DOI:
10.1039/C6RA02674E
(Paper)
RSC Adv., 2016,
6, 32471-32479
Long-term and oxidative-responsive alginate–deferoxamine conjugates with a low toxicity for iron overload
Received
29th January 2016
, Accepted 16th March 2016
First published on 21st March 2016
Abstract
To address the drawbacks of deferoxamine, a clinical drug widely used for iron-overload-related conditions, oxidation-responsive alginate–deferoxamine (Alg–DFO) conjugates were prepared and their structure characterized by size-exclusion chromatography, FTIR, and 1H NMR. The prepared conjugates exhibited long-term stability, with a half-life 9.8–17.8 times longer than that of the free DFO, depending on the molecular weight of the conjugated alginate. On the other hand, the conjugates could quickly respond to oxidative stimuli and be degraded, which suggests they hold the potential to be cleared from the body in an iron-overload-related oxidative environment to avoid their accumulation and to address safety concerns. The degradation mechanism for the oxidative response is proposed. At the same time, it is interesting to find that the conjugates are capable of protecting the iron-binding capacity of the attached DFO from the oxidation environment, which probably is a result of the antioxidant activities of the conjugated alginate. In addition, the conjugates show a lower cytotoxicity in comparison with the free DFO. Overall, it is anticipated that the Alg–DFO conjugates prepared in this work will have the potential to be used for treating iron-overload-related disease.
Introduction
Iron is one of the most important trace metals in the body for normal oxygen transport and metabolic processes, whereas its overloading is involved in numerous diseases, such as thalassemia, stroke, and Alzheimer's and Parkinson's diseases. To treat these conditions, a variety of iron chelators have been developed, in which the most widely used one in clinical practice is deferoxamine (DFO), due to its highly specific binding to iron with the highest stable constant of 1031 for the complex.1–3 However, the wider use of DFO over the past few decades has been significantly hindered by its drawbacks.1,4 First, it has a very short plasma half-life within 30 min in vivo, resulting from its rapid metabolism by the globulin in the blood, and thus it requires administration by intravenous or subcutaneous infusion over 8–12 h per day, 5–7 days per week, which results in very poor patient compliance. Second, the toxicity of DFO has been suggested to be involved in numerous complications, including growth retardation, endocrine dysfunction, cardiomyopathy, and peripheral neuropathies.
Polymer–drug conjugation is a promising strategy to overcome these drawbacks as it effectively improves a drug's long-term stability as well as enabling the drug to be administered at lower dosages and with less frequency, so as to relieve the toxicity.5,6 On the other hand, it is anticipated that the polymer carrier should ideally be degraded and cleared from the body after fulfillment of its role to avoid its accumulation in tissues, which could result in safety concerns. For this purpose, recent research has focused on the design of conjugates with stimuli-responsive properties, where the carriers, on the one hand, shield the drug during circulation and improve the stability, and, on the other hand, degrade at the target upon being triggered by some stimuli present at the local environment.7–11 These stimuli may be exogenous, such as light, a magnetic field, or ultrasound, or endogenous, e.g., pH, temperature, oxidative, or redox potential. Iron overload is closely associated with an oxidative environment since iron plays an important role in catalyzing oxidative reactions that yield highly reactive toxic hydroxyl radicals, leading to oxidative stress and cell death, activating lipid peroxidation, and exacerbating excitotoxicity. As a result, carriers with an oxidative response appear to be potential candidates for DFO conjugation in iron-overload-related environments.
Although there are many reports on oxidation-responsive polymer carriers for drug delivery, these carriers are mainly limited to four families,12 e.g., poly(propylene sulfide), selenium-based polymers, and aryl oxalate- and phenylboronic ester-containing polymers. Moreover, the clinical application of these polymer carriers is still challenging in many aspects, such as biocompatibility, biodegradation, and nontoxicity. The development of new carriers is therefore continuing to attract research interest. For example, a recent study reported that chitosan, a natural polysaccharide, can be used as a potential oxidative-response carrier for drug delivery.13 Alginate is another naturally occurring polysaccharide, composed of α-L-guluronate (G unit) and β-D-mannuronate (M unit) arranged as linear homopolymeric and heteropolymeric blocks, and has been widely used in drug, gene, and cell delivery systems due to its biocompatibility and non-immunogenicity.14–18 Also, it has been reported that alginate conjugation can improve the long-term stability and the solubility of the drugs.19–21 Moreover, like chitosan, alginate is readily degraded under oxidation conditions, such as in an aqueous solution of peroxide hydrogen,22,23 suggesting its possibility to be used as an oxidation-responsive drug carrier as well. However, to the best of our knowledge, there is no report on the design and preparation of oxidation-responsive drug conjugates using alginate or its derivatives as a polymer carrier.
Herein, we explore the possibility of preparing oxidation-responsive alginate–DFO (Alg–DFO) conjugates. As shown in Fig. 1A, we hypothesized that the conjugated alginate, on the one hand, could protect DFO from metabolism by globulin during circulation, thus improving the long-term stability of DFO, and on the other hand, it could offer a degraded response to oxidative stimuli and be cleared from the body. To achieve this goal, a series of alginate in their partially oxidized forms, as alginate dialdehyde (ADA), were prepared, and then the conjugates were formed by a Schiff-base reaction, followed by reduction (Fig. 1B). The structure of the conjugates was characterized by size-exclusion chromatography (SEC), FTIR, and 1H NMR. The stability of the conjugates was evaluated by a metabolism assay. The oxidative response was determined by the change of the molecular weight (MW) of the conjugates in an aqueous solution of H2O2, and the mechanism was proposed. Finally, the cytotoxicity of the conjugates was assessed and quantified using endothelial cells.
