DOI:
10.1039/C6RA02450E
(Paper)
RSC Adv., 2016,
6, 28156-28164
Stable monodisperse colloidal spherical gold nanoparticles formed by an imidazolium gemini surfactant-based water-in-oil microemulsion with excellent catalytic performance
Received
27th January 2016
, Accepted 4th March 2016
First published on 7th March 2016
Abstract
A facile and versatile method for the synthesis of stable monodisperse colloidal gold nanoparticles was developed using a water-in-oil microemulsion-templating strategy. The water-in-oil microemulsion was composed of cationic imidazolium gemini surfactant [C14-4-C14im]Br2, 1-heptane, 1-pentanol and HAuCl4 aqueous solution. The properties of these Au nanoparticles have been fully characterized using transmission electron microscopy (TEM), high-resolution TEM (HR-TEM) observations, X-ray diffraction (XRD), and UV-vis measurements. It can be observed that the monodisperse gold nanoparticles possess a hexagonal close-packed mode and can further aggregate to a rotundity which is shaped like a micro emulsion template while we can not get this kind of morphology using other templates such as [C14min]Br, sodium 1-tetradecanesulphonate or tetradecyltrimethylammonium bromide (TTAB). Moreover, the catalytic efficiency of the gold nanoparticles was evaluated by using the reduction of 4-nitroaniline (4-NA) by potassium borohydride (KBH4) in aqueous solution and electrochemical reduction of hydrogen peroxide (H2O2). These Au nanoparticles possess excellent properties making them fascinating candidates for a variety of applications such as catalysis and life science.
Introduction
Gold nanoparticles, with diameter ranges 1–10 nm (intermediate between the size of small molecules and that of bulk metal) and their arrays are some of the most studied nanomaterials, with promising applications in many fields such as electronics, optoelectronics, catalysis and biology owing to the quantum-mechanical rules.1–4 The resulting physical properties are neither those of bulk metal nor those of molecular compounds, but they strongly depend on the particle size, interparticle distance, nature of the protecting organic shell, and shape of the nanoparticles.5 Furthermore, two or three-dimensionally ordered lattices assembled using monodispersed colloidal spherical gold nanoparticles with tightly controlled sizes show a near-infrared absorption induced by the interparticle plasmonic coupling between adjacent gold nanoparticles, which have shown a number of unique applications in photothermal therapy,6,7 bioimaging, and targeted drug delivery.8,9
In general for the synthesis of gold nanoparticles of different sizes and shapes several procedures are already well-established, such as citrate reduction, the Brust–Schiffrin method, seeding growth, as well as physical methods such as photochemistry (UV, near-IR), sonochemistry, radiolysis, and using thermolysis, microemulsions, copolymer micelles, reversed micelles, and membrane templates.10–13 Among them, the syntheses of gold nanoparticles that involve a two-phase system with or without a surfactant that causes the formation of a microemulsion or a micelle maintaining a favorable microenvironment are superior because they can be directly formed and stabilized in reverse microemulsions with the size and shape controlled by the near-spherical water droplets dispersed in the continuous organic phase.14,15 For example, Lemke et al. have investigated the formation of gold clusters in a tailor-made polyelectrolyte-modified reverse microemulsion using poly(ethyleneimine) (PEI) as a cationic polyelectrolyte. It was found that PEI incorporated into a ternary W/O microemulsion consisting of water/heptanol/zwitterionic surfactant 3-(N,N-dimethyl-dodecylammonio)-propanesulfonate (SB) acts as a reducing and stabilizing agent and shows an additional templating effect. Li et al. developed a facile emulsion-templating approach to fabricate 3D plasmonic colloidosomes (PCs) composed of hexagonal close-packed (HCP) Au nanospheres based on a new reverse (water-in-1-butanol) emulsion system.16 However, the obtained black Au nanoparticles have a large size of 96 ± 2.6 nm, which is unfavorable for catalytic reactions. Chen et al. prepared colloidal Au nanospheres in hexane in a quaternary CTAB/n-pentanol/hexane/water reverse microemulsion (CTAB stands for cetyltrimethylammonium bromide).10 Nevertheless, because of the inalterable structures of CTAB, its size control capability with respect to nanoparticles is often limited. Therefore, the size distribution of CTAB microemulsion-protected gold nanoparticles is rather polydisperse.17–19
Moreover, the stability and assembly of colloidal gold nanoparticles is of great importance for their practical applications in many fields such as nano-optoelectronics, catalysis and bio-assays.20,21 Colloid stability can be controlled using various methods,22,23 for example, increasing electrolyte concentration, adjusting solution pH, adding different inorganic salts, or changing the capping agent or dispersion medium. On the basis of our previous work,24,25 stable monodisperse colloidal gold nanoparticles with small sizes and excellent catalytic performances are developed using a water-in-oil microemulsion-templating strategy. The water-in-oil microemulsion was composed of cationic imidazolium gemini surfactant [C14-4-C14im]Br2, 1-pentanol, 1-heptane and HAuCl4 aqueous solution. For the imidazolium gemini surfactant, the doubly broad imidazolium heads show a high capacity for solutes. Additionally, the attraction between the co-surfactants and the imidazolium ring could facilitate the stability of liquid film. Gemini surfactants are the best shape-directing agents26 and can be used as stabilizers for Au NPs.27 The morphology and properties of these Au nanoparticles have been fully characterized using transmission electron microscopy (TEM), high-resolution TEM (HR-TEM) observations, X-ray diffraction (XRD), and UV-vis measurements. Moreover, the catalytic efficiency properties of these gold nanoparticles were also investigated.
