DOI:
10.1039/C6RA02947G
(Paper)
RSC Adv., 2016,
6, 35091-35101
Preparation of calcium silicate/decellularized porcine myocardial matrix crosslinked by procyanidins for cardiac tissue engineering
Received
1st February 2016
, Accepted 31st March 2016
First published on 4th April 2016
Abstract
Decellularized myocardial extracellular matrix (ECM) patches have promising potential for myocardial repair, due to the well-preserved compositional cues, good biocompatibility and non-immunogenicity. However, the lack of vascularization and their low mechanical properties, chemical instability caused by decellularization process limit their application in cardiac tissue engineering. Therefore, we used calcium silicate (CS) extracts interpenetration method to prepare CS-loaded bioactive scaffold, since CS bioceramic was reported to have stimulative effect on angiogenesis. Meanwhile, procyanidins (PC), a natural plant polyphenol, was utilized to crosslink the decellularized myocardial ECMs, aiming at enhancing the mechanical properties and resistance to enzymatic proteolysis. The results showed that PC-crosslinked pcECMs displayed great crosslinking stability and efficiency, improved tensile strength and chemical stability as compared with non-crosslinked group, and the enhancement was depended on the concentration of PC. The CS-loaded ECMs showed stable Ca and Si ions release during 7 days and the ion concentration was adjusted in the range with angiogenic potential by controlling the original concentration of CS extracts. Cytotoxicity assay showed that the CS-loaded pcECMs crosslinked by PC were cytocompatible for supporting the adhesion and proliferation of H9c2 cardiomyoblasts, endothelial cells and fibroblasts. Moreover, the CS incorporating could enhance the proliferation rate and angiogenic gene expression of endothelial cells effectively. These findings verified the feasibility of PC crosslinking and CS incorporation as a novel approach to prepare bioactive complex decellularized myocardial scaffold, which can be applied to cardiac tissue engineering for myocardial repair.
Introduction
Myocardial infarction (MI) and heart failure continue to be the leading cause of death worldwide.1 A series of pathological changes occur after MI, consisting of irreversible cell loss, scar formation, ventricular dilatation and eventually heart failure. Currently, the efficient remedy for patients with impaired cardiac function is heart transplantation or mechanical ventricular assist devices.2,3 But the therapeutic efficacy of these approaches is limited, due to the shortage of donor organs and thrombus risk. In recent years, treatments such as cell therapy are developed, which can prevent or reverse myocardial injury to a certain degree, but the poor survival and low engraftment efficiency of the donor cells limit its application.4–6 In the past decades, tissue engineering strategies have attracted much attention due to the promising perspective in clinical applications.7–9
Among various tissue engineering scaffolds, decellularized extracellular matrix (ECM) derived from myocardium has gained much interest, due to the unique advantages such as well-preserved biological properties, fiber architecture and good biocompatibility.10–12 However, the lack of vascularization has always been the obstacle of the thick cardiac tissue regeneration, as the absence of blood vessel will prevent the supply of nutrients and oxygen throughout the construct and thus hamper the long-term survival.13 In order to promote angiogenesis in tissue-engineered constructs, angiogenic growth factors such as vascular endothelial growth factor (VEGF) and basic fibroblast growth factor (bFGF) have been widely used and created great functions.14,15 Nevertheless, the uncontrollable release behavior in vivo, short half-life and the difficulty to maintain bioactivity of the protein during fabrication process limit their application in tissue engineering.16 Several studies demonstrated that some bioactive inorganic materials such as calcium silicate (CS) may take the place of inductive growth factors, since they could stimulate proliferation and angiogenic gene expression of endothelial cells in vitro,16–18 and blood vessel formation in vivo.19 Our previous studies have shown that CS extracts could up-regulate the expression of VEGF, bFGF and their receptors, activate eNOS expression and enhance the NO expression from endothelial cells.18 However, no studies have reported the incorporation of CS into the decellularized tissues for myocardial regeneration.
Another drawback of the decellularized tissues is the mechanical and enzymatic degradation stability of the matrix, as the collagen density of tissues may be reduced after continuous decellularization,20,21 which might eventually cause the failure of the graft in cardiac tissue regeneration. The common solution to solve this problem is the chemical crosslinking treatments, which will increase the durability of ECMs derived from different tissues and organs.22–25 However, this method has not been applied to decellularized myocardial ECMs. As the introduction of crosslinking reagent may have adverse effect on compliance and cytocompatibility of the scaffolds, it is of great importance to choose appropriate crosslinking reagent. Various crosslinking reagents such as glutaraldehyde,23 1-ethyl-3(3-dimethyl aminopropyl) carbodiimide hydrochloride24 and tannic acid25 have been widely used to stabilize bioprosthetic heart valves and vascular grafts. But problems such as calcification and cytotoxicity are also encountered with crosslinked cardiovascular tissues. Procyanidins (PC) is a natural plant polyphenol belonging to the flavone family with anti-calcification, anti-inflammatory and anti-thrombotic bioactivities.26 Our previous study has shown that PC-crosslinking can inhibit calcification of cardiovascular scaffolds by blocking hydroxylapatite nucleation sites and inhibiting some calcification factors such as the adhesion of inflammation cells and the secretion of MMPs.27 In addition, PC-crosslinking is reported to be cytocompatible and can improve the mechanical properties and stability of scaffolds effectively.28,29 Thus, PC may act as the candidate crosslinking reagent to stabilize decellularized myocardial tissue and meanwhile keep the good cytocompatibility of the construct.
Accordingly, our hypothesis is that incorporation of CS into the PC crosslinked decellularized myocardial matrix may result in a stable scaffold with angiogenic activity for cardiac tissue engineering application. Therefore, the aim of this study was to test the feasibility of PC crosslinking and CS incorporation as a novel approach for preparation of bioactive composite decellularized myocardial ECM scaffolds, and to evaluate the synergetic effect of PC crosslinking and CS incorporation on the myocardial scaffolds, including crosslinking stability, mechanical properties, resistance to enzymatic proteolysis and cytotoxicity on H9c2 cardiomyoblasts, fibroblasts and HUVECs.