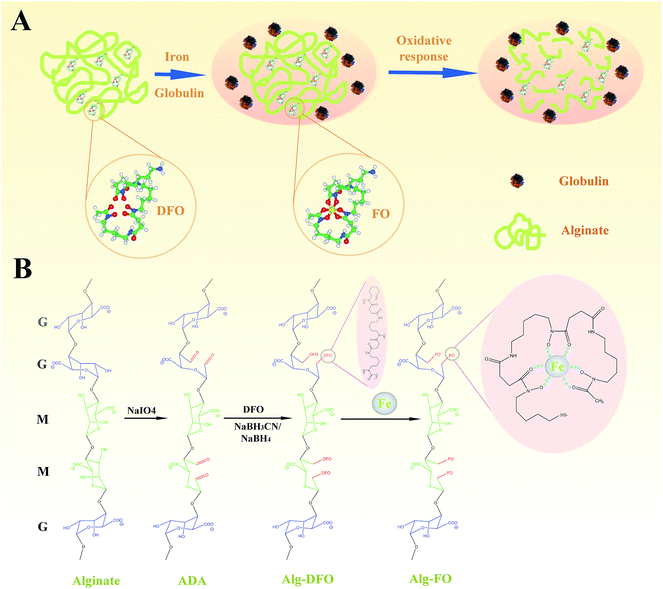 |
| Fig. 1 (A) Schematic of an Alg–DFO conjugate. On the one hand, the attached DFO binds iron while the alginate protects the DFO from metabolism by the globulin in the blood, and on the other hand, it is degraded at the target by the local oxidative environment. (B) Preparation pathway of the Alg–DFO conjugate and iron binding, wherein DFO transforms into feroxamine (FO). | |
Results and discussion
Preparation of Alg–DFO conjugates
As shown in Fig. 1B, Alg–DFO conjugates were prepared by Schiff-base reaction through the terminal amine groups in DFO and reactive aldehyde groups in ADA, followed by reduction with NaBH3CN and NaBH4. The structure characteristics of the prepared ADA and Alg–DFO conjugates are summarized in Table 1. Here, a series of ADA were prepared by the oxidation of alginate with different molar ratios of periodate/uronate units. The degree of oxidation (DO) and MW of the prepared ADA were coupled as the oxidation of alginate with sodium periodate results not only in cleavage of the C2–C3 bond, thus forming reactive aldehyde groups, but also in main chain scission as a simultaneous reaction. Therefore, for the prepared ADA, the higher the DO, the lower the MW. In addition, it was shown that the DO of the ADA was somewhat lower than that of the theoretical value. This is probably due to the formation of hemiacetals, which protects some –OH groups from further oxidation. For the prepared Alg–DFO conjugates, the MW was further decreased compared to that of the corresponding ADA. The reasons for this may be attributed to two aspects. First, the intermolecular hemiacetals formed in the ADA were opened by reduction reactions and thus decrease the MW. Second, the linkages of the oxidized residues are sensitive to hydrolysis,24 which will cause degradation during the preparation process. The DFO contents in the conjugates were increased with the increase of the DO, by 8.7, 14.7, and 20.4% by molar ratio and corresponding to around one DFO per eleven, seven, and five uronate units for Alg–DFO1, Alg–DFO2, and Alg–DFO3, respectively.
Table 1 The structural characteristics of the ADA and conjugates
Product |
Mw app (kDa) |
Mn app (kDa) |
PDI |
DO (%) |
DFO content (%) |
ADA1 |
554 |
108 |
5.11 |
13.1 |
— |
ADA2 |
358 |
74.6 |
4.80 |
26.8 |
— |
ADA3 |
259 |
61.5 |
4.21 |
43.2 |
— |
Alg–DFO1 |
245 |
48.7 |
5.04 |
— |
8.7 |
Alg–DFO2 |
128 |
35.8 |
3.59 |
|
14.7 |
Alg–DFO3 |
62.7 |
17.7 |
3.54 |
— |
20.4 |
FTIR
The FTIR spectra of the alginate sodium, ADA, DFO, and the conjugates are shown in Fig. 2. The alginate sodium exhibited the same spectra as in the literature:25 a broad band at about 3400 cm−1 assigned to O–H stretching vibrations and a weak signal at 2926 cm−1 due to C–H stretching vibrations; two peaks near 1612 and 1417 cm−1 that, respectively, represent asymmetric and symmetric stretching vibrations of the COO–; and bands at 1200–970 cm−1 that are mainly due to C–C and C–O stretching in the pyranoid ring and to C–O–C stretching of glycosidic bonds. In comparison with the spectrum of the alginate sodium, there were newly formed peaks at around 1730 cm−1 in three of the ADA products, which corresponded to the symmetric vibrational band of aldehyde groups and which therefore confirmed the oxidization of the alginate. In addition, the broad band centered around 3400 cm−1 became much narrower, which was probably due to the oxidization of the hydroxyl groups on the vicinal diol of the alginate. For DFO, the two bands at 3313 and 3104 cm−1 are attributed to the N–H stretching vibrations, and the bands at 2931 and 2858 cm−1 are, respectively, assigned to the asymmetric and symmetric stretching vibrations of the CH2. In the low wave-number region, the bands at 1628, 1566, 1460, 1200, and 1053 cm−1 are due to the stretching vibration of the C
O (amine I), the bending vibration of the N–H (amine II), the bending vibration of the CH2, the stretching vibration of the C–C, and the stretching vibration of the N–O of the hydroxamate groups, respectively.