Experimental section
Materials
The cationic imidazolium gemini surfactant ([C14-4-C14im]Br2) was synthesized according to the procedure described in ref. 28 and 29. Cyclohexane, chloroauric acid (HAuCl4), sodium borohydride (NaBH4), 1-pentanol and 1-heptane are from Sinopharm Chemicals. All other chemicals were analytical grade and used as received. Water used in the experiments was triply distilled using a quartz water purification system. Its conductivity was lower than 1.8 μS cm−1 as measured using a DDSJ-308A type conductivity instrument in our laboratory.
Preparation of AuCl4−-containing water-in-oil microemulsion
In the preparation of the Au3+-containing W/O microemulsion, 1-heptane was used as the continuous phase. [C14-4-C14im]Br2 and 1-pentanol were used as the surfactant and co-surfactant, respectively. HAuCl4 aqueous solution with a concentration of 0.25 mol L−1 was used as the discrete phase. The role of 1-pentanol is to lower the interfacial tension between the 1-heptane and water for the spontaneous formation of [C14-4-C14im]Br2 aggregates. Typically, the desired amounts of 1-pentanol were added to 1-heptane to form a stock solution, to which the desired amount of [C14-4-C14im]Br2 and HAuCl4 aqueous solutions were added under mechanical stirring (∼500 rpm). A typical composition of the microemulsion is as follows: 0.024 g [C14-4-C14im]Br2/1.4 mL 1-heptane/0.6 mL 1-pentanol/20 μL HAuCl4 (0.25 mol L−1)/H2O.
Synthesis of Au nanoparticles
In a typical experiment, 20 μL of aqueous solution of NaBH4 (typically 5 mol L−1) was added to the microemulsion mentioned above under stirring. The sample was kept at 25 ± 1 °C for a while to react under continuous stirring. The yellow-orange color of the AuCl4−-containing water-in-oil microemulsion finally changed to the color of dark red-violet immediately after the addition of NaBH4 because of the reaction between HAuCl4 and NaBH4, indicating the formation of Au NP assemblies in the microemulsion. At last, it formed a stable gold sol. During this process, pentanol is expected to increase the film flexibility of the microemulsion, thereby affecting the Au particle growth, and also its absorption on the interface of the microemulsion stabilizes the particles in solution by acting as a capping agent.30
Catalytic activity of gold nanoparticles
In a typical catalysis reaction, 300 μL of 1 mM 4-nitroaniline (4-NA) solution was mixed with 2.4 mL of DI water and stirred for 1–2 min for thorough mixing. After that, 20 μL of the obtained gold nanoparticles solution was added and the mixture was mixed well. Finally, 300 μL of 0.1 M ice-cold aqueous solution of potassium borohydride (KBH4) was added to the reaction mixture. The reaction was monitored using a UV-vis-NIR spectrophotometer. Absorption spectra were recorded every 2 min.