Experimental
1. Scaffolds preparation
1.1 Decellularization of porcine myocardial tissues. Fresh porcine hearts were harvested from a local slaughterhouse and immersed in cold, sterile phosphate buffered saline (PBS), supplemented with antibacterial and antimycotic antibiotics (200 U ml−1 penicillin, 0.2 mg ml−1 streptomycin). After removing adipose tissue, the left ventricular wall was dissected parallel to the epicardial plain into 2 mm-thick tissue slices. Then the myocardial slices were decellularized according to the reported process.20 Briefly, the myocardial slices were soaked in 0.1% sodium dodecyl sulfate (SDS, Sigma) with 0.01% trypsin, 1 mM phenylmethylsulfonylfluoride (protease inhibitor), 20 μg ml−1 RNase A and 0.2 mg ml−1 DNase for 2.5 weeks. The ultrasonic treatment (50 Hz, KUDOS) was applied for 10 min each day, and the SDS solution was changed every other day. Finally, the obtained decellularized pcECMs were washed with PBS thoroughly to remove the residual substances.
1.2 Crosslinking process by PC. The decellularized pcECMs were crosslinked by soaking in PC (Tianjin Jianfeng Natural Product R&D Co., Ltd) in PBS for 12 h at 37 °C under continuous shaking (120 rpm). The concentrations of PC solutions were 0, 0.01, 0.05, 0.1, 0.5, 1.0 mg ml−1 respectively, and correspondingly the groups were defined as pcECM, P001, P005, P01, P05, P1. The ratio of the volume of PC solution to the surface area of myocardial matrix was 2 ml/1 cm2.
1.3 Preparation of CS-loaded pcECM patches. The powders of CS ceramics were synthesized according to the published method.30 Briefly, 1 M of Na2SiO3 aqueous solution were mixed with 1 M Ca(NO3)2 solution in equal volumes and underwent continuous stirring at room temperature overnight. Then the obtained CS precipitate was filtered, washed with deionized water and subsequently ethanol, and finally dried at 80 °C overnight and calcined at 800 °C for 2 h. The CS/pcECM patches were prepared by soaking decellularized pcECMs in the ionic extracts of CS bioceramics, which were prepared according to the reported method.18 In brief, 1 g of ceramic powder was soaked in 5 ml of serum-free Dulbecco's modified Eagle's medium-high glucose (DMEM-high, Gibco) or endothelial basal medium-2 (EBM-2; Lonza) and incubated in a 5% CO2 atmosphere at 37 °C for 24 h. Then the supernatant was sterilized through a filter (Millipore, 0.22 mm) and stored at 4 °C. After immersing in gradient concentrations of ionic extracts for 48 h, the pcECMs were frozen at 80 °C and lyophilized for further use. Samples crosslinked by 0.1 mg ml−1 PC and soaked in CS extracts diluted at 1/64, 1/16 and 1/4 were defined as P01/CS 1/64, P01/CS 1/16 and P01/CS 1/4 respectively.
2. Assessment of decellularization process
2.1 Histology. The native and decellularized myocardial tissues were fixed with 4% paraformaldehyde, sectioned to 10 μm sections and stained with hematoxylin and eosin (H&E) and Masson's trichrome (MT) to evaluate the cellular nucleus and ECM components. Four randomly selected optical fields (0.083 mm2) of the H&E staining were analysed and an image analysis software (Image-Pro Plus 6.0, Wayne Rasband, NIH, USA) was used to assess the ratio of the amount of cellular nuclei in the decellularized matrix to that in native tissue.
2.2 Quantification of collagen. The collagen content was determined by the hydroxyproline levels according to the reported method.31 Lyophilized native and decellularized myocardial tissues were weighed, hydrolyzed in 6 M HCl at 110 °C for 5 h and neutralized to pH 7. Then hydroxyproline was measured using p-dimethyl-aminobenzaldehyde (Sigma-Aldrich) and chloramine-T (Sigma-Aldrich). The optical density (OD) value of the mixture at 570 nm was measured using a UV-Vis absorption spectrophotometer (Shimadzu UV-3101PC). Finally the hydroxyproline levels were determined against a standard OD value-to-concentration curve of trans-L-hydroxyproline (Sigma-Aldrich) over serial concentrations. The amount of collagen content of the myocardial sample was calculated using a hydroxyproline-to-collagen ratio of 1
:
7.2.
2.3 Scanning electron microscope characterizations. The decellularized myocardial matrices specimens were fixed in 2.5% glutaraldehyde solution for 4 h, washed extensively in PBS, dehydrated in ascending concentrations of ethanol and then air dried. The dried specimens were sputter-coated with gold and mounted for imaging on a JSM-6700F scanning electron microscope (Jeol, Tokyo, Japan).
3. Dynamic release of PC from the crosslinked pcECMs
The concentration of released PC from the crosslinked scaffolds was measured according to the published method.32 Briefly, the crosslinked pcECMs were immersed in PBS (10 ml for every 1 cm2-area segment) at 37 °C for different periods (1–7 days). Then 3 ml vanillin solution (12% in methanol) and 6 ml HCl solution (1.2 M in methanol) were added into 1 ml PC-released solution and mixed. After 25 min, the OD value at 500 nm was measured spectrophotometrically and the concentration of PC was determined against the standard curve of PC solutions over serial concentrations. Also, the surplus PC solution after crosslinking was collected and the concentration of PC was measured. The total amount of crosslinked PC (ΔW%) was calculated according to the formula:
ΔW (%) =(W0 − VCt)/W0 × 100, |
where W0 represents the original weight of PC used in each sample before crosslinking, and Ct represents the concentration of the surplus PC solution after crosslinking. V = 100 ml.
4. Mechanical properties of non- and PC-crosslinked pcECMs
Each sample from individual group was cut along the fiber direction to yield one strip with a width of 4 mm and a length of 25 mm.33 Then the uniaxial tension test of pcECM straps (n = 6) were conducted using a Shimadzu material testing instrument at 2 mm min−1 rate. After measurement, the tensile strength and strain at break were obtained, and the ultimate elastic modulus was determined from the slope of the stress–strain curve.
5. In vitro enzymatic proteolysis
As type I collagen is the major component in pcECM,34 collagenase-I (Sigma-Aldrich, 386 U mg−1 solid) was used in the in vitro enzymatic degradation test to evaluate the stability of the scaffolds. Lyophilized samples were weighed, cut into pieces, immersed in 1000 U ml−1 collagenase-I in PBS (pH 7.4), and incubated at 37 °C for 24 h under continuous shaking.35 Ethylenediaminetetraacetic acid (EDTA; Sigma) solution (50 μl, 10 mM) were added to stop the enzymatic proteolysis and all residual specimens were removed by filtering through a 0.45 μm cellulose filter. Then the residual specimens were lyophilized and weighed. The relative weight loss (ΔW′%) was calculated according to the formula:
ΔW′ (%) = (W′0 − W′t)/W′0 × 100, |
where W′0 represents the original weight of each pcECM sample and W′t represents the weight of the corresponding sample after the collagenase-I proteolysis.