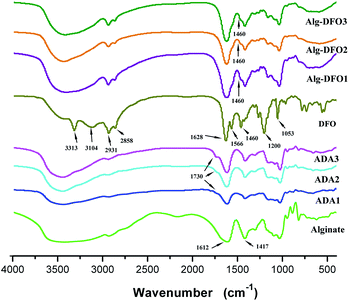 |
| Fig. 2 . FTIR spectra of the alginate sodium, ADA, DFO, and the conjugates. | |
The FTIR spectra of the three conjugates are similar. When comparing these spectra with those of ADA and DFO, it was found that: (1) the band at 1730 cm−1 had disappeared, indicating the formation of the Schiff's base involving the amino groups of DFO and the aldehyde groups of the ADA. The absorption of the amide resulting from the reduction of the Schiff's base was probably overlapped by the band from the amide of DFO and the band of COO– of ADA; (2) although the N–H stretching vibrations at 3313 and 3104 cm−1 in DFO were overlapped by the broad O–H stretching band of the hydroxyl groups in the ADA, relatively strong bands of the CH2 stretching vibrations at 2931 and 2858 cm−1 were observed in the spectra of the conjugates. In addition, the bending vibration of the CH2 at 1460 cm−1 in DFO was also observed in the conjugates. These results confirmed that the DFO was successfully conjugated to the molecular chains of the ADA.
1H NMR
1H NMR measurements were performed in order to further confirm the structure of the conjugates. The spectrum of the alginate sodium is shown in Fig. 3A, in which the signals of the anomeric protons of G-1, M-1, and G-5 appeared at the range of 4.2–4.9 ppm, while the other signals at 3.6–4.1 ppm are assigned to the protons of G-2, G-3, G-4, M-2, M-3, M-4, and M-5. The spectra of the three ADA (Fig. 3B) show some differences with that of the alginate sodium. The intensity of the peak at 3.6 ppm corresponding to the signals of protons of G-4, M-3, and M-5 was decreased, which was probably due to change of surroundings caused by oxidation and the breakage of the C2–C3 linkage. The new signal at 4.2 ppm was attributed to the protons of G-5 of the oxidized G units. In addition, there were two new signals at 5.3 and 5.5 ppm, which correspond to the protons of hemiacetals formed from aldehyde and hydroxyl groups.26 The 1H NMR spectrum of the DFO is shown in Fig. 3C. The signals at 2.0 and 2.8 ppm are, respectively, assigned to the protons of –CH3 groups and CH3SO3H. The signals at 1.2–1.6 ppm are assigned to H-2, H-3, H-4, H-9, H-10, H-11, H-16, H-17, and H-18 of the DFO. In the low field, the signals at 2.4–3.5 ppm are assigned to H-1, H-5, H-6, H-7, H-8, H-12, H-13, H-14, H-15, and H-19 of the DFO.27
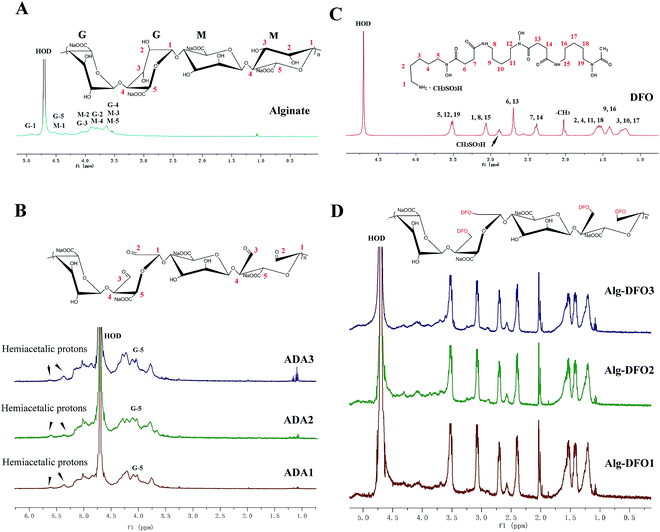 |
| Fig. 3 1H NMR spectra of the alginate sodium (A), ADA (B), DFO (C), and Alg–DFO (D). | |
In comparison with the spectra of the oxidized alginate and DFO, the spectra of the conjugates exhibit two regions, that is, the signals at 1.2–3.5 ppm assigned to the protons of the DFO, and the signals at 3.6–4.9 assigned to the protons of the uronate units of the alginate. In our study, to purify the conjugates, 1 M NaCl was used in the dialysis process to increase the ironic strength, so as to shield the electrostatic interaction between the carboxylic group of the oxidized alginate and the amino group of DFO. Therefore, the 1H NMR together with the FTIR results indicate that the DFO has been covalently linked to the oxidized alginate.
