DOI:
10.1039/C6RA02603F
(Paper)
RSC Adv., 2016,
6, 39245-39260
Bacterial siderophore mimicking iron complexes as DNA targeting antimicrobials†
Received
28th January 2016
, Accepted 10th April 2016
First published on 12th April 2016
Abstract
Microbial secretion of siderophores for iron uptake can be employed as an efficient strategy to smuggle in bactericidal agents by conjugation to iron. Three iron complexes: complex 1 [Fe(L1)2]Cl2 L1 = 3-(pyridin-2-yl)dipyrido[3,2-a:2′,3′-c]phenazine (pydppz or ligand 1), complex 2 [Fe(BHA)(L2)Cl]Cl·H20 (BHA = benzohydroxamate) L2 = pyrenyl-dipicolylamine (pydpa or ligand 2) and complex 3 [Fe(BHA)(L3)Cl]Cl·H20 L3 = phenyl-dipicolylamine (phdpa or ligand 3) were synthesized. The ligands were docked for binding to DNA and DNA polymerase I. The antibacterial efficacy of the iron complexes were evaluated against three pathogenic bacteria – Staphylococcus aureus (MRSA), Escherichia coli and Pseudomonas aeruginosa as well as cytotoxicity assessed in C2C12 mouse myoblast cells. In silico docking and molecular dynamic simulations of the ligands revealed stable and non-specific binding to DNA and DNA polymerase I, in the order: L1 > L2 > L3. The bactericidal effect of the iron complexes against MRSA predominantly occurred by bacterial DNA fragmentation as analyzed from gel electrophoresis and comet assay. The extent of DNA damage followed the order: complex 1 > complex 2 > complex 3, in commensurate with docking. Siderophore production elicited preferential bactericidal action of the iron complexes against MRSA. Both, complex 1 and complex 2 were 5–10 fold less toxic in C2C12 cells compared to MRSA. Taken together, a combination of DNA targeting and siderophore mimicking ligands conjugated to iron can be deployed as Trojan horse for the entry of antimicrobials into pathogenic bacteria. Thus, DNA targeting antimicrobials offer a promising solution to persistent bacterial infections. Their selectivity towards microbes can be promoted via siderophore uptake pathways.
1. Introduction
Iron, in its soluble form, is an essential nutrient for most bacteria. Iron is a co-factor for iron–sulphur clusters and haem proteins along with enzymes, like superoxide dismutase, peroxidase, glutamate synthase, and nitrogenase. These enzymes facilitate cellular processes in bacteria, such as oxidative phosphorylation, nitrogen fixation, electron transport and so on.1 Further, iron is a coenzyme or enzyme activator of ribonucleotide reductase, which converts ribonucleotides to deoxyribonucleotidides.2 However, most of the iron in aerobic environments exists as insoluble Fe-oxides or hydroxides. The solubility of ferric iron is 10−17 M at neutral pH, while bacteria require 10−5 to 10−7 M of iron for optimal growth.3 Hence, most pathogenic bacteria secrete high affinity Fe scavenging organic molecules called siderophores, which facilitate iron acquisition by forming soluble iron–chelate complexes. These iron–chelates are actively transported across the cell membrane and such transport is mediated by periplasmic binding proteins and cytoplasmic membrane transporters.4 Fe is a hard Lewis acid and hence most of the chelating siderophore molecules coordinate to ferric ions through Lewis bases with hard donor atoms, like neutral oxygen and nitrogen atoms or negatively charged oxygen. In general, three types of iron coordinating motifs are present in most microbial siderophores. These include hydroxyl groups from catechol moieties like in enterobactin secreted by E. coli, hydroxamate as in coprogen B and citrate derived α-hydroxy carboxylate motifs as in petrobactin or carboxylates in rhiozoferrin.5 The bidentate chelating siderophores have a greater affinity to ferric iron and form more stable hexacoordinate complexes, resulting from a smaller entropy change as compared to monodentate ligands. The secretion of siderophores under iron – deficient conditions is implicated in bacterial virulence and pathogenecity.6,7 Under infection of host by bacterial pathogens, the microbes secrete siderophore molecules and assimilate iron from mammalian iron-binding proteins, such as transferrin and lactoferrin.8
One of the antimicrobial strategies being employed is the synthesis of artificial siderophores conjugated to antibiotics that are up taken by bacteria along with iron–chelates of the antibiotic-conjugated siderophores. Another less explored option is DNA targeting antimicrobials with specificity or selectivity for inducing bacteriotoxicity. The iron–chelates of siderophores can be used as ‘Trojan horse’ to circumvent permeability-mediated drug resistance and selective DNA targeting for bactericidal action against most pathogenic bacteria.9 In the present study, the iron complexes: complex 1 [Fe(L1)2]Cl2 L1 = 3-(pyridin-2-yl)dipyrido[3,2-a:2′,3′-c]phenazine (pydppz or ligand 1), complex 2 [Fe(BHA) (L2)Cl]Cl·H20 (BHA = benzohydroxamate) L2 = pyrenyl-dipicolylamine (pydpa or ligand 2), and complex 3 [Fe(BHA)(L3)Cl]Cl·H20 L3 = phenyl-dipicolylamine (phdpa or ligand 3), are tested for their antibacterial and antibiofilm efficacy against pathogenic Gram-positive bacteria – Staphylococcus aureus (MRSA, USA 300) and Gram-negative bacteria – E. coli K12 (MG 1655) wild type and Pseudomonas aeruginosa. Under similar dosage, the cytotoxicity of the above complexes is assessed in the dosage window of antimicrobial action using C2C12 mouse myoblast cells as a representative non-cancerous cell line. This is in the light of earlier studies reporting comparable photocytotoxicity elicited by identical iron complexes in HeLa cancer cells, MCF-7 breast cancer cells and human keratinocyte cells HaCaT (non-tumorogenic).10,11 One can, therefore, argue that such similar iron complexes could be potent bactericidal agents under non-photodynamic conditions, and presents a strong case for in silico and in vitro investigations of the bacteriotoxicity as envisaged in the current study. Hence, we have employed docking and molecular dynamic simulations to correlate in silico predictions and in vitro bacteriotoxicity results, in a unique approach to corroborate theory and experiments. Further, the therapeutic safety of such metal complexes as antibacterial agents is also assessed by TOPKAT, a computer assisted program that predicts chemical toxicity.12 Summarizing, we have deployed a novel approach via bacterial siderophore mimicking iron complexes as a Trojan horse strategy for bacterial uptake and eliciting bactericidal action via DNA intercalation and fragmentation.
2. Materials and methods
2.1 Ligand exchange of labile iron complexes
The three iron complexes were synthesized following earlier reported protocols.10,11 The detailed synthesis procedures are presented in the ESI† along with the reaction schemes and characterization of the ligands and complexes. Here, the labile nature of the synthesized iron complexes is characterized by ligand exchange reaction with a strong metal chelator 8-hydroxyquinoline (8-HQ).13 Incidentally, 8-HQ has been reported as a potential phytosiderophore.14 It is also known to be a chemical moiety present in quinolobactin, a natural siderophore produced by Pseudomonas fluorescens15 as well as an integral component of oxinobactin and sulfaxinobactin, both synthetic analogues of enterobactin siderophore secreted by E. coli.16 The ligand exchange of the iron complexes with an excess of 8-HQ was studied by monitoring the absorption spectral changes recorded using UV-vis spectrophotometer (Eppendorf). For the study, 2
:
1 equivalents of 8-HQ
:
complex 1 and 1
:
1 equivalents of 8-HQ
:
complex 2/complex 3 in DMSO were mixed and the absorption spectra were recorded immediately and after a time interval. Further absorption spectra of Fe3+
:
8-HQ in 1
:
3, 1
:
2 and 1
:
1 mole ratios were recorded using 0.4 mM of Fe(NO3)3 as the iron precursor. These measurements were made to compare the absorption spectra of the iron complexes after ligand exchange with 8-HQ.
2.2 DNA binding and fragmentation
Virtual screening of potential DNA and/or protein binding drug candidates has become a common and standard technology in modern drug discovery.17 The AutoDock18 and similar softwares have been widely used for virtual screening of such drug molecules. Here, we have used Autodock Vina19 and AutoDock tools17 to calculate and analyze the binding of the ligands of the labile iron complexes with few random nucleic acid sequences, B-DNA Dickerson dodecamer and DNA polymerase I. The molecular coordinates were taken from the Protein Data Bank (PDB; http://www.rcsb.org). Discovery Studio20 was used to visualize and generate graphical images of interactions. The default parameters were used for the molecular docking simulation. Binding energy, number of hydrogen bonds, number of electrostatic interaction and number of hydrophobic interaction in the docking mode with highest score has been taken as a measurement of theoretical binding strength. The experimental investigations of DNA binding and fragmentation were carried out by performing DNA gel electrophoresis and comet/single cell gel electrophoresis (SCGE) assays.