6. Dynamic release of ions from the CS-loaded pcECM patches
Samples (1 cm in diameter) were soaked in 300 μl DMEM-high and incubated in a humidified incubator at 37 °C with 5% CO2 for different time periods (1–7 days). At selected time point, the ion extracts were collected and measured in terms of the calcium and silicon (Ca and Si) ion concentrations by inductively coupled plasma optical emission spectroscopy (ICP-OES; Varian 715 ES, USA). The DMEM-high was changed every other day.
7. Cytotoxicity of pcECM patches
The effects of pcECM patches on cell adhesion and viability were evaluated using H9c2 cardiomyoblasts (CMs), human umbilical vein endothelial cells (HUVECs) and human dermal fibroblasts (HDFs). H9c2 CMs were obtained from the Cell Bank of the Chinese Academy of Sciences (Shanghai, China), and HUVECs were isolated from the human umbilical cord vein according to the published method.36 HDFs were isolated and cultured from the superficial layer of adult human skin dermatome at a depth of 400 μm according to the description by Sorrell JM.37 H9c2 CMs and HDF were cultured with DMEM-high supplemented with 10% fetal bovine serum (FBS, HyClone), and HUVEC was cultured with EBM-2 supplemented with 10% FBS and 1% endothelial cell growth supplement/heparin kit (ECGS/H, Promocell). All cells below passage 7 were used in this study.
Before culturing, all the samples were punctuated into circular membranes (1 cm in diameter), put into a 48-well plate and sterilized by UV-light irradiation. For the cell adhesion test, 8000 cells were pipetted slowly onto the scaffolds, and 1 h later 300 μl culture medium was added to the plates. After incubation for 24 h, the seeded myocardial matrices were collected for SEM observation. For cell viability test, samples were seeded with 5000 cells and incubated for different time periods (1, 3 and 7 days). At selected time points, the number of cells on the samples was evaluated by CCK-8 (Cell counting kit-8, Beyotime) assay according to the manufacturer's instruction. The absorbance was measured spectrophotometrically at 450 nm, with a reference wavelength of 650 nm. At the end of culture, the samples were rinsed twice in PBS and stained with a live/dead staining kit (Invitrogen) according to the manufacturer's instruction. Confocal images of stained cells on the matrices were captured using a confocal laser scanning microscope (Leica TCS SP8).
8. RNA isolation and quantitative reverse transcriptase PCR (QRT-PCR)
Samples (1 cm in diameter) in P01 and P01/CS 1/4 group were soaked in 300 μl EBM-2 supplemented with 10% FBS and 1% ECGS/H at 37 °C with 5% CO2 for 3 days. The ion extracts were collected and the culture medium was changed every day. For RNA extraction, 3 × 105 HUVECs were seeded onto the 6-well plates and allowed to adhere for 12 h. Then the culture medium was replaced by the collected ion extracts and incubated for 72 h. Total RNA was extracted from cells on the scaffolds using TRIzol reagent (Ambion) and chloroform according to the manufacturer's instructions. cDNA synthesis was performed using a ReverTra Ace-a kit (Toyobo Co. Ltd, Japan) according to the manufacturer's instructions. Gene expression was measured by quantitative real-time PCR. Reaction mixtures contained 1 μl aliquot of cDNA, which was diluted 1
:
10 in sterilized Mill-Q water, 9 μl of SYBR-Green and 9 μl forward or reverse primers. Real-time PCR analysis was performed using the 7900 Real-time PCR system (Applied Biosystems) using the following program: 95 °C for 1 min (stage 1); 95 °C for 15 s, 60 °C for 15 s and 72 °C for 45 s, for 40 cycles (stage 2). Each sample was run in triplicate. The output data from Rotor-Gene 6000 analysis software (version 1.7, Corbett Life Science) were transferred to Microsoft Excel for further analysis. The results were normalized to GAPDH and expressed as fold change for Blank (cells cultured with the EBM-2 culture medium). Primers and their sequences were listed in Table 1.
Table 1 Primer sequences used in quantitative real-time polymerase chain reaction
Gene |
Gene bank |
Primer sequences |
Tm (°C) |
VEGF165 |
AB_021221 |
F: 5′ TGCGGATCAAACCTCACCA 3′ |
58 |
R: 5′ CAGGGATTTTTCTTGTCTTGCT 3′ |
KDR |
NM_002253 |
F: 5′ GTGATCGGAAATGACACTGGAG 3′ |
60 |
R: 5′ CATGTTGGTCACTAACAGAAGCA 3′ |
GAPDH |
NM_002046 |
F: 5′ GATTTGGTCGTATTGGGCG 3′ |
60 |
R: 5′ CTGGAAGATGGTGATGG 3′ |
9. Statistical analysis
All data were analyzed and expressed as mean ± SD from deviation. At minimum, four samples were represented for each data point. Two-tailed Student's t-test was used for comparisons at single time points, whereas standard analysis of variance was used when groups were compared through time. The differences were considered significant at the level of p < 0.05.
Results
1. Macrostructure, histology and morphology of myocardial matrices before and after decellularization
After 2.5-week decellularization, the native porcine myocardium turned into white and shrunken extracellular matrix (Fig. 1A and B). Complete acellularity of pcECM was validated by histological staining and quantification of cellular nuclei (Fig. 1G). H&E staining showed that the cells with their nuclei in native myocardium were eliminated, leaving ECM fibers and gaps between fibers (Fig. 1C and D). MT staining showed that the component of pcECM fibers was mainly collagen (Fig. 1E and F). The collagen quantification (Fig. 1H) showed a slight decrease of collagen in pcECM (192.22 μg mg−1) as compared to that in the native myocardium (199.39 μg mg−1). SEM images of the microstructure of pcECM (Fig. 1I and J) showed an interconnective porous network of dense and weaved fibers without any cellular components. The pore size estimated from the SEM images was between 2 to 6 μm.