Stability of the conjugates
One of the major drawbacks of DFO is its rapid metabolism by the globulin in the blood and the loss of its ability to bind iron. Thus, a metabolism study was performed to investigate whether the conjugation of DFO to alginate and the variation in the MW of the conjugates could influence the stability to bind iron and provide sufficient protection against metabolism by the globulin. As shown in Fig. 4A–D, all the Alg–DFO conjugates demonstrated a similar absorption maximum at 429 nm to free DFO, suggesting that the iron-binding characteristic of DFO was not compromised by conjugation to alginate. For the free DFO, with the incubation time prolonged, the absorbance was quickly decreased to more than 50% in 1 h, indicating rapid metabolism. In contrast, the conjugates exhibited much longer stability than that of the free DFO. After incubation for 9 h, the absorbance decreased to 57.6%, 46.1%, and 42.7%, respectively, for Alg–DFO1, Alg–DFO2, and Alg–DFO3. The time course of the normalized remaining DFO is shown in Fig. 4E and the in vitro half-life was obtained by the fitting curve. The free DFO had a short half-life of 0.8 h, while the half-lives of the Alg–DFO1, Alg–DFO2, and Alg–DFO3 were, respectively, 14.2, 11.4 and 7.8 h, which were approximately 17.8, 14.3, and 9.8 times longer than that of the free DFO.
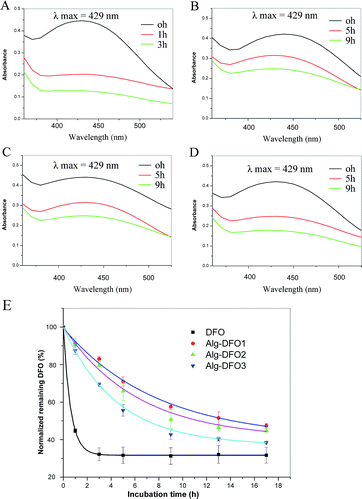 |
| Fig. 4 UV-Visible spectra of the free DFO (A), Alg–DFO1 (B), Alg–DFO2 (C) and Alg–DFO3 (D) incubation in metabolism solution at the setting time points. (E) The time course of the normalized remaining DFO content in the conjugates (error bars represent mean ± SD for n = 3). | |
Alginate is one of the hydrophilic polymers that possesses many hydroxyl and carboxylic groups along the chains. These hydrophilic characteristics can effectively resist the adsorption of proteins such as enzymes, and therefore decrease the degradation of the attached drugs. For instance, alginate–cisplatin conjugates showed a significant decrease of plasma clearance when compared with the free cisplatin.20 Similarly, our results also showed a resistance to metabolization and a prolonged half-life for the Alg–DFO conjugates, which indicated that the conjugation of DFO to alginate was an effective approach to increase the stability of the DFO. In addition, it was shown that the higher the MW of the conjugate, the longer the half-life, which is consistent with previous reports on the effect of conjugate MW on plasma half-life.28
Oxidative response of the conjugates
The oxidative response was studied by checking the MW change of the conjugate after incubation in an oxidative environment of 0.1% H2O2 at 37 °C. As shown in Fig. 5A, all the conjugates exhibited a similar tendency for MW change where the Mw app decreased rapidly from the initial 245, 128, and 62.7 kDa to 22.8, 14.3, and 10.8 kDa at 30 min, and then to 8.8, 7.8, and 6.5 kDa at 60 min for Alg–DFO1, Alg–DFO2, and Alg–DFO3, respectively. Here, a significant reduction in Mw app was observed, which indicated that the conjugates could be degraded in response to an oxidative stimulus, and the resulting degradation fragments would be expected to be cleared from the body easily. Meanwhile, the stability of the conjugates and the free DFO in the oxidative environment were also determined by their iron-binding capacity, and the results are shown in Fig. 5B. The iron-binding capacity of the free DFO was seriously weakened by the oxidative environment, with a remaining DFO content of around 80% after incubation for 3 h. In contrast, the remaining DFO of the conjugates was significantly higher than that of the free DFO (p < 0.05), suggesting that the conjugated alginate could protect the DFO from oxidation. Indeed, alginate molecular chains possess antioxidant activities in terms of a radical scavenging capability through both hydrogen abstraction and radical addition.29
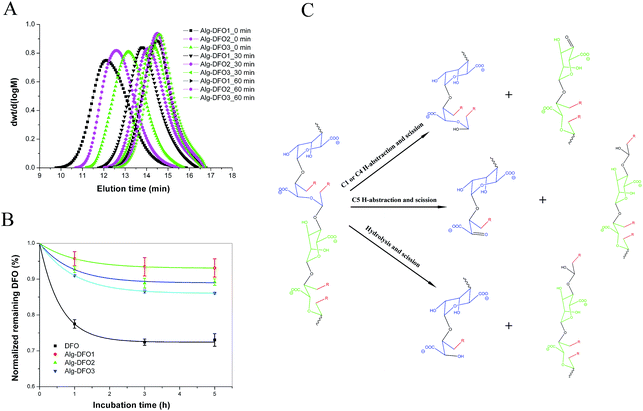 |
| Fig. 5 (A) Time course of the Mw app of the conjugates incubation in 0.1% H2O2. (B) Time course of the normalized remaining DFO in the conjugates in 0.1% H2O2 (error bars represent mean ± SD for n = 3). (C) Proposed degradation mechanism of the conjugates. | |