2.2.1 Docking and molecular dynamics simulation with DNA. We have performed docking of ligands from our complexes with a standard representative B-DNA Dickerson dodecamer (PDB ID: 1BNA)21 sequence and some randomly generated DNA sequences of 10 or 15 base pairs (ESI Table S1†). The randomly generated sequences are constructed using Discovery Studio.20 Prior to docking, the ligands were energy minimized in Discovery Studio using CHARm forcefield. The ligands were docked using AutoDock Vina and AutoDock Tools. The results were analyzed using Discovery Studio for calculating the number of interactions in the best binding mode of the ligand with receptor molecule. Top five binding mode energies of docking ligand 1, 2 and 3 to Dickerson dodecamer are tabulated (ESI Table S2†). The binding energy and the number of interactions of the ligands with the receptor DNA are reported. The best binding modes for interaction between Dickerson dodecamer and ligands were subjected to 10 ns of Nanoscale Molecular Dynamics (NAMD) simulation with 2 fs step-size in NVT model with CHARm force field to confirm stable binding of the ligand (ESI Fig. S3†).
2.2.2 Docking and molecular dynamics simulation with DNA polymerase I. DNA polymerase I was obtained from PDB (structure ID: 3EYZ).22 The ligands were docked using AutoDock Vina and AutoDock Tools at the PDB defined binding site of the protein. The best fit model was analyzed using Discovery Studio 4.0 Client and the affinities along with the number of interactions are reported. The best binding modes of ligands with 3EYZ were subjected to 10 ns of NAMD with 2 fs step-size in NVT model with CHARm forcefield to confirm stable binding of the ligand (ESI Fig. S3†).
2.2.3 DNA gel electrophoresis. For analyzing the extent of bacterial DNA fragmentation by the iron complexes, DNA gel electrophoresis of bacterial cell lysates was carried out following the protocol described elsewhere.23 In brief, the bacteria treated with 100 μM of the iron complexes for 4 h were harvested by centrifugation at 12
000 × g for 5 min. The cell pellets were resuspended in 50 μL of lysostaphin (Sigma; 100 μg mL−1) and incubated at 37 °C for 10 min. Subsequently, 50 μL of proteinase K (100 μg mL−1) and 100 μL of 1× TE buffer (10 mM Tris–HCl of pH 8 and 0.1 mM EDTA) were added to each tube and incubated for an additional 10 min at 37 °C. Finally, the bacterial suspensions were placed in boiling water for 5 min to complete the lysis. 50 μL of the lysates were loaded into the wells of a 0.8% agarose gel and the samples were run at 5 V cm−1.
2.2.4 Single cell gel electrophoresis (SCGE)/comet assay. Comet or alkaline SCGE assay was performed to ascertain bacterial DNA strand breaks upon treatment with 10 μM of the iron complexes following the protocol established by Olive.24 In brief, the iron complex treated MRSA were mixed with low melting agarose and applied on a glass slide precoated with 1.5% agarose. Upon solidification of the gel, the embedded bacteria were lysed by overnight incubation of the slides in alkaline lysis buffer (1.2 M NaCl, 100 mM EDTA, 0.1% sodium lauroyl sarcosinate and 0.26 M NaOH) at 4 °C. Subsequently, the slides were carefully washed in ice-cold rinse buffer (0.03 M NaOH and 2 mM Na2EDTA). The slides were subjected to electrophoresis at 0.6 V cm−1, 10 mA for 25 min, removed and immersed in DI water. Finally the slides were stained with propidium iodide (10 μg mL−1) and visualized under fluorescence (Nikon) and Confocal (Carl Zeiss 700) microscopes.
2.3 Antimicrobial and anti-biofilm testing
2.3.1 Bacterial strains and culture protocol. For evaluation of the antimicrobial efficacy of the iron complexes, one Gram positive bacterium – Staphylococcus aureus (MRSA, USA300) and two Gram negative bacteria – E. coli K12 wild type and Pseudomonas aeruginosa were chosen as representative microbes for the study. The freeze dried stocks of the above bacterial species were revived on tryptone soya agar plates. Single colonies of the bacteria were cultured overnight for 10–12 h in 5 mL of Luria broth (LB – 20 g L−1) media and 50 μL was sub-cultured in 4 mL of fresh LB for 2.5 h. The optical density of the seeding bacteria was adjusted to A600 nm = 0.1 (107 to 108 bacteria per mL) and used for the experiments.
2.3.2 Determination of minimum inhibitory concentration (MIC). The minimum inhibitory concentrations (MIC) of the iron complexes were determined by microbroth dilution method in 96-well plates. The purified test compounds were dissolved in DMSO (Merck) to prepare 1 mM of fresh stock solutions. The working solution concentrations of 200, 100, 50, 25 and 12.5 μM were prepared by two-fold serial dilution with LB media. 100 μL of the above working solutions were added to 100 μL of bacterial suspension with A600 nm = 0.1 (107 to 108 bacteria per mL). With the help of a microplate reader (Eppendorf AF2200) equipped with a shaker and thermostat set to 37 °C, the bacterial growth curves were monitored over a period of 18 h, in a real time kinetic cycle with readings taken at 10 min intervals followed by orbital shaking. The minimum concentration at which there was no rise or 99% decline in the growth curves was designated as minimum inhibitory concentration (MIC).
2.3.3 Bacterial viability in mature biofilms. MRSA biofilms were grown in 96 well microtiter plates for a period of 48 h with a media change after 24 h. The mature biofilms were washed with 1× phosphate buffered saline (PBS) and treated with 0, 25, 50 and 100 μM of the iron complexes for 24 h. The bacterial viability in the control and treated biofilms was determined by resazurin dye reduction test, as reported in one of our earlier study.25 Resazurin (7-hydroxy-3H-phenoxazin-3-one 10-oxide) is a blue-colored dye, which is enzymatically reduced to a pink fluorescent molecule, resofurin by viable bacteria. After the treatment of the biofilms with the iron complexes, the media from the wells was aspirated and washed thrice with 1× PBS to remove the planktonic bacteria. Subsequently, 200 μL of fresh LB was added to each well followed by 10 μL of resazurin (0.5 μg per well; Sigma) and incubated for 45 min at 37 °C. With the help of fluorimeter (Eppendorf AF2200) equipped with the dye excitation filter at 535 nm, the emission fluorescence intensity of resofurin was measured at 590 nm.
2.3.4 Total biomass quantification. The total biomass of the biofilms comprising of live/dead bacteria along with the secreted polysaccharide matrix were quantified by staining with 0.1% (w/v) crystal violet.25 The control and treated biofilms were washed with 1× PBS and stained with 200 μL of 0.1% (w/v) crystal violet for 10 min. Subsequently, the biofilms were carefully aspirated to remove the unbound stain and washed with PBS to remove the excess dye. The bound crystal violet stain was then solubilized with 150 μL of 33% acetic acid by incubation for 15 min. From each well, 125 μL of the eluate was used to measure the absorbance of the samples at 600 nm in a multimode plate reader (Eppendorf AF2200).
2.3.5 Fluorescence microscopy of live/dead biofilms. For the live/dead visualization of biofilms, MRSA biofilms were grown on tissue culture glass cover slips. After the experimental duration, the biofilms were washed carefully with 1× PBS and stained by the contents of live/dead Baclight kit (Invitrogen; L7012). The kit contains a green fluorescent dye, Syto9 which binds to the DNA of all bacteria along with polysaccharide matrix while propidium iodide (PI) intercalates with the DNA of membrane compromised cells. Each sample was stained with 500 μL of 1
:
2000 dilutions of the two dyes and incubated for 20 min, as optimized in our earlier studies.26,27 The samples were then washed twice with 1× PBS and were visualized under a fluorescence microscope (Nikon) with an oil immersion lens.
2.3.6 Bacterial siderophore detection assay. The production of bacterial siderophores by MRSA under iron deficient conditions and when supplemented with different concentrations of freely available Fe2+ as well as iron complexes was investigated in planktonic cultures, following the method provided by Neilands et al.7 For the study, the MRSA strain was cultured in normal LB media until the bacteria reached the logarithmic growth phase. Subsequently, the bacteria were harvested by centrifugation at 2400 × g for 5 min and resuspended in iron deficient minimum salt medium 9 (MM9). The recipe and preparation of MM9 media was followed from an earlier work.28 The MM9 media was further supplemented with 0.3% casamino acids and 0.05% glutamic acid as nitrogen source while glucose was the carbon source.The bacteria in the logarithmic phase were cultured for 48 h in iron deficient MM9 media and supplemented with 3.13, 6.25, 12.5 and 25 μM concentrations of Fe2+ and iron complexes in a 96 well microtiter plate. It is understood that these are MIC/sub-MIC concentrations of the iron complexes can elicit siderophore production. After 48 h, the bacteria were centrifuged at 9600 × g and the supernatants were collected. 100 μL of the supernatant was treated with 100 μL of Chrome Azurol S (CAS) assay solution. The recipe and protocol for preparing the CAS assay solution was followed from a previous study.29 The 1
:
1 mixtures of the culture supernatant and CAS assay solution were allowed to equilibrate in dark for 1 h at room temperature. The absorbance of the yellowish brown solution was measured at 630 nm in a multimode plate reader. Also, a wavelength scan of the absorbance spectra in the UV-visible range was performed in order to ascertain the type of Fe–siderophore species.
2.4 Cytotoxicity assessment
2.4.1 C2C12 mouse myoblast culture. The C2C12 mouse myoblast cell line was procured from the National Centre for Biological Sciences, Bangalore, India. The C2C12 cells were revived from cryopreserved stock and grown in complete growth medium containing DMEM (Dulbecco's modified Eagle's medium; Invitrogen), 20% FBS (fetal bovine serum; Invitrogen), 1% antibiotic antimycotic solution (Sigma) and 2 mM L-glutamine (Invitrogen). The cells were maintained in a CO2 incubator (Sanyo, MCO-18AC, USA) at 37 °C, 95% humidity and 5% CO2. Upon reaching 70–80% confluency, the cells were detached using 0.05% trypsin–EDTA and harvested by centrifugation at 425 × g for 5 min. The cells were sub-cultured for further use as required.