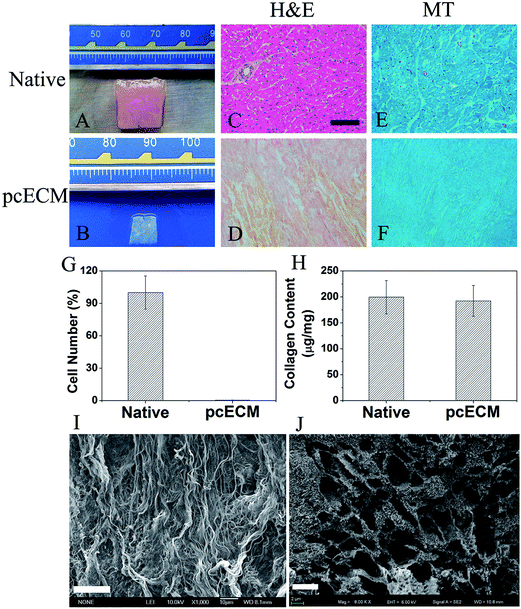 |
| Fig. 1 Characterization of decellularization process. Morphology (A and B), H&E (C and D), MT staining (E and F) and quantification of cell nuclei (G) and collagen (H) of the native myocardium and pcECM. H&E staining: red color and blue point represent ECM and cell nuclei respectively. MT staining: bright blue represents collagen. (I) and (J) represent the microstructure of pcECM longitudinally (I) and transversally (J) revealed by SEM. Scale bars in H&E and MT staining indicate 200 μm. Scale bars in (I) and (J) indicate 20 μm and 4 μm respectively. | |
2. Crosslinking stability of PC-crosslinked pcECMs
After PC crosslinking, the color of pcECMs changed gradually from white to crimson as the concentration of PC increased (Fig. 2A). The crosslinking stability of PC-crosslinked groups was assessed by dynamic release of PC when soaking in PBS for 7 days. The curves of dynamic release of PC (Fig. 2B) revealed that the surplus PC released rapidly from each crosslinked sample during the first day. Then the releasing rate became slower over soaking days and became non-detectable after seven days at the latest. The amounts of PC released from the crosslinked samples were all at a very low level, with the largest concentration 79.4 μg ml−1 found in 1 mg ml−1 PC-crosslinked group during the first day. After 7 days of soaking, the accumulative amount of crosslinked PC in all the crosslinked samples was calculated in the range of 62.0% (P1) to 89.2% (P001), and was found depended on the original concentrations of crosslinking reagent (Fig. 2C).
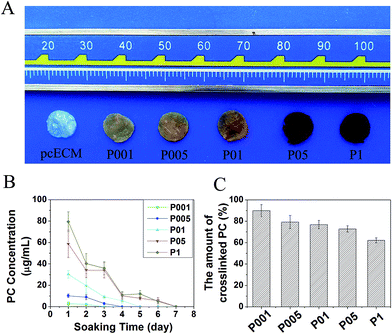 |
| Fig. 2 Morphology (A), dynamic release of surplus PC in PBS (B) and the accumulative amount of crosslinked PC (C) of PC-crosslinked pcECMs. | |
3. Mechanical properties of non- and PC-crosslinked pcECMs
Fig. 3 depicted the stress–strain curves of representative individual samples and quantitative analysis of mechanical properties of myocardial tissues. After decellularization, pcECM exhibited reduced tensile strength (468.59 kPa for pcECM, 844.06 kPa for native), elastic modulus (1.23 MPa for pcECM, 3.77 MPa for native) and increased strain at break (36.64% for pcECM, 30.74% for native) as compared with native myocardium (Fig. 3B–D). In contrast, the PC-crosslinked samples showed an increase in the ultimate tensile strength, elastic modulus and a decrease in strain at break as compared with non-crosslinked one. The 1.0 mg ml−1 PC-crosslinked samples exhibited the maximal tensile strength (1276.19 kPa), elastic modulus (6.03 MPa) and the minimal strain at break (19.05%). Especially, samples crosslinked with 0.05 or 0.1 mg ml−1 PC possessed the comparable mechanical properties to native myocardium, as the tensile strength (778.85 kPa for P005, 866.63 kPa for P01), elastic modulus (2.33 MPa for P005, 2.95 MPa for P01) and strain at break (36.84% for P005, 38.75% for P01) were similar to that of native myocardium.
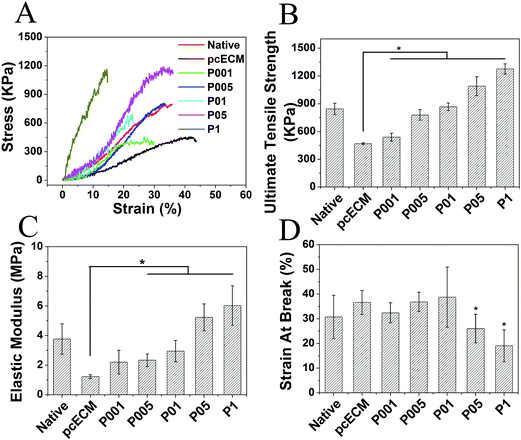 |
| Fig. 3 Uniaxial mechanical properties of the native myocardium, non- and PC-crosslinked pcECMs. (A) Stress–strain curves. (B) Ultimate tensile strength. (C) Elastic modulus. (D) Strian at break. Native: native myocardium. * represents significant difference (p < 0.05) compared with non-crosslinked pcECM. | |
4. In vitro resistance to enzymatic proteolysis
As shown in Fig. 4, 78.4% of non-crosslinked pcECMs were degraded after digestion by collagenase-I for 24 h, while after PC crosslinking, the resistance to enzymatic degradation of samples was improved. The relative weight loss of samples crosslinked by 0.01, 0.05, 0.1, 0.5, 1.0 mg ml−1 PC was 58.9%, 25.7%, 10.9%, 4.0% and 3.8% respectively, suggesting the decrease of the degradation rate of the matrix with the increase of the original PC concentration. Furthermore, when the PC concentration is higher than 0.1 mg ml−1, further increase of the PC concentration did not have significant effect on the degradation rate, suggesting that not much more reactive sites were available for further binding with PC.
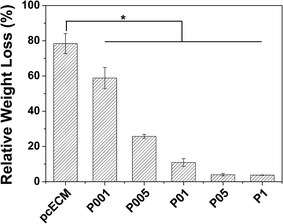 |
| Fig. 4 The relative weight loss of non- and PC-crosslinked pcECMs after in vitro enzymatic degradation by collagenase-I. * represents significant difference (p < 0.05) compared with non-crosslinked pcECM. | |
5. Cytocompatibility of non- and PC-crosslinked pcECMs
After 7 days of incubation, H9c2 CMs, HUVECs and HDFs viability was verified by CCK-8 assay (Fig. 5). The OD value of all the samples after 7 days' culture was almost 2-fold higher than that of 3 days' culture, which was similar to that on the plate (Blank). At the same culture period there was no obvious difference in cell proliferation between the non- and PC-crosslinked pcECMs, or among different crosslinked groups. The results demonstrated that PC-crosslinking treatment did not significantly affect metabolic activity and growth of CMs, HUVECs and HDFs.