In this work, we explored the possibility of using alginate in its partially oxidized form as an oxidation-responsive carrier for DFO conjugation and delivery, and the results showed that the conjugates could quickly respond to oxidative stimuli, with a decrease of more than 90% of Mw app after 60 min incubation. Compared to the degradation of alginate with H2O2, the conjugates in this study exhibited faster degradation. To explain this phenomenon, a mechanism was proposed (Fig. 5C). First, naturally occurring polysaccharides, such as alginate and chitosan, usually contain traces of metal ions, which would readily catalyze the decomposition of hydrogen peroxide to form highly reactive hydroxyl radicals via a Fenton reaction.22,30 The formed hydroxyl radicals are capable of abstracting hydrogen atoms of the polysaccharides and then rearranging their structure, with scission of the linkages. Thus, free-radical-induced degradation may play an important role in the oxidative response of the conjugates. That is, H-abstraction, on the one hand, from C-1 or C-4 on the conjugated alginate residues, like other polysaccharides, would lead to scission of the glycosidic bonds,23 while on the other hand, H-abstraction from C-5 on the oxidized residues would also lead to scission of the chains because of the C2–C3 cleavage and ring opening, of which the latter, in particular, is an additional feature of the conjugates and will cause more chain scission. Besides the free radical-induced degradation, the conjugates are capable of hydrolysis due to the oxidized residues,24 although the degradation rate is slow.
Cytotoxicity
DFO has been studied to be cytotoxic in a dose-dependent manner. In order to investigate whether Alg–DFO conjugates can reduce the toxicity of free DFO, the cell viability of HUVECs incubation with different concentrations of the conjugates for 48 h was evaluated by MTT assay. The results are shown in Fig. 6A. For the free DFO group, a significant decrease in the viability of the cells was shown when the concentration was in the range of 0.005–0.5 mM, with a viability of 48.6–85.2%. In comparison, all the conjugate groups exhibited viabilities greater than 90% in the equivalent DFO concentration of 0.005–0.05 mM. When the concentration was further increased, the viabilities decreased from 90.0%, 92.4%, and 92.0% to 54.9%, 57.3%, and 61.7% for Alg–DFO1, Alg–DFO2, and Alg–DFO3, respectively. At every corresponding equivalent DFO concentration, the cell viability of the conjugate group was significantly higher than that of the free DFO group. These results indicated that the cytotoxicity of the conjugates was reduced.
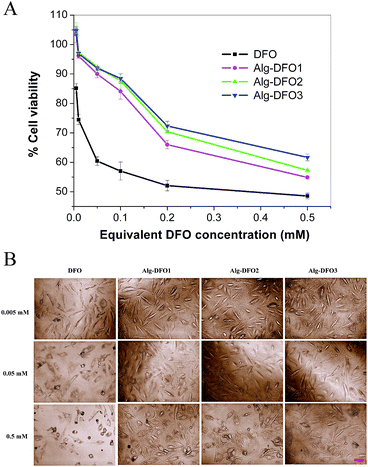 |
| Fig. 6 (A) Cell viability of HUVECs in the presence of the conjugates and the free DFO (error bars represent mean ± SD for n = 3). (B) Cell morphology of HUVECs in the presence of the conjugates and the free DFO on day 2, scale bar 50 μm. | |
The cell morphology was studied by phase contrast microscopy. As shown in Fig. 6B, the cells showed a normal spindle shape in all the groups at low concentrations. However, more cells with a round shape were observed and more and more cells died in the free DFO group when the concentration was increased. On the contrary, more cells kept spreading and less cells died in the conjugate groups. These results are consistent with the above MTT assay, which confirmed the reduced cytotoxicity for the conjugate groups. The cytotoxicity of DFO has been suggested to result from the ability of DFO molecules to diffuse into cells and chelate intracellular iron stores,31 which results in a lack of this essential trace metal and thus adversely affects the cells viability. When DFO conjugates to alginate, the molecular size is significantly larger than that of the free DFO, which makes it more difficult to diffuse into the cells and this therefore reduces the cytotoxicity.
Experimental
Materials
Sodium alginate (viscosity ≥ 2000 cps, M/G 1.96, Sigma code A-2033), deferoxamine mesylate salt (DFO), glutaraldehyde, and diphenyl tetrazolium bromide (MTT) were obtained from Sigma-Aldrich (St. Louis, MO, USA). Sodium cyanoborohydride (NaBH3CN) and sodium borohydride (NaBH4) were purchased from Aladdin Co. (Shanghai, China). Fetal bovine serum, DMEM, trypsin, penicillin, and streptomycin were purchased from Gibco (Grand Island, NY, USA). Sodium periodate, peroxide hydrogen, and the other reagents (analytical grade) were obtained from Kelong Co. (Chengdu, China).