2.4.2 Cell viability (MTT) assay. The cytotoxicity of the three iron complexes in C2C12 mouse myoblast cells was assessed by a 96-well microtiter based assay. Around 5000 cells per well were seeded, supplemented with 200 μL of complete media and incubated for 24 h at 37 °C in a CO2 incubator. As performed in the antimicrobial assays, the cells were treated with 25, 50 and 100 μM concentrations of the iron complexes for 24 h and 72 h. After the stipulated time intervals, the media from the control and treated cultures was aspirated and fresh media containing 15% MTT (5 mg mL−1; Sigma) reagent was added. Following incubation with the dye for 4 h, the resultant formazan crystals were solubilized in DMSO and the absorbance was measured at 600 nm using a multimode plate reader (AF2200, Eppendorf).
2.4.3 Cell apoptosis/necrosis analysis by flow cytometry. The mode of cell death caused by the iron complexes in the treated myoblasts was monitored by flow cytometry. The disruption of mitochondrial membrane potential is an indicator of early cell apoptosis. JC-1 is a carbocyanine dye, which is sensitive to mitochondrial membrane potential.30 JC-1 aggregates in the live cells show red fluorescence, while mitochondrial membrane depolarized cells exhibit green fluorescence due to JC-1 monomers in the mitochondria.31 The induction of mitochondrial membrane depolarization by the iron complexes was evaluated using flow cytometry. In a manner similar to the above, the C2C12 cells were treated with 25, 50 and 100 μM concentrations of the iron complexes for 24 h and harvested by centrifugation at 425 × g for 5 min. For flow cytometry, the cell suspensions in PBS were incubated with JC-1 dye (10 μg mL−1) at 37 °C for 10 min. The dead cells (apoptotic/necrotic) cells were determined by staining with PI (10 μg mL−1) and incubation for 5 min. The samples were run on BD FACS Canto II flow cytometry. The data were analyzed using BD FACS Diva Version 6.1.1 software.
2.4.4 TOPKAT predictions. TOPKAT was employed to predict the comparative in vivo toxicity of the iron complex ligands with some of the FDA approved molecules. TOPKAT (TOxicity Prediction by Komputer Assisted Technology) is a computational tool for quantitative structure activity relationship (SAR) based toxicity prediction performed with the help of structural descriptors. The molecule is broken into fragments by the software, along with chemical descriptors such as molecular weight, symmetry of the molecule of interest, and the toxicity is predicted.32 In the present study, we apply TOPKAT to predict the toxicity of our ligands and compare the same in reference to commercially available photodynamic therapy (PDT) drug porfimer (Drug Bank ID: DB00707) and a DNA targeted drug doxorubicin (DB00997). We used the Predictive Toxicology packages provided by Accelrys through Discovery Studio platform and used two models, TOPKAT_rat_inhalational_LC50 and TOPKAT_rat_oral_LD50, to predict the toxicity/safety of our ligands in relation to DB00707 and DB00997.
2.5 Statistical analysis
The statistical analysis for all the presented data was carried out using IBM SPSS Statistics 20 software. All the experiments were carried out in triplicate and the analysis of variance (one way ANOVA) was adopted to determine the statistical significance between the iron complex treated bacteria/cells and the untreated controls. For the data analysis, Tukey and Games–Howell tests were employed to determine the statistical significance at p < 0.05, where p denotes the probability that there is no significant difference between the means.
3. Results
We investigated the DNA binding affinities of the iron complex ligands in silico as well as antibacterial efficacy and cytotoxicity of the labile iron complexes in vitro. The degradation of bacterial DNA by the iron complexes is studied by gel electrophoresis methods. Further, the in vivo toxicity of the iron complexes is predicted by TOPKAT, in comparison to FDA approved drugs. Overall, the utility of in silico methods for predicting chemical and molecular toxicity are demonstrated in the present study.
3.1 Labile behavior of the iron complexes
The molecular structures of the iron complexes and their corresponding ligands are shown in Fig. 1. The labile behavior of the iron complexes is established by ligand exchange reaction with 8-hydroxyquinoline (8-HQ), a strong metal chelator. Following the addition of excess of 8-HQ, an instantaneous color change was observed for both complex 2 and 3. The change, as recorded in the absorption spectra, indicated a rapid ligand exchange reaction. However, in case of complex 1, we observed a continuous decrease in the intensity of the absorption peak in the UV range. The addition of few drops of hydrogen peroxide (H2O2) accelerated the ligand exchange reaction, resulting in a quick change in the absorption spectra (see ESI Fig. S2A†). The ligand exchange reactions of complex 2 and complex 3 with 8-HQ are shown in Fig. S2B and C,† respectively. A clear correlation of the absorption spectra of the iron complexes, following ligand exchange with 8-HQ (Fig. S2A–C†) can be observed by comparison with the UV-visible absorption spectra for iron complexes prepared using ferric iron
:
8-HQ in 1
:
3, 1
:
2 and 1
:
1 mole ratios (Fig. S2D†). The absorption maxima at 450 nm and near 600 nm recorded for complex 1, 2 and 3 after ligand exchange with 8-HQ match well with the ferric iron
:
8-HQ in different mole ratios. The above results qualitatively proves the labile nature of the three iron complexes used further for molecular docking and elucidating the bacterial/cell cytotoxicity, as described in the subsequent sections.
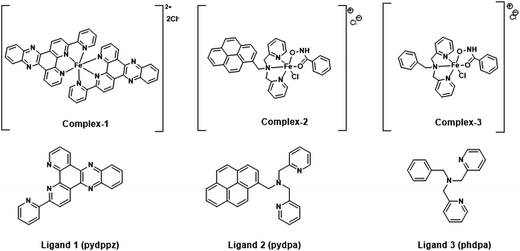 |
| Fig. 1 Molecular structures of the siderophore mimicking iron complexes and associated ligands synthesized for the study. | |
3.2 In silico and in vitro analysis of DNA binding and fragmentation
The docking results of the ligands from the labile iron complexes have been presented in terms of the binding energies and number of interactions between the ligand and the receptor sequence of DNA/protein. The best binding modes and binding stability of the ligands to DNA and DNA Pol I were determined by docking studies and molecular dynamic simulations.
3.2.1 Docking with DNA. The binding energies of Dickerson dodecamer with the ligands 1, 2, 3 (which are ligands associated with complex 1, 2, 3 respectively) are shown in Table 1. The binding energy data in Table 1 lists the order for binding energy of the ligands to the B-DNA Dickerson dodecamer sequence as ligand 1 > ligand 2 > ligand 3. The docking results show the possible modes of binding of the iron complexes in biological systems. The binding energy data (Table 1) show that the ligands from the labile iron complexes can intercalate with cellular DNA, thereby hindering DNA replication and cell multiplication. Similar affinity values generated by docking with random DNA sequences (10–15 basepairs) also exhibit the same trend and those results are presented in ESI Table S1.† Also, the number of different kind of interactions between the ligand and receptor in the optimal binding configuration is an important parameter to predict the goodness of the binding. This is visualized using Discovery Studio. The number and types of receptor–ligand interactions in case of B-DNA Dickerson dodecamer is shown in Table 1. In contrast to the binding energy pattern, the number of ligand receptor interactions presents the following trend: ligand 2 > ligand 3 = ligand 1. This is due to counting of the hydrophobic interactions, which contribute marginally to the overall binding energy. But in complex 1, two molecules of ligand 1 are present in one molecule of iron complex. This implies that upon cellular internalization and degradation of the complex, two molecules of ligand 1 will be released making the effective ligand concentration double compared to the initial molar concentration of the iron complex. To account for this, we can say that the effective number of ligand–DNA interactions upon dissociation of complex 1 is double i.e. 2 × 2 = 4.
Table 1 Binding energies and number of interactions between ligand 1, 2 and 3 with Dickerson dodecamer (DD-DNA) at best binding mode
Ligand |
Binding energy for DD-DNA (1BNA) (kcal mol−1) |
Types and no. of ligand–DNA interactions |
H-bond (conventional) |
Electrostatic |
Hydrophobica |
Total |
Contacts include π–σ hydrophobic contact from DNA and ligand. |
Ligand 1 |
9.9 |
2 |
0 |
0 |
2 |
Ligand 2 |
8.1 |
1 |
1 |
1 |
3 |
Ligand 3 |
6.3 |
2 |
0 |
0 |
2 |
Binding energy is a better parameter to determine the strength of interaction compared to counting number of interactions, because there may be a handful of weak interactions of very low significance, though the total interaction is not very strong. From the best five docking mode energies of ligand 1, 2 and 3 binding to Dickerson dodecamer (shown in ESI Table S2†), we can see the energies are very closely spaced. This suggests that there is no preferential site and the ligands bind to DNA in a non-specific manner. The representative graphical image of interaction between Dickerson dodecamer (PDB ID: 1BNA) and ligand 1 is shown in Fig. 2A(a) while those with ligand 2 and ligand 3 are shown in Fig. S3.† All such observations corroborate well with the fact that the ligands bind to the DNA groove, as experimentally observed by Garai et al.10 Further, the binding constants (Kb) for the intercalation of the iron complexes with calf-thymus DNA (determined by UV-vis absorption titrations) have been listed in Table 5. In the present work, we confirm that the previously reported experimental data10,11 (Table 5) are in excellent agreement with the docking results of specific and randomly designed DNA sequences with the aromatic ligands of the iron complexes (Tables 1 and S1†).