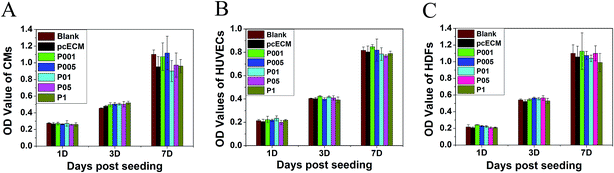 |
| Fig. 5 The CCK-8 assay of H9c2 CMs (A), HUVECs (B) and HDFs (C) on non- and PC-crosslinked pcECMs. | |
Above all, P01 group was chosen to be incorporated with CS, because of the most matched mechanical properties, chemical stability and great cytocompatibility. In order to assist the mechanical support and meanwhile keep the contractile activity of myocytes, the myocardial scaffold should possess the modest elastic modulus. Our results showed that the tensile strength and elastic modulus of the P01 group most resembled those of the native myocardium (Fig. 3), suggesting that the pcECM crosslinked by 0.1 mg ml−1 PC possessed the appropriate stiffness.
6. Dynamic release of ions from the CS-loaded pcECMs
Fig. 6 showed the release curves of Ca and Si ions from the CS-loaded pcECMs crosslinked by 0.1 mg ml−1 PC during 7 days. The concentration of Ca and Si ions (Fig. 6A and B) released from the scaffolds was depended on the original concentration of CS extracts. The Ca and Si ion concentrations of all groups were below 8 and 4 ppm respectively, and declined with the increase of soaking time until non-detectable after 7 days. Similarly, the accumulative amount of released ions was also dependent on the original concentration of CS extracts, and the highest concentration was found in P01/CS 1/4 group (17.6 ppm for Ca ion and 7.4 ppm for Si ion).
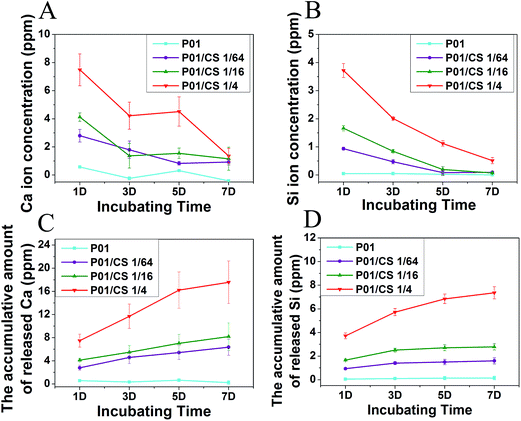 |
| Fig. 6 The releasing process of Ca (A and C) and Si (B and D) ions from the CS-loaded pcECM patches crosslinked by 0.1 mg ml−1 PC. (A and B) The change of ions concentration during 7 days. (C and D) The accumulative amount of released ions. The PBS solution was changed every other day. | |
7. Cytocompatibility of CS-loaded pcECMs
As shown in the CCK-8 assay (Fig. 7), the OD value of H9c2 CMs, HUVECs and HDFs increased with the increasing culture period. The cell viability on the scaffolds after 7 days of incubation increased with the increase of the original concentration of CS extracts in the scaffolds. Especially for the HUVECs, P01/CS 1/4 group showed statistically significant enhancement in cell proliferation as compared with P01 group. Therefore, P01/CS 1/4 was chosen to evaluate the morphology and distribution of cells on it in comparison with the non-crosslinked pcECM and P01 without CS loading.
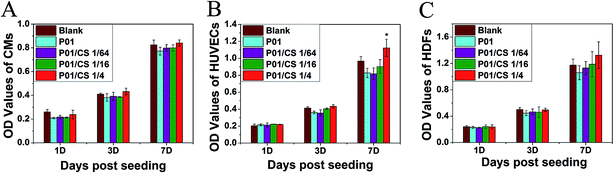 |
| Fig. 7 The CCK-8 assay of H9c2 CMs (A), HUVECs (B) and HDFs (C) seeded on CS-loaded pcECMs. * represents significant difference (p < 0.05) compared with P01 group. | |
Fig. 8 illustrated the morphology of H9c2 CMs, HUVECs and HDFs adhering on aforementioned scaffolds after incubation for 24 h. The CMs developed spindle-shaped cell morphology on non-crosslinked pcECM, while spread better on the samples crosslinked by PC. The HUVECs and HDFs displayed a positive adhesion on all the groups, and HDFs showed fiber-shaped morphology. Live/dead assays (Fig. 9) showed that all the samples seeded with cells exhibited a uniform cell distribution after 7 days of incubation, indicating the great cytocompatibility of the decellularized pcECM both before and after PC crosslinking and CS incorporation.
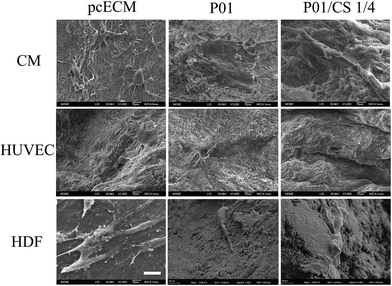 |
| Fig. 8 SEM images of pcECM, P01 and P01/CS 1/4 samples seeded with H9c2 CMs, HUVECs and HDFs after incubated for 24 h. Scale bar indicates 20 μm. | |
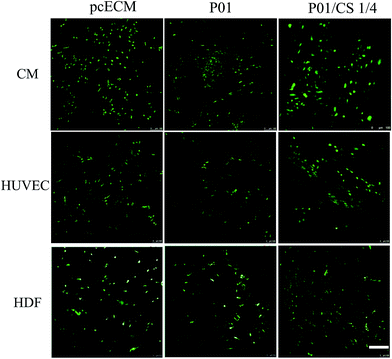 |
| Fig. 9 Live/dead images of pcECM, P01 and P01/CS 1/4 samples seeded with H9c2 CMs, HUVECs and HDFs after incubated for 7 days. Scale bar indicates 200 μm. | |
8. Expression of VEGF and KDR in HUVECs
After 72 h of incubation in the ion extracts of P01 and P01/CS 1/4 samples, the expression of VEGF and KDR in HUVECs were measured by QRT-PCR. As shown in Fig. 10, the gene expressions of VEGF and its receptor KDR in the HUVECs cultured in the ion extracts of P01/CS 1/4 group were about four and two times higher respectively than those in the control medium (Blank) or in the ion extracts of P01 group. The up-regulation of angiogenic growth factors might result from the released Ca and Si ions in the extracts from the P01/CS 1/4 group, indicating that the CS incorporation may play a key role in the angiogenic activity of the myocardial scaffold.