Preparation of ADA
ADA was prepared according to previous reports with minor modification.32 In brief, 5.0 g of alginate sodium (25 mmol of uronate units) was dissolved in 350 ml deionized water and 50 ml ethanol, and then different ratios of sodium periodate to uronate units (15, 30, 50 mol%) were used. For each reaction, the solution was stirred for 6 h in the dark at room temperature before 10 ml ethylene glycol was added to quench the reaction and the mixture was stirred for another 2 h. Each prepared ADA was separated and purified by precipitation with the addition of NaCl (5.0 g) and ethanol (1000 ml). The precipitant was re-dissolved in about 200 ml deionized water and precipitated with 1000 ml ethanol again, and the process was repeated three times. To further purify the product, the precipitant was re-dissolved in about 200 ml deionized water and then dialyzed using a dialysis tube (MWCO, 3500) against deionized water, with several changes of water until the dialyzate was periodate free. Finally, the solution was lyophilized to obtain the product. The products prepared from 15, 30, and 50 mol% of periodate/uronate units are referred to herein as ADA1, ADA2, and ADA3, respectively.
The chemical structure of the ADA was characterized by SEC, FTIR, and 1H NMR. The DO was determined by potentiometric titration of aldehyde groups by the hydroxylamine hydrochloride/sodium hydroxide method.32 The DO value was calculated by the following eqn (1).
|
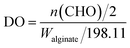 | (1) |
Preparation of Alg–DFO conjugates
Each ADA (0.5 g) was dissolved with deionized water (30 ml) at room temperature under stirring, and then DFO (1.2 g) was added to the solution with stirring. After 2 h, 0.25 g of NaBH3CN, previously dissolved with 2 ml of deionized water, was slowly added to the reaction solution and stirred at room temperature for 4 h, and then 0.5 g of NaBH4 was added to the solution similar to the process for addition of the NaBH3CN. After 24 h, the reaction solution was dialyzed using a dialysis tube (MWCO, 3500) against 1 M NaCl for 3 days, followed by dialysis against deionized water for 3 days. Finally, the solution was lyophilized to obtain the conjugate. The conjugates prepared from ADA1, ADA2, and ADA3 were marked as Alg–DFO1, Alg–DFO2, and Alg–DFO3, respectively.
The chemical structure of the conjugate was characterized by SEC, FTIR, and 1H NMR. The amount of DFO in the conjugate was determined by conversion to the iron-saturated complex, ferrioxamine, using UV-Vis spectroscopy33 (Analytik Jena Scandrop 100, Germany). Briefly, 2.5 mg of conjugate was dissolved in ferrous sulfate solution (2.5 ml, ∼10.0 mM) and was left to stand overnight at room temperature. The absorbance at 429 nm was measured and the DFO concentration was calculated using a molar absorptivity for ferrioxamine of 2300 M−l cm−1. The degree of DFO (% DFO) incorporation was calculated and described as moles of DFO attached per uronate residue.
Characterization
The weight average apparent molecular weight (Mw app), number average apparent molecular weight (Mn app), and polydispersity index (PDI) of the products were determined by a SEC equipped with a Waters 515 HPLC pump, a Waters 2410 refractive index detector, and a Waters hydrogel linear column operated at a flow rate of 0.6 ml min−1. Each sample was eluted with a solution of 0.2 M NaNO3. Dextranum standards were used for column calibration and as a relative reference for the molecular weight calculations. All the data were collected and analyzed using Empower software.
Samples were ground with KBr powder and compressed into pellets for FTIR determination. The spectra of the samples were recorded as % transmittance using a Nicolet 670 FTIR Spectrometer (USA).
1H NMR analyses were recorded on a Bruker AV II-400 MHz spectrometer at 298 K. 10 mg of each sample was dissolved in 1 ml of D2O.
Metabolism of the conjugates and half-life
Metabolism studies were performed to investigate the stability of the conjugate relative to that of free DFO. The content of DFO was determined by converting it with trivalent iron into a brownish-red complex FO and then measured by spectroscopy. For plasma preparation, male 8 week-old Sprague Dawley rats were used as experimental animals. The protocol was approved by the Animal Ethical Committee of the Sichuan University and Chinese national guidelines for the care and use of laboratory animals were applied. The rats were anesthetized with pentobarbital (45 mg kg−1, intraperitoneal). Blood was collected by cardiac puncture following termination by CO2 inhalation. Plasma was immediately separated by centrifugation at 2000 rpm for 10 min at 4 °C. Krebs–Ringer bicarbonate (KRB) buffer was prepared by mixing the following ingredients: 2.5 mM CaCl2·2H2O, 2.4 mM MgSO4·7H2O, 1.2 mM KH2PO4, 4.8 mM KCl, 119.0 mM NaCl, 32.5 mM NaHCO3, and 5.6 mM glucose.