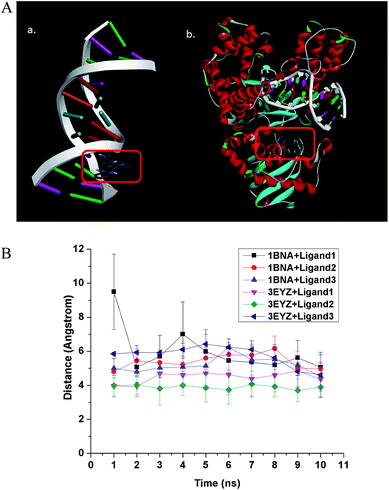 |
| Fig. 2 (A) Interaction of ligand 1 with (a) B-DNA Dickerson dodecamer (1BNA) and (b) DNA Pol I (3EYZ). All the three ligands bind to the minor groove of DNA in a non-specific manner. For DNA Pol I, ligand 1 bind to the active site specifically, ligand 2 exhibits moderate specificity and ligand 3 has a non-specific binding to the active site. The binding is stable in all cases irrespective of the specificity, as confirmed by the molecular dynamics calculations. (B) Average distance between centroid of the ligand and three randomly chosen atoms at the binding site of the receptor molecule has been calculated in an interval of 1 ns of the 10 ns molecular dynamics (MD) calculation. The average distance between centroid of ligand and randomly chosen atoms at the binding site with time has been plotted. It shows stable binding of the ligands to DNA (1BNA)/DNA Pol I (3EYZ). | |
The best ligand binding modes were subjected to 10 ns molecular dynamics simulation to confirm the stability of the complexes. Total energy versus time plot (ESI Fig. S4†) suggests stable binding. We plotted distance between randomly chosen atoms of the ligand and binding site with time, and the plot also indicates stable binding (Fig. 2B). The ligands bind to DNA in a non-specific manner and such binding is stable, as suggested by the molecular dynamics simulation.
3.2.2 Docking with DNA polymerase I. The binding energy of the ligands to DNA Pol I is presented in Table 2. A similar order of ligand affinity to the DNA Pol I enzyme like that towards DNA was observed i.e., as ligand 1 > ligand 2 > ligand 3. Further, the number of interactions for the ligands with DNA Pol I, in the optimal binding configuration is listed in Table 2. Here too, the same phenomenon is observed of ligand 1, as in case of docking with DNA, where we see a different trend for number of interaction: ligand 2 < ligand 1 < ligand 3. Obviously, the hydrogen bonds for ligand 1 appear to contribute more towards binding energy than the electrostatic interactions for ligand 2. Due to this, the binding energy trend diverges from the trend for number of contacts. It can be reiterated here that complex 1 will release two ligand 1 molecules upon cellular uptake, making its effective molar concentration double compared to the intake of the other two complexes. From the best five docking mode (see ESI Table S2†), we can see the best binding energy for ligand 1 with DNA Pol I is > 1 kcal mol−1 than the next best binding candidate, suggesting specific binding of ligand 1 to the active site of the protein. In case of ligand 2 with DNA Pol I, the difference between the best binding energy and the next best candidate is less, but the molecular dynamics simulation (ESI Fig. S4D–F†) suggest tight binding, implying a fairly specific binding. However, in case of ligand 3 with DNA Pol I, the binding energies of all top candidates are close to each other, suggesting non-specific binding of ligand 3 at the active site of DNA Pol I. A representative image showing the interaction of ligand 1 with DNA Pol I is shown in Fig. 2A(b), while those with ligand 2 and ligand 3 are shown in ESI Fig. S3.† Further, ligand 1 has a pyrene ring moiety similar to that of drug molecule 1,2,3-trihydroxy-1,2,3,4-tetrahydrobenzo[A]pyrene (Drug bank ID: DB07435). This molecule is reported to act by binding to DNA polymerase I as one of its target receptor.33 The best binding modes of ligands with 3EYZ were subjected to 10 ns MD simulation to confirm the stability of the complex. Stable binding is confirmed by total energy versus time plot (Fig. S4D–F†). In Fig. 2B, we have plotted average distance between the centroid of the ligand and three randomly chosen atoms of the binding site of receptor molecule in every 1 ns of the 10 ns MD simulation. The plot in Fig. 2B importantly suggests stable binding of the ligands to receptor molecules.
Table 2 Binding energies and number of interactions between ligands 1, 2 and 3 with DNA Pol I at best binding mode
Ligand |
Binding energy for DNA Pol I (3EYZ) (kcal mol−1) |
Types and no. of ligand–DNA Pol I interactions |
H-bond (conventional) |
Electrostatic |
Hydrophobic |
Total |
Ligand 1 |
10.6 |
4 |
2 |
0 |
6 |
Ligand 2 |
9.6 |
2 |
6 |
0 |
8 |
Ligand 3 |
6.4 |
2 |
2 |
0 |
4 |
3.2.3 Bacterial DNA fragmentation. The DNA gel electrophoresis bands of cell lysates from bacteria treated with 100 μM of the iron complexes are shown in Fig. 3B. Beside the DNA ladder in lane 1, distinct bands corresponding to the genomic (∼1000 base pairs) and plasmid DNA (∼800 bp) can be seen in the control (lane 2) and complex 3 (lane 5) treated samples. In lane 3, it is evident that complex 1 caused a near complete degradation of the bacterial DNA leading to the absence of genomic and plasmid DNA bands and the formation of faint band from extra small sized DNA fragments (<50 bp). In lane 4, the genomic and plasmid DNA bands are very faint indicating their susceptibility to degradation. In the lanes 2, 4 and 5, RNA bands can also be seen at close to 50 bp. Overall, complex 1 and 2 induced degradation of complete and partial degradation of genomic and plasmid DNA at a concentration of 100 μM.
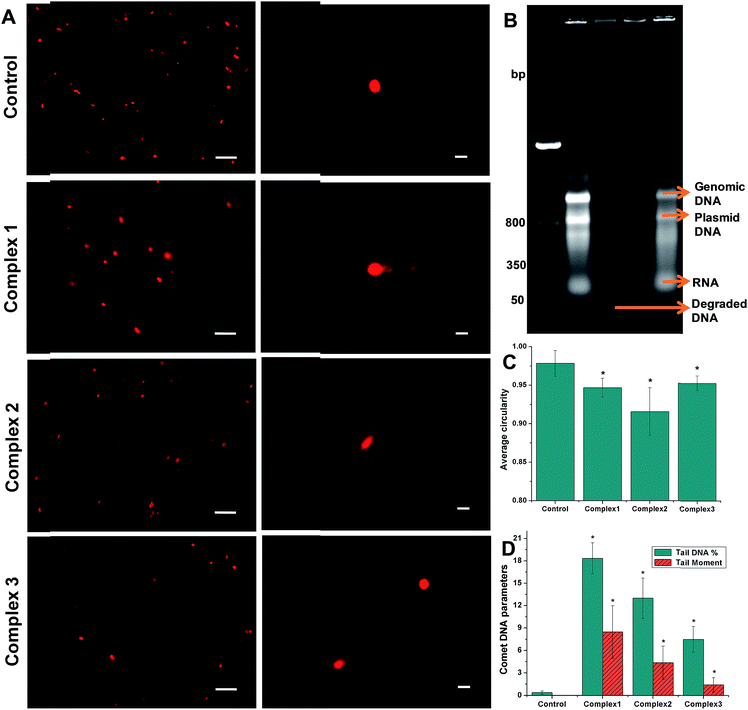 |
| Fig. 3 Representative comet/SCGE images visualized under (A) fluorescence microscope (left column) – scale bar = 10 μm and confocal microscope (right column) – scale bar = 1 μm. (B) DNA gel electrophoresis of bacterial cell lysates showing DNA ladder – lane 1, control – lane 2, complex 1 – lane 3, complex 2 – lane 4 and complex 3 – lane 5; (C) average circularity of the nucleoid of the control and iron complex treated bacteria determined from a minimum of n = 6 images using Image J; (D) comet DNA parameters of the control and iron complex treated bacteria determined using OpenComet plugin in Image J. The data shown are mean ± sd of n ≥ 6 images analyzed from two independent experiments that yielded similar results. * indicate statistically significant difference (p < 0.05) of the iron complex treated samples w.r.t control. | |
Comet assay or SCGE was carried out to ascertain damage to the bacterial genomic DNA by the iron complex ligands at lower dosage of 10 μM. Fig. 3A present low and high magnification images of control and iron complex treated bacterial DNA captured under a fluorescence and confocal microscope respectively. Using the image analysis tools provided by Image J software, the average circularity of bacterial DNA was determined from a minimum of n = 6 images, excluding the blotched signals. It can be clearly seen from Fig. 3C that average circularity of the DNA decreased from spherical in the control towards convex/ellipsoid shape in the iron complex treated samples. Further analysis of the DNA comets was performed with the help of the OpenComet plugin in Image J. An automated scoring and analysis of the comets was carried out following the established guidelines.34 The parameters used to estimate DNA damage were the tail DNA% and tail moment of the comets. Fig. 3D shows that the DNA damage parameters of the iron complex treated bacteria were significantly greater than their respective control parameters. Importantly, the order of DNA strand breakage is commensurate with the order of DNA binding affinities of the iron complex ligands.