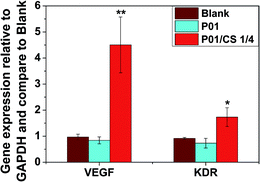 |
| Fig. 10 Expression of VEGF and KDR from HUVECs cultured with control medium and ion extracts from P01 and P01/CS 1/4 groups for 72 h. The data for the gene expression were quantified relative to the same gene expression in HUVECs cultured with control medium. * and ** represent significant difference (p < 0.05 and p < 0.01, respectively) compared with P01 group. | |
Discussion
Fabrication of decellularized myocardial grafts or patches for cardiac repair is currently limited by several obstacles, including lack of vascularization and impaired ECM structure, which will result in the unmatched mechanical properties and increased susceptibility to enzymatic proteolysis.38–40 Several studies have focused on exploring efficient decellularization protocols for the isolation of ECM from myocardium tissues,11,20,41–43 but it is a big challenge to balance the complete acellularization against the well-preserved ECM composition, structure and stability. In this study, for the first time, we have demonstrated the utility of PC crosslinking and CS incorporation to engineer a bioactive decellularized porcine cardiac ECM scaffold. The CS-loaded pcECMs, which were crosslinked by PC, displayed great stability, improved mechanical properties and resistance to enzymatic proteolysis as compared with non-crosslinked one, and meanwhile stimulated the proliferation of HUVECs.
In this study, the tensile strength and elastic modulus of decellularized pcECM decreased as compared with native myocardium, due to the loss of cardiomyocytes which are responsible for part contractions.44 Similar observations were found by Yuval et al.,21 who obtained decellularized pcECM based on a combination of hyper–hypotonic washes, enzymatic digestion, and Triton-X-100 treatment. Therefore, crosslinking is necessary for the matrix to regain the proper mechanical property. After crosslinking by PC, the tensile strength and elastic modulus of pcECMs increased with the increase of PC concentration. When the concentration of PC was lower than 0.1 mg ml−1, the elastic modulus was at a low level, meaning that those crosslinked groups possessed a soft and stretched state. Interestingly, samples crosslinked with 0.05 or 0.1 mg ml−1 PC had the similar mechanical parameters to that of native myocardium, indicating that those samples are mechanically matched and had the potential for in situ myocardial repair. The results of in vitro enzymatic degradation showed that PC crosslinking could significantly enhance the resistance to proteolysis of samples, further confirming that PC-crosslinked pcECMs were chemically stable. And this enhancement was depended on the PC concentration, indicating that the degradation of pcECM could be regulated by changing the amount of original PC reagents. This controllable degradation rate of scaffolds may have significance in cardiac tissue engineering application, which requires further in vivo investigation.
The concentration of PC used in this study was chosen less than 1 mg ml−1, since over-crosslinking may cause an increase in the stiffness of the matrix, and the low concentration of PC might be propitious to the maintaining of the soft state. Previous studies have demonstrated that the crosslinking process involves reaction between the phenolic hydroxyl of PC and the matrix protein amide carbonyl forming hydrogen bond.28,45 Compared with other crosslinking reagents forming covalent bond,22,24 PC crosslinking is weaker but more widely distributed. After crosslinking, PC can release from the samples under continuous shaking in PBS due to the relatively weak hydrogen bond, causing crosslinking instability.29 But in this study, the concentration of PC in all the crosslinked groups was no more than 1 mg ml−1, which was much less than that used in other studies.29,46 Therefore, PC could react thoroughly with the excessive collagen matrix, resulting in small amount PC release and low releasing rate, while good crosslinking efficiency was achieved (Fig. 2). Moreover, the improved mechanical properties and resistance to enzymatic proteolysis of the PC-crosslinked pcECM also confirmed the effectiveness of the low concentration PC crosslinking.
To assess the potential of PC-crosslinked pcECM serving as a cytocompatible scaffold for cardiac tissue engineering, we studied the viability of H9c2 CMs, HUVECs and HDFs on the matrices. These types of cells were chosen as they had been commonly used in different studies in cardiac tissue engineering.42,47,48 Our study showed that all the cells could adhere and propagate well on non- and PC-crosslinked pcECMs. No obvious difference was found on cell viability between the non- and PC-crosslinked groups during the time period tested (Fig. 5, 8 and 9), indicating that the crosslinking of pcECM by PC did not cause cytotoxity. It is known that PC is naturally derived polyphenols, and was reported to be 100 times less cytotoxic to valve interstitial cells as compared with GA.29 Here we confirmed that PC in the concentration range used in the present study was safe for CMs, HUVECs and HDFs.
One of the innovative points of the present study is the method for preparation of bioactive decellularized myocardial matrix using CS extracts interpenetration approach. Traditional way to prepare bioceramic/polymer composites is to suspend ceramic powders into polymer solution. Leu and Leach49 have obtained bioactive glass (BG)/collagen sponges by mixing the collagen solutions with glass particulates and allowing them to gel. However, this method was improper to be used into the preparation of decellularized myocardial scaffolds, since they may impair the ECM structure by mechanical stirring or not allow the bioceramic to penetrate into the center of the matrix by the coating method. In the present study, we utilized the CS extract solutions, which were abundant with Ca and Si ions, as the propagation medium to interpenetrate throughout the matrix and finally formed composite pcECM scaffold with homogeneous distributed CS component within the decellularized matrix after lyophilization. This bioceramic extracts interpenetration method has the advantage of gentle conditions, and easy to perform, which may be applied to all kind of decellularized tissues for preparation of bioactive tissue derived composites materials for tissue regeneration applications.