The metabolism studies were carried out as per the method described previously with a slight modification.34 In brief, for example, in the case of Alg–DFO2, 9 mg of conjugate was dissolved in 10 ml of KRB buffer following the addition of 2 ml of plasma. The solution was vortexed and then incubated at 37 °C under 95% O2
:
5% CO2 in a shaker. 1 ml of the incubation solution was pipetted out and deproteinized with 1 ml of 20% trichloroacetic acid at every setting time before the supernatant was collected by centrifugation at 14, 000 rpm for 10 min at 25 °C. The collected supernatant (1 ml) was then mixed with 0.4 ml of ferric chloride solution (0.06%), allowed to stand for 5 min to let the iron complex form, neutralized with 2 N NaOH, 0.4 g of solid sodium chloride added, and then mixed with 1.4 ml of benzyl alcohol, and finally centrifuged to separate the phases. The benzyl alcohol extract was analyzed by UV-Vis spectroscopy at a wavelength of 429 nm determined by a full wavelength scan. The DFO content remaining in the conjugate was calculated by a standard curve established using known quantities of DFO in the same condition except that the plasma was replaced by an equal volume of KBS buffer. The half-life was obtained by fitting the time course of the normalized DFO content remaining in the conjugate. The DFO at equivalent concentration was incubated in the same condition and used as a control.
Oxidative response study
Hydrogen peroxide was added into the conjugate solutions to simulate an oxidative environment and to evaluate the oxidative response of the conjugates. Briefly, 12 mg of conjugate or free DFO was dissolved in 10 ml of deionized water following the addition of 2 ml of 0.6% H2O2. The solution was vortexed and then incubated at 37 °C. Samples of the incubation solution were withdrawn at every setting time and analyzed by SEC. The iron-binding abilities of the conjugates in the oxidative environment were also studied. 1 ml of the incubation solution was withdrawn at every setting time and then mixed with 0.4 ml of ferric chloride solution (0.06%), allowed to stand for 5 min to let the iron complex form, and the absorbance was then determined using a full wavelength scan. The normalized remaining DFO was calculated according to the above description.
Cell culture
Human umbilical vein endothelial cells (HUVECs) obtained from the Type Culture Collection of Chinese Academy of Sciences (Shanghai, China) were used for the cell assay. HUVECs were grown and maintained in DMEM medium supplemented with 10% FBS, 100 μg ml−1 penicillin, and 100 μg ml−1 streptomycin at 37 °C in a 5% CO2 atmosphere, with the medium changed every day until a confluent cell monolayer was formed. The cells in their three passages were used in the experiments.
Preparation of conjugate solutions
Each conjugate and free DFO were dissolved in PBS at a 10 mM DFO equivalent concentration. Completely dissolved solutions were sterilized with 0.2 μm Millipore filters. Prior to the cell culture, each solution was, respectively, diluted to 0.5, 0.2, 0.1, 0.05, 0.01, and 0.005 mM with DMEM medium supplemented with 10% FBS, 100 μg ml−1 penicillin, and 100 μg ml−1 streptomycin. For the control, equal volumes of PBS were added into the same DMEM medium and supplements.
Cytotoxicity evaluation
HUVECs were harvested and seeded in 96-well plates at a density of 3 × 103 cells per well. After the cells attachment, the culture media were replaced with the above conjugate solutions and controls. The cytotoxicity of the conjugates was quantitatively evaluated by cell viability using MTT assay, which is based on the viable cells ability to reduce a tetrazolium-based compound, MTT, to a purplish formazan product. The cells were observed and recorded with a phase contrast microscope (Axio Obeserver D1, Carl Zeiss, Germany) after 48 h, and then the culture media were discarded and rinsed with fresh media three times to remove remaining conjugates or free DFO before adding 20 μl of MTT solution (5 mg ml−1) to each well, and the cells were then incubated at 37 °C for 4 h. After removing the culture media, 150 μl of DMSO was added, and the plates were shaken for 10 min. The optical density of each well was determined using a microplate reader at a wavelength of 490 nm. The normalized viability (%) was expressed by the ratio of the optical density of the conjugate or free DFO to that of control.
Statistical analysis
Quantitative data were presented as the mean ± standard deviation (SD). Statistical analysis was performed using one-way analysis of variance (ANOVA) on SPSS 13.0. A value of p < 0.05 was considered to be statistically significant.
Conclusions
In conclusion, we successfully prepared and characterized oxidation-responsive Alg–DFO conjugates. The conjugates exhibited long-term stability and an oxidative-stimulus respondent degradation as well as protection of the iron-binding capacity. The degradation mechanism was also proposed. In addition, the conjugates showed lower cytotoxicity compared to the free DFO. These results suggest the potential of the conjugates for treating iron-overload-related conditions.
Acknowledgements
This study is supported by the National Natural Science Foundation of China (No. 81401528), China National Funds for Distinguished Young Scientists (No. 51425305), Sichuan Province Science and Technology Support Program (No. 2015SZ0147, 2015SZ0171, and 2014SZ0043), Funds of West China Hospital of Sichuan University (171140322), and 1.3.5 project for disciplines of excellence, West China Hospital, Sichuan University.
Notes and references
- A. V. Hoffbrand, A. Taher and M. D. Cappellini, Blood, 2012, 120, 3657–3669 CrossRef CAS PubMed.
- M. Khalili, M. A. Ebrahimzadeh, M. Kosaryan, A. Abbasi and M. Azadbakht, RSC Adv., 2015, 5, 4804–4810 RSC.
- G. Gautam, E. A. Akam, A. V. Astashkin, J. J. Loughrey and E. Tomat, Chem. Commun., 2015, 51, 5104–5107 RSC.
- M. D. Cappellini and P. Pattoneri, Annu. Rev. Med., 2009, 60, 25–38 CrossRef CAS PubMed.