3.3 Antimicrobial testing of iron complexes
The iron complexes 1, 2 and 3 were tested against Gram positive S. aureus and Gram-negative E. coli and P. aeruginosa, for their antibacterial and anti-biofilm activity. All the protocols used for the testing of the iron complexes are in consonance with standard microbial practices and methods, as mentioned in the guidelines by the National Committee for Clinical Laboratory Standards (NCCLS).35
3.3.1 MIC assay. The Minimum Inhibitory Concentration (MIC) was determined by continuous monitoring of the bacterial growth curves for a period of 16 h. The minimum concentration at which the exponential growth phase is absent has been designated as the minimum inhibitory concentration. The MIC values for the iron complexes against three bacterial strains are shown in Table 3. From the observation of the growth curve patterns shown in Fig. 4, it is clear that complex 1 is the most potent antibacterial agent among the iron complexes. The antibacterial effect is more pronounced in case of S. aureus, wherein the observed MIC was as low as ∼3 μM. In E. coli, a distinct exponential phase was not observed for any dose of complex 1, leading to a plateau in their growth (Fig. 4). It is probable that these bacteria became dormant and entered into an early stationary phase for survival. For P. aeruginosa too, complex 1 led to an extended lag phase for upto 8 h, which is followed by multiplication of the resistant cells as seen from the exponential growth phase. The detailed growth curves of the three bacterial strains treated with various doses of complex 2 and 3 are presented in the ESI as Fig. S5 and S6,† respectively. The growth curves in Fig. S5† for complex 2 revealed a MIC of 25 μM against MRSA, no inhibition against E. coli and an elongation of the lag phase in P. aeruginosa, recorded for ≥ 6.25 μM. On the contrary, Fig. S6† shows the no growth inhibition by complex 3 for any dosage against the three bacterial strains. It may be noted that we have not assayed for ferrous/ferric ion related bacteriotoxicity. This is in the light of earlier literature reports which indicate negligible toxicity of iron towards bacterial growth. No toxicity was recorded even at concentrations of 20 mg L−1 (350 μM),36 which is 3 times greater than the maximum concentration (100 μM) of the iron complex used in the current study.
Table 3 MIC of the iron complexes against representative bacterial strains
Complex |
MIC (μM) of iron complexes against pathogenic bacteria |
S. aureus (MRSA) |
E. coli |
P. aeruginosa |
Complex 1 |
3.13 |
>100 |
>100 |
Complex 2 |
25 |
>100 |
>100 |
Complex 3 |
>100 |
>100 |
>100 |
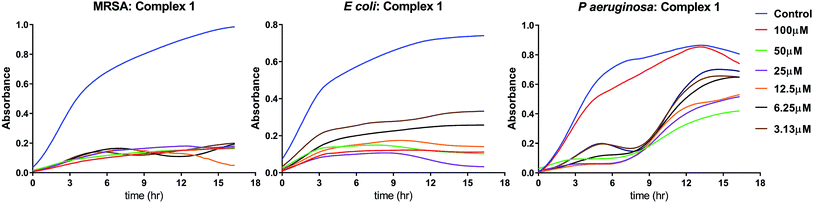 |
| Fig. 4 MIC determination of complex 1 against three representative bacterial strains. For each graph, the X-axis scale corresponds to time (0–18 h) and the Y-axis scale corresponds to optical density (OD @ 600 nm) | |
3.3.2 Anti-biofilm evaluation. Two quantitative biochemical assays were carried out in order to assess the effect of iron complexes on the viability and stability of mature biofilms of S. aureus. The 48 h cultured biofilms in microtiter plates were treated with the iron complexes for 24 h and evaluated by resazurin and crystal violet assays. The resazurin dye reduction test indicates around 80% reduction in the viability of S. aureus biofilms, following treatment with iron complex 1 and 2 at 25–100 μM concentration for 24 h (Fig. 5A). In case of complex 3, a maximum reduction of 50% in bacterial viability was observed at 25–100 μM dosage of the complex. This suggests the higher anti-biofilm efficacy of the complex 1 and 2 in comparison to complex 3, which is commensurate with the molecular docking predictions. On the other hand, the crystal violet assay revealed around 60%, 70% and 50% decrease in the total mass of the biofilms as compared to the control for complex 1, 2 and 3, respectively (Fig. 5B). Fig. 5A and B are the line graphs depicting the anti-biofilm effect of the iron complexes. Further, fluorescence microscopy data show a gradual increase in the percentage of PI stained (dead) bacterial populations with increasing concentration of the iron complexes. Fig. 6 shows representative images of biofilms stained with live/dead Baclight kit after treatment with different doses of the iron complexes. It is evident from the images that both complex 1 and 2 cause destruction of the biofilm and the bacteria exhibit greater planktonic character compared to the dense biofilms in the control. However, in case of complex 3, the biofilm phenotype is persistent even in the treated MRSA biofilms. Nevertheless, the co-localization of Syto9 and PI in the complex 3 treated biofilms indicates partial bacterial injury. Thus, the fluorescence micrographs provide complementary evidence on the reduced viability and stability of the biofilms upon treatment with iron complexes.
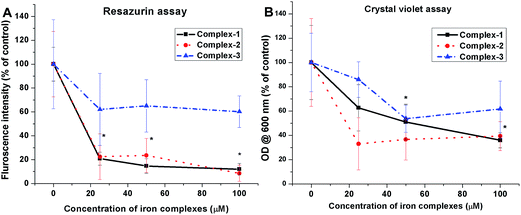 |
| Fig. 5 Anti-biofilm efficacy of iron complexes evaluated by (A) resazurin assay and (B) crystal violet assay. All the experiments were performed in triplicate (n = 3). Data shown are mean ± SD of replicates obtained from three independent experiments that yielded similar results. * indicate statistically significant difference (p < 0.05) of the iron complex treated samples w.r.t control. | |
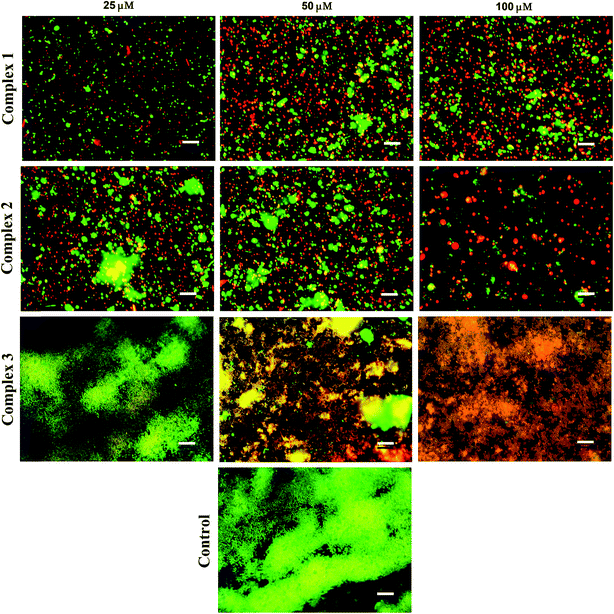 |
| Fig. 6 Representative fluorescence microscopy images of S. aureus (MRSA) stained with live/dead Baclight kit after exposure to different concentrations of the iron complexes. Green represents live bacteria stained by Syto9, while red represents dead bacteria stained with propidium iodide (PI); scale bar = 10 μm. | |
3.3.3 Siderophore production under iron limited culture conditions. The production of bacterial siderophores by MRSA under iron limited culture was assessed by CAS assay. The bacteria in the logarithmic growth phase were treated with sub-MIC doses (3.13, 6.25, 12.5 and 25.0 μM) of the iron complexes for 48 h. Fig. 7A comprises of line graphs, which show the relative production of siderophores, measured as absorbance at 630 nm, for different concentrations of Fe2+ and iron complexes. Upon treatment with Fe2+, the siderophore production increased mildly upto 6.25 μM and was completely absent at 25 μM. In earlier studies, 20 μM has been reported to be the threshold concentration of soluble iron for siderophore production by bacteria.37 In the case of iron complexes, a spike in the biosynthesis of bacterial siderophores can be noted at 3.13 μM dosage, while higher concentrations of the complex appeared to retard or inhibit siderophore production. This may be expected as higher concentrations of iron complexes also inhibit the metabolic activity in bacteria in nutrient deficient cultures. However, the most striking feature of the siderophore production assay is that the iron complexes triggered a much greater synthesis of siderophores at 3.13 μM than the free Fe2+ ions. In summary, the production and transport of bacterial siderophores opens up some interesting prospects for the entry and transport of chemotherapeutic agents into bacteria.
