniline–gold nanoparticle composites for DNA detection, J. Electroanal. Chem., 2013, 711, 38–44 CrossRef CAS.
- E. Spain, R. Kojima, R. B. Kaner, G. G. Wallace, J. O'Grady, K. Lacey, T. Barry, T. E. Keyes and R. J. Forster, High sensitivity DNA detection using gold nanoparticle functionalised polyaniline nanofibers, Biosens. Bioelectron., 2011, 26, 2613–2618 CrossRef CAS PubMed.
- T. Yang, N. Zhou, Y. Zhang, W. Zhang, K. Jiao and G. Li, Synergistically improved sensitivity for the detection of specific DNA sequences using polyaniline nanofibers and multi-walled carbon nanotubes composites, Biosens. Bioelectron., 2009, 24, 2165–2170 CrossRef CAS PubMed.
- J. Yang, X. Wang and H. Shi, An electrochemical DNA biosensor for highly sensitive detection of phosphinothricin acetyltransferase gene sequence based on polyaniline-(mesoporous nanozirconia)/poly-tyrosine film, Sens. Actuators, B, 2012, 162, 178–183 CrossRef CAS.
- M. U. Anu Prathap, R. Srivastava and B. Satpati, Simultaneous detection of guanine, adenine, thymine, and cytosine at polyaniline/MnO2 modified electrode, Electrochim. Acta, 2013, 114, 285–295 CrossRef CAS.
- S. Radhakrishnan, C. Sumathi, V. Dharuman and J. Wilson, Polypyrrole nanotubes–polyaniline composite for DNA detection using methylene blue as intercalator, Anal. Methods, 2013, 5, 1010–1015 RSC.
- N. Prabhakar, G. Sumana, K. Arora, H. Singh and B. D. Malhotra, Improved electrochemical nucleic acid biosensor based on polyaniline-polyvinyl sulphonate, Electrochim. Acta, 2008, 53, 4344–4350 CrossRef CAS.
Footnotes |
† Glassy carbon electrode. |
‡ Micromolar. |
§ Picogram/milliliter. |
¶ Nanogram/milliliter. |
|| Picomolar. |
** Femtomolar. |
|
This journal is © The Royal Society of Chemistry 2016 |
Click here to see how this site uses Cookies. View our privacy policy here.