Characterizations
TEM observations were carried out on a JEM 100-CXII with an accelerating voltage of 80 kV. HR-TEM observations were performed on a JEOL-2010 with an accelerating voltage of 200 kV. FE-SEM observations were carried out on a JEOL JSM-6700F. The colloidal gold nanoparticles were also determined using FF-TEM. A small amount of the solution was mounted onto a specimen holder. The sample was frozen by quickly plunging the specimen holder into liquid ethane that had been cooled using liquid nitrogen. Fracturing and replication were carried out on a freeze-fracture apparatus (EM BAF 060, Leica, Germany) at a temperature of 1708 °C. Pt/C was deposited at an angle of 45° to shadow the replicas, and C was deposited at an angle of 90° to consolidate them. The replicas were transferred onto a copper grid and then observed by using a JEOL JEM-1400 transmission electron microscope operated at 120 kV. The images were recorded on a Gatan multiscan CCD and processed with Digital Micrograph.
XRD patterns were collected on a Rigaku D/Max 2200 PC diffractometer with a graphite monochromator and Cu-Kα radiation (λ = 0.15418 nm). For UV-vis measurements, the nanoparticles were dispersed in ethanol and the spectra were collected on a UV-vis spectrophotometer (Lambda-35, Perkin-Elmer).
All electrochemical measurements were carried out on an electrochemical workstation (CHI760D, Chenhua Instrument Shanghai Co., Ltd., China). A conventional three-electrode configuration was used. The working electrode is a glassy carbon electrode (GCE, 4 mm diameter); the reference electrode is a saturated calomel electrode (SCE) and Pt is used as the counter electrode.
Results and discussion
The morphology of gold nanoparticles in aerosol
Firstly, the morphology of the gold nanoparticles in aerosol was investigated and the TEM images of the gold nanoparticles that were synthesized using the water-in-oil microemulsion method are given in Fig. 1. It can be clearly identified from the images that the gold nanoparticles are monodisperse and spherical. The monodisperse gold nanoparticles nearly possess a hexagonal close-packing mode and can be further aggregated to a rotundity which is shaped like a micro emulsion template as shown in Fig. 1D. HR-TEM images (Fig. 2A) further confirm the particle size and the Au character. It can be seen that the lattice of the (111) plane of Au with an interplanar spacing of 0.235 nm could be easily identified. Fig. 2B is the selected area electron diffraction (SAED) pattern of the Au nanodots. Elemental analysis using energy-dispersive X-ray spectroscopy (EDX) also proves that the nanoparticles with a Au component are present and that it is the main element (Fig. 2C). The particle size distribution shows that the gold particles appeared to be essentially spherical with an average diameter of 3.8 ± 0.6 nm and a relatively narrow size distribution (Fig. 2D). All of these results indicated that by using the water-in-oil microemulsion method at 0.024 g [C14-4-C14im]Br2/1.4 mL 1-heptane/0.6 mL 1-pentanol/20 μL HAuCl4 (0.25 mol L−1)/20 μL NaBH4 (5 mol L−1), we can obtain spherical Au nanoparticles with a small and uniform size. Furthermore, FF-TEM