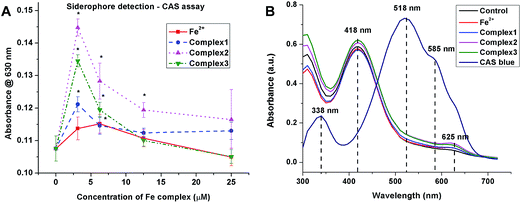 |
| Fig. 7 (A) Staphylococcal siderophore production under iron limiting culture for 48 h determined by CAS assay. All the experiments were performed in triplicate (n = 3). Data shown are mean ± SD of replicates obtained from three independent experiments that yielded similar results. * indicate statistically significant difference (p < 0.05) of the iron complex treated samples w.r.t control. (B) UV-visible absorption spectra of the blue CAS complex and 1 : 1 mixtures of CAS blue and culture supernatants after treatment with iron complexes for 48 h. | |
Furthermore, the UV-visible absorption spectra of the CAS solution and 1
:
1 mixture of culture supernatants and CAS shuttle solutions show marked differences in the absorption maxima (Fig. 7B). The absorption spectrum of the CAS blue assay solution exhibited absorption maxima at 338 nm and 518 nm along with a shoulder peak at 585 nm. In contrast, the 1
:
1 equilibrated mixtures of CAS blue and culture supernatants containing siderophores exhibited absorption maximum at 418 nm, corresponding to Fe–CAS red complex and another low intensity absorption maximum at 625 nm due to Fe–siderophore complex. The UV-visible absorption bands for the three iron complexes (1, 2 and 3) were however not detected. This suggests that the labile iron complexes have apparently undergone complete ligand exchange with the siderophore molecules and transported into the bacterial cells via membrane receptors.
3.4 Cell cytotoxicity assessment
In a manner similar to the treatment of suspension cultures and bacterial biofilms, cell monolayers of C2C12 mouse myoblasts were treated with 25, 50 and 100 μM concentrations of the iron complexes. The results were analyzed using MTT assay and flow cytometry.
3.4.1 MTT assay. The cytotoxicity of the iron complexes against C2C12 mouse myoblasts was determined in terms of their IC50 concentrations, which is the dose at which 50% of the cells are viable. The IC50 values determined after 24 and 72 h of treatment with the iron complexes are summarized in Table 4. The dose-dependent toxicity profiles of the iron complexes against the C2C12 cells have been depicted as line plots in Fig. 8A and B. The complex 1 was the most toxic with IC50 values of 41.3 and 14.5 μM, respectively after 24 and 72 h of culture of C2C12 cells. However, complex 2 and complex 3 exhibited similar levels of cytotoxicity at both the timepoints. At 24 h, the IC50 values were around 60 and 50 μM for complex 2 and complex 3, respectively. The values decreased identically to ∼20 μM for both complexes 2 and 3 after 72 h. Thus, a time-dependent cytotoxicity can be inferred from the myoblast response towards iron complexes. However, it may be worthwhile to note that identical iron complexes were toxic at 2–10 times lower concentration towards bacteria than that of the eukaryotic cells. This presents a therapeutic dosage window for antibacterial application.
Table 4 MTT based cytotoxicity estimation of the iron complexes against C2C12 mouse myoblast cells
Complex |
IC50 (μM) of iron complexes against C2C12 mouse myoblasts |
24 h |
72 h |
Complex 1 |
41.3 ± 1.1 |
14.5 ± 1.3 |
Complex 2 |
59.1 ± 1.1 |
21.6 ± 1.0 |
Complex 3 |
48.9 ± 1.0 |
21.4 ± 1.0 |
Table 5 Calf-thymus (ct) DNA binding data of the iron complexes10,11
Complex |
Equilibrium binding constants (Kb/M−1) determined from UV-vis absorption titrations |
Complex 1 |
3.4 (±0.6) × 106 |
Complex 2 |
6.4 (±0.8) × 105 |
Complex 3 |
2.6 (±0.5) × 105 |
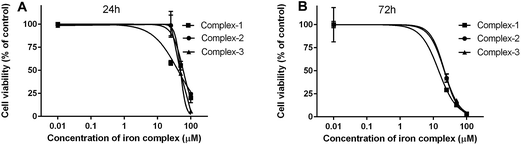 |
| Fig. 8 Cytotoxicity of the iron complexes against C2C12 mouse myoblast cells after (A) 24 h and (B) 72 h of exposure. The data were fit by non-linear regression method after normalization of the treated samples w.r.t untreated control (100% viability). All the data points are mean ± SD of (n = 3) replicates obtained from three independent experiments that yielded similar results. | |
3.4.2 Mode of cell death inferred from flow cytometry data. In order to ascertain the mode of cell death, the cells treated with the iron complexes were analyzed by flow cytometry. In an earlier report, identical iron complexes were reported to localize within the mitochondria of cancerous Hela cells.10 Hence, in the current study, the alteration in the mitochondrial membrane potential was used as an indicator of early apoptosis. Also, the percentage of necrotic cells was detected by propidium iodide (PI) staining. The panels in Fig. 9A and B show bivariate flow cytometry dot plots, revealing the percentage of necrotic (PI stained) cells and apoptotic (JC-1 green stained) cells, respectively, after 24 h exposure to complex 1. Similar flow cytometry data were recorded for identical dosage of iron complexes 2 and 3 (data not shown). Among the three complexes, ligand 2 and ligand 3 coordinated to ferric iron in complex 2 and 3 appeared to trigger mild, but significant necrosis in C2C12 cells (Fig. 10A). On the contrary, a dose-dependent increase in the percentage of early apoptotic/mitochondrial membrane depolarized cells was observed for all the iron complexes and notably in case of the complex 1 (Fig. 10B). Thus, it may be concluded that the iron complexes predominantly caused cytotoxicity via cellular apoptosis. This observation is consistent with an earlier study, wherein DNA fragmentation was used as the marker for apoptosis when cancer cells were treated with iron complexes under photodynamic conditions.10
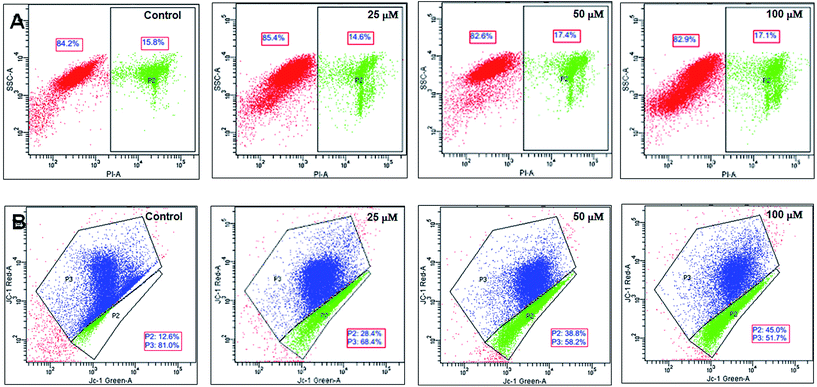 |
| Fig. 9 Mode of cell death detected by flow cytometry after exposure of C2C12 mouse myoblasts to different concentrations of complex 1. Panel (A) shows the percentage of PI stained (necrotic) cells while panel (B) shows the percentages of red (live) and green (apoptotic) fluorescing populations after staining with a mitochondrial membrane potential sensitive probe, JC-1. | |
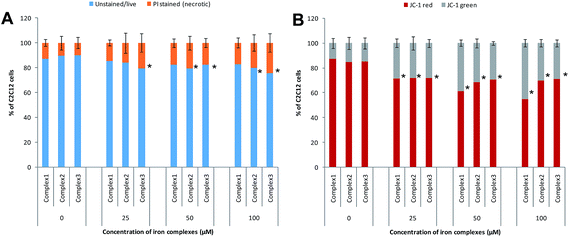 |
| Fig. 10 Flow cytometry analysis of mode of cell death after treatment with different concentrations of iron complexes – (A) bar chart showing the percentage of necrotic cells by PI staining; (B) bar chart showing the percentage of live (JC-1 red stained) and apoptotic/mitochondrial membrane depolarized cells (JC-1 green stained). All the experiments were performed in triplicate (n = 3). Data shown are mean ± SD of replicates obtained from three independent experiments that yielded similar results. * indicate statistically significant difference (p < 0.05) of the iron complex treated samples w.r.t control. | |
3.4.3 TOPKAT toxicity predictions. For therapeutic safety evaluation of our iron complexes in comparison to FDA approved drug molecules, TOPKAT was employed to predict acute systemic toxicity of the ligands via oral and inhalation routes. The rat oral LD50 (LD – lethal dose) module of the statistically-based TOPKAT program performs 19 regression analyses using experimental values of approximately 4000 chemicals from the registry of toxic effects of chemical substances (RTECS) database. Similarly, the rat inhalation LC50 (LC – lethal concentration) values were predicted by applying regression analyses of experimental values from RTECS database. Fig. 11 is a bar chart showing the percentage toxicity (logarithmic axis) of our ligands for oral delivery and inhalation, normalized to the PDT drug porfimer (DB009977) taken as 100%. The TOPKAT predicted values of Rat Inhalational LC50 and Rat Oral LD50 for our ligands and reference molecules (porfimer and doxorubicin) is presented in Table 6. The values clearly indicate the toxicity of all of our ligands are comparable to the FDA approved PDT drug porfimer and the DNA targeted anticancer/antibiotic doxorubicin. Thus, the iron complexes tested in our study are predicted to be safe for therapeutic application.