- H. Xiao, G. T. Noble, J. F. Stefanick, R. Qi, T. Kiziltepe, X. Jing and B. Bilgicer, J. Controlled Release, 2014, 173, 11–17 CrossRef CAS PubMed.
- A. Basu, K. R. Kunduru, E. Abtew and A. J. Domb, Bioconjugate Chem., 2015, 26, 1396–1412 CrossRef CAS PubMed.
- A. Gulzar, S. Gai, P. Yang, C. Li, M. B. Ansari and J. Lin, J. Mater. Chem. B, 2015, 3, 8599–8622 RSC.
- S. Kaur, C. Prasad, B. Balakrishnan and R. Banerjee, Biomater. Sci., 2015, 3, 955–987 RSC.
- J. Wu, L. Zhao, X. Xu, N. Bertrand, W. Choi, B. Yameen, J. Shi, V. Shah, M. Mulvale, J. L. Maclean and O. C. Farokhzad, Angew. Chem., 2015, 127, 9350–9355 CrossRef.
- P. S. Pramod, R. Shah and M. Jayakannan, Nanoscale, 2015, 7, 6636–6652 RSC.
- L. A. Ekanger, M. M. Ali and M. J. Allen, Chem. Commun., 2014, 50, 14835–14838 RSC.
- C. Song, F. Du and Z. Li, J. Mater. Chem. B, 2014, 2, 3413–3426 RSC.
- Y. Xu, L. Wang, Y. Li and C. Wang, Carbohydr. Polym., 2014, 114, 27–35 CrossRef CAS PubMed.
- S. Biswas, M. Chattopadhyay, K. K. Sen and M. K. Saha, Carbohydr. Polym., 2015, 121, 403–410 CrossRef CAS PubMed.
- M. Tian, B. Han, H. Tan and C. You, Carbohydr. Polym., 2014, 112, 502–511 CrossRef CAS PubMed.
- J. Boekhoven, R. H. Zha, F. Tantakitti, E. Zhuang, R. Zandi, C. J. Newcomb and S. I. Stupp, RSC Adv., 2015, 5, 8753–8756 RSC.
- C. Su and F. Cheng, RSC Adv., 2015, 5, 90061–90064 RSC.
- S. Saha, M. U. Chhatbar, P. Mahato, L. Praveen, A. K. Siddhanta and A. Das, Chem. Commun., 2012, 48, 1659–1661 RSC.
- Y. Brudno, E. A. Silva, C. J. Kearney, S. A. Lewin, A. Miller, K. D. Martinick, M. Aizenberg and D. J. Mooney, Proc. Natl. Acad. Sci. U. S. A., 2014, 111, 12722–12727 CrossRef CAS PubMed.
- T. Imai, K. Fujii, S. Shiraishi and M. Otagiri, J. Pharmacol. Sci., 1997, 86, 244–247 CrossRef CAS PubMed.
- S. D. K. Sreenivasan, Carbohydr. Polym., 2014, 99, 499–507 CrossRef PubMed.
- X. Li, A. Xu, H. Xie, W. Yu, W. Xie and X. Ma, Carbohydr. Polym., 2010, 79, 660–664 CrossRef CAS.
- Z. Yang, J. P. Li and H. S. Guan, Carbohydr. Polym., 2004, 58, 115–121 CrossRef CAS.
- I. M. N. Vold, K. A. Kristiansen and B. E. Christensen, Biomacromolecules, 2006, 7, 2136–2146 CrossRef PubMed.
- E. Gómez-Ordóñez and P. Rupérez, Food Hydrocolloids, 2011, 25, 1514–1520 CrossRef.
- C. G. Gomez, M. Rinaudo and M. A. Villar, Carbohydr. Polym., 2007, 67, 296–304 CrossRef CAS.
- B. Borgias, A. D. Hugi and K. N. Raymond, Inorg. Chem., 1989, 28, 3538–3545 CrossRef CAS.
- Y. Wang, S. Hao, Y. Liu, T. Hu, G. Zhang, X. Zhang, Q. Qi, G. Ma and Z. Su, J. Controlled Release, 2010, 145, 306–313 CrossRef CAS PubMed.
- M. Falkeborg, L. Cheong, C. Gianfico, K. M. Sztukiel, K. Kristensen, M. Glasius, X. Xu and Z. Guo, Food Chem., 2014, 164, 185–194 CrossRef CAS PubMed.
- M. Tian, H. Tan, H. Li and C. You, RSC Adv., 2015, 5, 69445–69452 RSC.
- T. Saito and Y. Tabata, Acta Biomater., 2014, 10, 3641–3649 CrossRef CAS PubMed.
- Y. Xu, C. Huang, L. Li, X. Yu, X. Wang, H. Peng, Z. Gu and Y. Wang, Carbohydr. Polym., 2013, 95, 148–154 CrossRef CAS PubMed.
- P. E. Hallaway, J. W. Eaton, S. S. Panter and B. E. Hedlund, Proc. Natl. Acad. Sci. U. S. A., 1989, 86, 10108–10112 CrossRef CAS.
- H. G. Meyer-brunot and H. Keberle, Biochem. Pharmacol., 1967, 16, 527–535 CrossRef CAS PubMed.
Footnote |
† These authors contributed equally to this work. |
|
This journal is © The Royal Society of Chemistry 2016 |