The amount of incorporated CS was the key point to endue the pcECM with the angiogenic activity. Li et al. have reported that CS extracts diluted at 1/64 and 1/256 could stimulate the proliferation of HUVECs, up-regulated the expression of VEGF and bFGF, and enhance the NO expression from HUVECs.18 In this study, the concentration of ions released from our scaffolds during the first day was 2.8 to 7.5 ppm for Ca ion and 0.9 to 3.7 ppm for Si ion respectively, which was in the similar range of the effective concentrations as compared with previous studies (1.8 and 0.7 ppm of Si ion for CS 1/64 and CS 1/256 respectively).18 In addition, the release rate of ions during 7 days was steady, meaning that the CS-loaded pcECMs would have continuing effect on cell growth and metabolic activity. And this was verified by the cytotoxity results that the CS-loaded scaffolds enhanced the proliferation of the three types of cells (Fig. 7), among which the HUVECs showed statistically significant enhancement as compared with pcECM without CS incorporation. The result of gene expression (Fig. 10) also indicated that the released ions from the CS-loaded scaffolds could stimulate the up-regulation of the expression of proangiogenic factor VEGF and its receptor KDR. In addition, the amount of incorporated CS was limited in a low level, and was not high enough to induce the deposition of calcium salts, which was verified by the steady release of Ca ions of the CS-loaded scaffolds (Fig. 6), suggesting that the incorporated CS component may not induce the calcification of the pcECM. Our results suggest that the CS-loaded pcECM crosslinked by PC has bioactivity to enhance angiogenesis and may have great potential for cardiac tissue regeneration.
Conclusions
Our study demonstrated that PC crosslinking and CS incorporation of decellularized pcECM is an effective approach to fabricate bioactive composite myocardial scaffold, with great crosslinking stability, steady ion release activity and cytocompatibility, and evidently improved mechanical properties and resistance to enzymatic proteolysis. The CS extracts interpenetration method was developed for the first time to prepare the patch scaffold with homogeneous distributed CS component. By controlling the concentration of original CS extracts, the concentration of ions released from the scaffolds could be regulated in the effective range for angiogenic stimulation. Especially the P01/CS 1/4 group, which was crosslinked by 0.1 mg ml−1 PC and immersed in CS extracts diluted at 1/4, possessed comparable tensile strength and compliance to native myocardium, and bioactivity to stimulate the proliferation of HUVECs and the up-regulation of proangiogenic factor VEGF and its receptor KDR from the HUVECs. These results suggest that PC crosslinking and CS incorporation may be a novel method for preparation of complex myocardial ECM scaffolds, which may be used for myocardial tissue repair.
Acknowledgements
This work was supported by the National Natural Science Foundation of China (Grant No. 81190132).
References
- W. Rosamond, K. Flegal, G. Friday, K. Furie, A. Go, K. Greenlund, N. Haase, M. Ho, V. Howard, B. Kissela, S. Kittner, D. Lloyd-Jones, M. McDermott, J. Meigs, C. Moy, G. Nichol, C. J. O'Donnell, V. Roger, J. Rumsfeld, P. Sorlie, J. Steinberger, T. Thom, S. Wasserthiel-Smoller, Y. L. Hong and A. H. Assoc, Circulation, 2007, 115, E69–E171 CrossRef PubMed.
- U. Martin and A. Haverich, Eur. J. Cardio. Thorac. Surg., 2014, 45, 216–219 CrossRef PubMed.
- H. Jawad, N. N. Ali, A. R. Lyon, Q. Z. Chen, S. E. Harding and A. R. Boccaccini, J. Tissue Eng. Regener. Med., 2007, 1, 327–342 CrossRef CAS PubMed.
- R. Sharma and R. Raghubir, Stem Cells Dev., 2007, 16, 517–536 CrossRef CAS PubMed.
- M. Roccio, M. J. Goumans, J. P. G. Sluijter and P. A. Doevendans, Panminerva Med., 2008, 50, 19–30 CAS.
- P. A. Lalit, D. J. Hei, A. N. Raval and T. J. Kamp, Circ. Res., 2014, 114, 1328–1345 CrossRef CAS PubMed.
- W. H. Zimmermann, I. Melnychenko and T. Eschenhagen, Biomaterials, 2004, 25, 1639–1647 CrossRef CAS PubMed.
- C. Galvez-Monton, C. Prat-Vidal, S. Roura, C. Soler-Botija and A. Bayes-Genis, Rev. Esp. Cardiol., 2013, 66, 391–399 CrossRef.
- L. L. Y. Chiu and M. Radisic, Curr. Opin. Chem. Eng., 2013, 2, 41–52 CrossRef.
- H. C. Ott, T. S. Matthiesen, S.-K. Goh, L. D. Black, S. M. Kren, T. I. Netoff and D. A. Taylor, Nat. Med., 2008, 14, 213–221 CrossRef CAS PubMed.
- J. M. Wainwright, C. A. Czajka, U. B. Patel, D. O. Freytes, K. Tobita, T. W. Gilbert and S. F. Badylak, Tissue Eng., Part C, 2010, 16, 525–532 CrossRef CAS PubMed.
- K. A. Robinson, J. Li, M. Mathison, A. Redkar, J. Cui, N. A. Chronos, R. G. Matheny and S. F. Badylak, Circulation, 2005, 112, I135–I143 CrossRef PubMed.
- W. H. Zimmermann, Antioxid. Redox Signaling, 2009, 11, 2011–2023 CrossRef CAS PubMed.
- J. A. Kanczler, P. J. Ginty, J. J. A. Barry, N. M. P. Clarke, S. M. Howdle, K. M. Shakesheff and R. O. C. Oreffo, Biomaterials, 2008, 29, 1892–1900 CrossRef CAS PubMed.
- J. Sohier, L. Moroni, C. van Blitterswijk, K. de Groot and J. M. Bezemer, Expert Opin. Drug Delivery, 2008, 5, 543–566 CrossRef CAS PubMed.
- A. A. Gorustovich, J. A. Roether and A. R. Boccaccini, Tissue Eng., Part B, 2010, 16, 199–207 CrossRef CAS PubMed.
- A. Hoppe, N. S. Guldal and A. R. Boccaccini, Biomaterials, 2011, 32, 2757–2774 CrossRef CAS PubMed.
- H. Li and J. Chang, Acta Biomater., 2013, 9, 5379–5389 CrossRef CAS PubMed.
- A. Leu, S. M. Stieger, P. Dayton, K. W. Ferrara and J. K. Leach, Tissue Eng., Part A, 2009, 15, 877–885 CrossRef CAS PubMed.