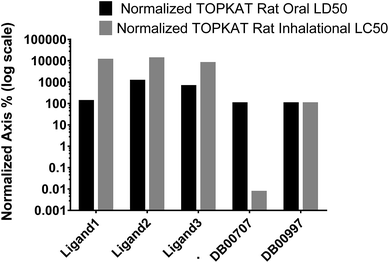 |
| Fig. 11 TOPKAT in vivo toxicity predictions for the ligands of the three iron complexes and a photodynamic therapy (PDT) drug, porfimer (DB00707) normalized to anticancer/antibiotic, doxorubicin (DB00997). The toxicity of doxorubicin was set to 100%. | |
Table 6 TOPKAT in vivo toxicity predictions for the ligands of iron complexesa
Molecule/drug |
Rat_inhalational_LC50 (mg m−3 h−1) |
Rat_oral_LD50 (g kg−1 of body weight) |
TOPKAT |
Experimental |
TOPKAT |
Experimental |
ND – not determined, NA – not applicable, * information from material safety datasheets MSDS. |
Ligand 1 |
5985.149 |
ND |
0.29 |
ND |
Ligand 2 |
7017.849 |
ND |
2.583 |
ND |
Ligand 3 |
4218.606 |
ND |
1.461 |
ND |
Porfimer (DB00707) |
0.004 |
NA |
0.227 |
0.065* |
Doxorubicin (DB00997) |
55.258 |
NA |
0.227 |
0.570–0.698* |
4. Discussion
4.1 Iron complexes elicit bactericidal action by siderophore mediated uptake and DNA fragmentation
Iron is an essential micronutrient for bacteria to replicate and cause infection in vertebrate hosts. Recent works have shown that iron is critical for biofilm formation by bacteria on solid surfaces.38,39 It was elucidated that iron chelators in culture media prevented S. aureus attachment onto material surfaces and reduced polysaccharide intercellular adhesion (PIA) secretion.39 In another study, the addition of gallium (Ga) to the culture media was shown to prevent biofilm formation in Pseudomonas aeruginosa. The chemical similarity of Ga to Fe supposedly interfered in Fe uptake and signaling leading to the anti-biofilm effect.38 The addition of exogenous iron effect reversed the effect in both cases. Under iron starvation, bacteria are reported to secrete iron chelating molecules called siderophores. Based on the chemical moieties that coordinate to iron, siderophores are broadly classified into cateocholate and hydroxamate types.40 There can be two possible antimicrobial strategies based on siderophore production and iron transport: (i) the synthesis of iron chelating molecules with strong binding affinity to iron and thus deprive bacteria with essential iron necessary for biofilm formation. (ii) Siderophore transport also makes such iron chelate producing bacteria more susceptible to the entry of lethal molecules/drugs and antimicrobial payloads conjugated to labile iron complexes.
In the present study, the latter of the above two strategies has been applied successfully against MRSA in planktonic and biofilm cultures. The iron complexes containing identical hydroxamate siderophore moieties and/or different DNA intercalators were 5–10 times less toxic in C2C12 cells as compared to MRSA. Especially in the case of complex 1, a MIC value of ∼3 μM was recorded against MRSA while the IC50 doses against C2C12 cells were ∼40 μM and ∼15 μM after 24 h and 48 h of treatment, respectively. These results are promising in the light of earlier works showing similar photocytotoxicity of the iron complexes in both cancerous and non-cancerous cell lines.10,11 The mechanism of bactericidal action involves siderophore mediated uptake of iron complexes and bacterial DNA fragmentation as testified by gel electrophoresis and comet assay (Fig. 3). A high dose of 100 μM caused complete degradation of both genomic and plasmid DNA by complex 1, while a much lower concentration of 10 μM led to the formation of the formation of distinct comet tails. A comparison of the DNA fragmentation induced by the iron complexes is shown in Fig. 3D.
4.2 DNA as the primary target for the development of next generation antimicrobials
In the light of the emergence of multidrug bacterial resistance, DNA is of late being explored as the primary target in the development of antibacterial drugs.41 In this regard, a host of DNA groove binding molecules in the form of transition metal complexes with aromatic π-acceptor ligands are also being developed.42 Such metal complexes can interact with DNA by – (i) direct metal atom/ion coordination to the DNA bases, (ii) non-covalently by van der Waals or π-interactions of the aromatic residues with DNA, (iii) through heteroatoms such as O, N and S by hydrogen bonding between the heterocyclic aromatic ligands and DNA.43 In the current study, the antimicrobial potential of iron complexes comprising of DNA intercalating ligands has been successfully elucidated against Gram-positive MRSA. Further, an excellent correlation between in silico docking predictions and in vitro antibacterial results arising from bacterial DNA fragmentation is evident from the data presented in Tables 1–3.
However, one of the problems posed by DNA groove binding molecules is their potential host cytotoxicity and lack of specificity/selectivity to the target organisms. One of the methods of achieving selectivity towards bacterial pathogens is the conjugation of antimicrobials/antibiotics to siderophores.44 In the present study, the cytotoxicity of the iron complexes towards mammalian cells have been circumvented considerably by the design of the iron complexes sporting benzhydroxamate ligands. These ligands have a structural similarity to the hydroxamate class of bacterial siderophores, which promoted greater bactericidal than cytocidal action, as suggested by the data in Tables 3 and 4 Further, TOPKAT in vivo toxicity analysis of our iron complexes predicts them to be comparable to FDA approved drug molecules (Table 6). Taken together, we demonstrate in this study the design of iron complexes conjugated to DNA intercalators and siderophore mimicking chelators as a potent strategy for the development of DNA targeting antimicrobial agents.
5. Conclusions
The design of labile iron complexes with a combination of ligands, which possess structural similarity to siderophores for bacterial uptake and those eliciting bactericidal action by DNA intercalation and fragmentation is demonstrated as an efficient strategy to overcome the pathogenic MRSA in the present work. Such iron complexes are 5–10 fold less toxic in C2C12 mouse myoblasts and this presents a therapeutic dosage window for their application as DNA targeting antimicrobial agents. Further, TOPKAT in vivo toxicity predictions confirm the therapeutic safety of the complexes in comparison to representative FDA approved drugs. The experimental bacteriotoxicity and bacterial DNA fragmentation elicited by the iron complexes are commensurate with the molecular docking determined binding affinities of the ligands to DNA and DNA polymerase I. Thus, DNA targeting in conjunction with siderophore mimics can be deployed as an efficient antimicrobial strategy to address persistent bacterial infections.
Acknowledgements
The authors acknowledge the major funding support from the Department of Science and Technology (DST) and Department of Biotechnology (DBT), Govt. of India under ‘Centers of Excellence and Innovation in Biotechnology’ scheme through the center of excellence project – 'Translational Center on Biomaterials for Orthopedic and Dental Applications'. Prof. A. R. Chakravarty from Department of Inorganic and Physical Chemistry, IISc is acknowledged for his guidance and suggestion for synthesis and characterization of the complexes used in this study. Supercomputer Education and Research Centre (SERC), IISc is deeply acknowledged for providing the necessary software and computational tools for performing the Docking and Molecular Dynamics studies. The authors thank the IISc FACS facility for helping with the flow cytometry experiments. One of the authors, Sunil Kumar B. (09/079 (2501)/2011-EMR-I dt. 16-11-2011) acknowledges the Council for Scientific and Industrial Research (CSIR) for providing scholarship during the period of study and Subhendu Pandit acknowledges Department of Science and Technology (DST) for his KVPY undergraduate scholarship.
References
- A. J. M. Messenger and R. Barclay, Bacteria, iron and pathogenicity, Biochem. Educ., 1983, 11(2), 54–63 CrossRef CAS.
- A. Symeonidis and M. Marangos, Iron and Microbial Growth, Insight and Control of Infectious Disease in Global Scenario, 2012, ISBN 978-953-51-0319-6 Search PubMed.
- S. C. Andrews, A. K. Robinson and F. Rodriguez-Quinones, Bacterial iron homeostasis, FEMS Microbiol. Rev., 2003, 27(2–3), 215–237 CrossRef CAS PubMed.
- J. S. Brown and D. W. Holden, Iron acquisition by Gram-positive bacterial pathogens, Microbes Infect., 2002, 4(11), 1149–1156 CrossRef CAS PubMed.
- F. C. Beasley and D. E. Heinrichs, Siderophore-mediated iron acquisition in the Staphylococci, J. Inorg. Biochem., 2010, 104(3), 282–288 CrossRef CAS PubMed.
- M. Miethke and M. A. Marahiel, Siderophore-based iron acquisition and pathogen control, Microbiol. Mol. Biol. Rev., 2007, 71(3), 413–451 CrossRef CAS PubMed.
- B. Schwyn and J. B. Neilands, Anal. Biochem, 1987, 160, 47–56 CrossRef CAS PubMed.
- C. Ratledge and L. G. Dover, Iron metabolism in pathogenic bacteria, Annu. Rev. Microbiol., 2000, 54, 881–941 CrossRef CAS PubMed.
- C. Ji, P. A. Miller and M. J. Miller, Iron Transport-Mediated Drug Delivery: Practical Syntheses and In Vitro Antibacterial Studies of Tris-Catecholate Siderophore-Aminopenicillin Conjugates Reveals Selectively Potent Antipseudomonal Activity, J. Am. Chem. Soc., 2012, 134(24), 9898–9901 CrossRef CAS PubMed.
- A. Garai, U. Basu, I. Khan, I. Pant, A. Hussain, P. Kondaiah and A. R. Chakravarty, Iron(III) benzhydroxamates of dipicolylamines for photocytotoxicity in red light and cellular imaging, Polyhedron, 2014, 73, 124–132 CrossRef CAS.
- A. Garai, U. Basu, I. L. A. Pant, P. Kondaiah and A. R. Chakravarty, Polypyridyl iron(II) complexes showing remarkable photocytotoxicity in visible light, J. Chem. Sci., 2015, 127(4), 609–618 CrossRef CAS.
- N. F. Cariello, J. D. Wilson, B. H. Britt, D. J. Wedd, B. Burlinson and V. Gombar, Comparison of the computer programs DEREK and TOPKAT to predict bacterial mutagenicity. Deductive Estimate of Risk from Existing Knowledge. Toxicity Prediction by Komputer Assisted Technology, Mutagenesis, 2002, 17(4), 321–329 CrossRef CAS PubMed.
- V. Prachayasittikul, S. Prachayasittikul, S. Ruchirawat and V. Prachayasittikul, 8-Hydroxyquinolines: a review of their metal chelating properties and medicinal applications, Drug Des., Dev. Ther., 2013, 7, 1157–1178 CrossRef PubMed.
- R. K. Tewari, G. Bachmann and F. Hadacek, Iron in complex with the alleged phytosiderophore 8-hydroxyquinoline induces functional iron deficiency and non-autolytic programmed cell death in rapeseed plants, Environ. Exp. Bot., 2015, 109, 151–160 CrossRef CAS.
- D. Mossialos, J.-M. Meyer, H. Budzikiewicz, U. Wolff, N. Koedam, C. Baysse, V. Anjaiah and P. Cornelis, Quinolobactin, a New Siderophore of Pseudomonas fluorescens ATCC 17400, the Production of Which Is Repressed by the Cognate Pyoverdine, Appl. Environ. Microbiol., 2000, 66(2), 487–492 CrossRef CAS PubMed.
- A. du Moulinet d'Hardemare, G. Gellon, C. Philouze and G. Serratrice, Oxinobactin and sulfoxinobactin, abiotic siderophore analogues to enterobactin involving 8-hydroxyquinoline subunits: thermodynamic and structural studies, Inorg. Chem., 2012, 51(22), 12142–12151 CrossRef PubMed.
- D. Seeliger and B. L. de Groot, Ligand docking and binding site analysis with PyMOL and Autodock/Vina, J. Comput.-Aided Mol. Des., 2010, 24(5), 417–422 CrossRef CAS PubMed.
- G. M. Morris, R. Huey, W. Lindstrom, M. F. Sanner, R. K. Belew, D. S. Goodsell and A. J. Olson, AutoDock4 and AutoDockTools4: Automated docking with selective receptor flexibility, J. Comput. Chem., 2009, 30(16), 2785–2791 CrossRef CAS PubMed.
- O. Trott and A. J. Olson, AutoDock Vina: Improving the speed and accuracy of docking with a new scoring function, efficient optimization, and multithreading, J. Comput. Chem., 2009, 31(2), 455–461 Search PubMed.
- Accelrys Software Inc., Discovery Studio Modeling Environment, Release, 40, 2013.
- H. R. Drew, S. Samson and R. E. Dickerson, Structure of a B-DNA dodecamer at 16 K, PNAS, 1982, 79(13), 4040–4044 CrossRef CAS.
- A. A. Golosov, J. J. Warren, L. S. Beese and M. Karplus, The mechanism of the translocation step in DNA replication by DNA polymerase I: a computer simulation analysis, Structure, 2010, 18(1), 83–93 CrossRef CAS PubMed.
- S. Unal, J. Hoskins, J. E. Flokowitsch, C. Y. Wu, D. A. Preston and P. L. Skatrud, Detection of methicillin-resistant Staphylococci by using the polymerase chain reaction, J. Clin. Microbiol., 1992, 30(7), 1685–1691 CAS.
- V. Didenko and P. Olive, The Comet Assay, in In Situ Detection of DNA Damage, Humana Press, 2002, vol. 203, pp. 179–194 Search PubMed.
- S. K. Boda, J. Broda, F. Schiefer, J. Weber-Heynemann, M. Hoss, U. Simon, B. Basu and W. Jahnen-Dechent, Cytotoxicity of Ultrasmall Gold Nanoparticles on Planktonic and Biofilm Encapsulated Gram-Positive Staphylococci, Small, 2015, 11(26), 3183–3193 CrossRef CAS PubMed.
- S. K. Boda, I. Bajpai and B. Basu, Inhibitory effect of direct electric field and HA-ZnO composites on S. aureus biofilm formation, J. Biomed. Mater. Res., Part B, 2015 DOI:10.1002/jbm.b.33455.
- S. K. Boda, K. Ravikumar, D. K. Saini and B. Basu, Differential viability response of prokaryotes and eukaryotes to high strength pulsed magnetic stimuli, Bioelectrochemistry, 2015, 106, 276–289 CrossRef CAS PubMed.
- R. M. Murugappan, A. Aravinth, R. Rajaroobia, M. Karthikeyan and M. R. Alamelu, Optimization of MM9 Medium Constituents for Enhancement of Siderophoregenesis in Marine Pseudomonas putida Using Response Surface Methodology, Indian J. Microbiol., 2012, 52(3), 433–441 CrossRef CAS PubMed.
- D. B. Alexander and D. A. Zuberer, Use of chrome azurol S reagents to evaluate siderophore production by rhizosphere bacteria, Biol. Fertil. Soils, 1991, 12(1), 39–45 CrossRef CAS.
- M. Reers, T. W. Smith and L. B. Chen, J-aggregate formation of a carbocyanine as a quantitative fluorescent indicator of membrane potential, Biochemistry, 1991, 30(18), 4480–4486 CrossRef CAS PubMed.
- S. T. Smiley, M. Reers, C. Mottola-Hartshorn, M. Lin, A. Chen, T. W. Smith, G. D. Steele Jr and L. B. Chen, Intracellular heterogeneity in mitochondrial membrane potentials revealed by a J-aggregate-forming lipophilic cation JC-1, PNAS, 1991, 88(9), 3671–3675 CrossRef CAS.
- A. Amini, S. H. Muggleton, H. Lodhi and M. J. E. Sternberg, A Novel Logic-Based Approach for Quantitative Toxicology Prediction, J. Chem. Inf. Model., 2007, 47(3), 998–1006 CrossRef CAS PubMed.
- V. Law, C. Knox, Y. Djoumbou, T. Jewison, A. C. Guo, Y. Liu, A. Maciejewski, D. Arndt, M. Wilson and V. Neveu, et al., DrugBank 4.0: shedding new light on drug metabolism, Nucleic Acids Res., 2014, 42, D1091–D1097 CrossRef CAS PubMed.
- B. M. Gyori, G. Venkatachalam, P. S. Thiagarajan, D. Hsu and M.-V. Clement, OpenComet: An automated tool for comet assay image analysis, Redox Biol., 2014, 2, 457–465 CrossRef CAS PubMed.
- C. C. Ginocchio, Role of NCCLS in antimicrobial susceptibility testing and monitoring, Am. J. Health-Syst. Pharm., 2002, 59(3), S7–S11 Search PubMed.
- E. V. Sorokina, T. P. Yudina, I. A. Bubnov and V. S. Danilov, Assessment of iron toxicity using a luminescent bacterial test with an Escherichia coli recombinant strain, Microbiology, 2013, 82(4), 439–444 CAS.
- R. Z. Sayyed, M. D. Badgujar, H. M. Sonwane, M. M. Mhaske and S. B. Chincholkar, Production of microbial iron chelators (siderophores) by fluorescent Pseudomonads, J. Ind. Microbiol. Biotechnol., 2005, 4, 486–490 Search PubMed.
- Y. Kaneko, M. Thoendel, O. Olakanmi, B. E. Britigan and P. K. Singh, The transition metal gallium disrupts Pseudomonas aeruginosa iron metabolism and has antimicrobial and antibiofilm activity, J. Clin. Invest., 2007, 117(4), 877–888 CrossRef CAS PubMed.
- M.-H. Lin, J.-C. Shu, H.-Y. Huang and Y.-C. Cheng, Involvement of Iron in Biofilm Formation by Staphylococcus aureus, PLoS One, 2012, 7(3), e34388 CAS.
- J. B. Neilands, Methodology of siderophores, in Siderophores from Microorganisms and Plants, Springer, Berlin Heidelberg, 1984, vol. 58, pp. 1–24 Search PubMed.
- A. Bolhuis and J. R. Aldrich-Wright, DNA as a target for antimicrobials, Bioorg. Chem., 2014, 55, 51–59 CrossRef CAS PubMed.
- H.-K. Liu and P. J. Sadler, Metal Complexes as DNA Intercalators, Acc. Chem. Res., 2011, 44(5), 349–359 CrossRef CAS PubMed.
- I. Mames, A. Rodger and J. Kowalski, Tetraaza[14]macrocyclic Transition Metal Complexes as DNA Intercalators, Eur. J. Inorg. Chem., 2015, 630–639 CrossRef CAS.
- T. A. Wencewicz, T. E. Long, U. Mollmann and M. J. Miller, Trihydroxamate Siderophore-Fluoroquinolone Conjugates Are Selective Sideromycin Antibiotics that Target Staphylococcus aureus, Bioconjugate Chem., 2013, 24(3), 473–486 CrossRef CAS PubMed.
Footnotes |
† Electronic supplementary information (ESI) available: See DOI: 10.1039/c6ra02603f |
‡ Equal contribution. |
|
This journal is © The Royal Society of Chemistry 2016 |
Click here to see how this site uses Cookies. View our privacy policy here.