- B. Wang, A. Borazjani, M. Tahai, A. L. D. Curry, D. T. Simionescu, J. J. Guan, F. To, S. H. Elder and J. Liao, J. Biomed. Mater. Res., Part A, 2010, 94, 1100–1110 Search PubMed.
- Y. Eitan, U. Sarig, N. Dahan and M. Machluf, Tissue Eng., Part C, 2010, 16, 671–683 CrossRef CAS PubMed.
- C. E. Schmidt and J. M. Baier, Biomaterials, 2000, 21, 2215–2231 CrossRef CAS PubMed.
- Y. L. Zhao, Z. G. Zhang, J. L. Wang, P. Yin, Y. Wang, Z. Y. Yin, J. Y. Zhou, G. Xu, Y. Liu, Z. G. Deng, M. C. Zhen, W. G. Cui and Z. C. Liu, J. Mater. Sci.: Mater. Med., 2011, 22, 1407–1417 CrossRef CAS PubMed.
- P. Zilla, D. Bezuidenhout and P. Human, Ann. Thorac. Surg., 2005, 79, 905–910 CrossRef PubMed.
- J. C. Isenburg, D. T. Simionescu and N. R. Vyavahare, Biomaterials, 2005, 26, 1237–1245 CrossRef CAS PubMed.
- G. Aldini, M. Carini, A. Piccoli, G. Rossoni and R. M. Facino, Life Sci., 2003, 73, 2883–2898 CrossRef CAS PubMed.
- X. Wang, W. Zhai, C. Wu, B. Ma, J. Zhang, H. Zhang, Z. Zhu and J. Chang, Acta Biomater., 2015, 16, 81–93 CrossRef CAS PubMed.
- B. Han, J. Jaurequi, B. W. Tang and M. E. Nimni, J. Biomed. Mater. Res., Part A, 2003, 65, 118–124 CrossRef PubMed.
- W. Y. Zhai, J. Chang, K. L. Lin, J. Y. Wang, Q. Zhao and X. N. Sun, Biomaterials, 2006, 27, 3684–3690 CAS.
- H. Y. Li, W. Y. Zhai and J. Chang, J. Biomater. Appl., 2009, 24, 231–246 CrossRef CAS PubMed.
- F. Bolland, S. Korossis, S. P. Wilshaw, E. Ingham, J. Fisher, J. N. Kearney and J. Southgate, Biomaterials, 2007, 28, 1061–1070 CrossRef CAS PubMed.
- M. C. GarciaParrilla, F. J. Heredia, A. M. Troncoso and A. G. Gonzalez, Talanta, 1997, 44, 119–123 CrossRef CAS.
- H. W. Sung, Y. Chang, C. T. Chiu, C. N. Chen and H. C. Liang, Biomaterials, 1999, 20, 1759–1772 CrossRef CAS PubMed.
- X. B. Liu, J. A. Wang, X. Y. Ji, S. P. Yu and L. Wei, Stem Cell Res. Ther., 2014, 5, 111–122 CrossRef PubMed.
- C. Yao, P. Prevel, S. Koch, P. Schenck, E. M. Noah, N. Pallua and G. Steffens, Cells Tissues Organs, 2004, 178, 189–196 CrossRef CAS PubMed.
- L. Bordenave, C. Baquey, R. Bareille, F. Lefebvre, C. Lauroua, V. Guerin, F. Rouais, N. More, C. Vergnes and J. M. Anderson, J. Biomed. Mater. Res., 1993, 27, 1367–1381 CrossRef CAS PubMed.
- J. M. Sorrell, M. A. Baber, L. Brinon, D. A. Carrino, M. Seavolt, D. Asselineau and A. I. Caplan, Exp. Dermatol., 2003, 12, 315–323 CrossRef CAS PubMed.
- P. V. Kochupura, E. U. Azeloglu, D. J. Kelly, S. V. Doronin, S. F. Badylak, I. B. Krukenkamp, I. S. Cohen and G. R. Gaudette, Circulation, 2005, 112, I144–I149 Search PubMed.
- S. F. Badylak, P. V. Kochupura, I. S. Cohen, S. V. Doronin, A. E. Saltman, T. W. Gilbert, D. J. Kelly, R. A. Ignotz and G. R. Gaudette, Cell Transplant., 2006, 15, S29–S40 Search PubMed.
- C. Witzenburg, R. Raghupathy, S. M. Kren, D. A. Taylor and V. H. Barocas, J. Biomech., 2012, 45, 842–849 CrossRef PubMed.
- J. B. Schulte, A. Simionescu and D. T. Simionescu, Tissue Eng., Part C, 2013, 19, 518–530 CrossRef CAS PubMed.
- Y. Wang, T. Bronshtein, U. Sarig, F. Y. C. Boey, S. S. Venkatraman and M. Machluf, Int. J. Biosci., Biochem. Bioinf., 2012, 2, 363 Search PubMed.
- U. Sarig, G. C. T. Au-Yeung, Y. Wang, T. Bronshtein, N. Dahan, F. Y. C. Boey, S. S. Venkatraman and M. Machluf, Tissue Eng., Part A, 2012, 18, 2125–2137 CrossRef CAS PubMed.
- J. W. Holmes, T. K. Borg and J. W. Covell, Annu. Rev. Biomed. Eng., 2005, 7, 223–253 CrossRef CAS PubMed.
- A. B. Dongmo, A. Kamanyi, M. S. Anchang, B. C. A. Nkeh, D. Njamen, T. B. Nguelefack, T. Nole and H. Wagner, J. Ethnopharmacol., 2001, 77, 137–141 CrossRef CAS PubMed.
- W. Zhai, H. Zhang, C. Wu, J. Zhang, X. Sun, H. Zhang, Z. Zhu and J. Chang, J. Biomed. Mater. Res., Part B, 2014, 102, 1190–1198 CrossRef PubMed.
- I. Kutschka, I. Y. Chen, T. Kofidis, T. Arai, G. von Degenfeld, A. Y. Sheikh, S. L. Hendry, J. Pearl, G. Hoyt, R. Sista, P. C. Yang, H. M. Blau, S. S. Gambhir and R. C. Robbins, Circulation, 2006, 114, I167–I173 Search PubMed.
- A. V. Shinde and N. G. Frangogiannis, J. Mol. Cell. Cardiol., 2014, 70, 74–82 CrossRef CAS PubMed.
- A. Leu and J. K. Leach, Pharm. Res., 2008, 25, 1222–1229 CrossRef CAS PubMed.
|
This journal is © The Royal Society of Chemistry 2016 |