measurements were performed to observe our aerosol. It can also be observed that the closely packed hexagonal arrays (Fig. 3) can be observed over large areas (several μm2). The space (ca. 3–5 nm) between two neighboring nanoparticles is slightly less than twice the surfactant chain length of [C14-4-C14im]Br2, suggesting that the partial interdigitation of the hydrophobic chains of [C14-4-C14im]Br2 at the surface of the Au nanocrystals occurs during the formation of the superstructures.31
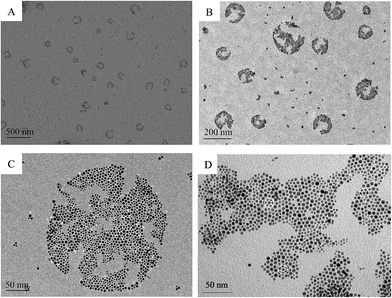 |
| Fig. 1 TEM images with different magnifications of the gold nanoparticles that were synthesized using the water-in-oil microemulsion method at 0.024 g [C14-4-C14im]Br2/1.4 mL 1-heptane/0.6 mL 1-pentanol/20 μL HAuCl4 (0.25 mol L−1)/20 μL NaBH4 (5 mol L−1)/H2O. | |
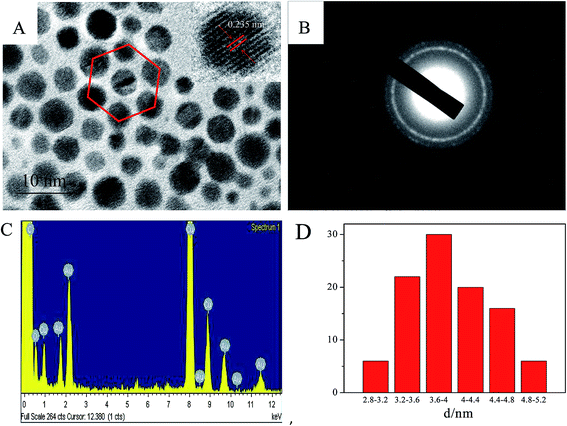 |
| Fig. 2 HR-TEM (A) images of gold nanoparticles that were synthesized using the water-in-oil microemulsion method at 0.024 g [C14-4-C14im]Br2/1.4 mL 1-heptane/0.6 mL 1-pentanol/20 μL HAuCl4 (0.25 mol L−1)/20 μL NaBH4 (5 mol L−1)/H2O. (B) The selected area electron diffraction (SAED) pattern and (C) the EDS results. (D) The particle size distribution calculated based on 100 nanoparticles. | |
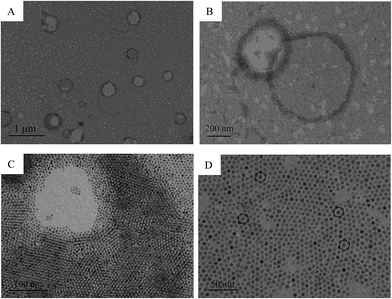 |
| Fig. 3 FF-TEM images with different magnifications of the gold nanoparticles (A–D) that were synthesized using the water-in-oil microemulsion method at 0.024 g [C14-4-C14im]Br2/1.4 mL 1-heptane/0.6 mL 1-pentanol/20 μL HAuCl4 (0.25 mol L−1)/20 μL NaBH4 (5 mol L−1)/H2O. | |
The analysis of the UV-vis absorption and XRD results
Moreover, Fig. 4 shows the UV-vis absorption of the gold nanoparticles that were synthesized using the water-in-oil microemulsion method. It can be seen that the obvious absorption peak for gold nanoparticles (plasmon resonance band) is located at λ = 522 nm, indicating the formation of monodispersed gold nanoparticles in aerosol as can be observed in the TEM images. The wide-angle XRD patterns of the Au nanoparticles are summarized in Fig. 5. It can be seen that the obvious diffraction peaks of the Au nanocrystals locate at 38.25°, 44.46°, and 64.69°, respectively, which corresponds to the (111), (200), and (220) planes of metallic Au with a face-centered cubic (fcc) structure (JCPDS no. 65-8601).32 It should be noted that the diffraction peak corresponding to the (111) plane is the dominant facet of the Au nanocrystal. Moreover, the Au nanoparticles also displays a halo centered at 2θ = 23.8° (Fig. 5), indicating the amorphous carbon feature of the particles which implies that there are remaining surfactants on the surfaces of the Au nanoparticles.
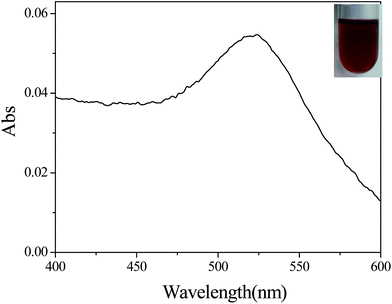 |
| Fig. 4 UV-vis absorption of the gold nanoparticles that were synthesized using the water-in-oil microemulsion method at 0.024 g [C14-4-C14im]Br2/1.4 mL 1-heptane/0.6 mL 1-pentanol/20 μL HAuCl4 (0.25 mol L−1)/20 μL NaBH4 (5 mol L−1)/H2O. Inset is the photo of a typical sample of stable gold sol. | |
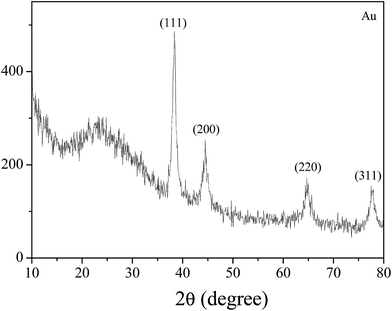 |
| Fig. 5 XRD patterns of Au nanoparticles synthesized at 0.024 g [C14-4-C14im]Br2/1.4 mL 1-heptane/0.6 mL 1-pentanol/20 μL HAuCl4 (0.25 mol L−1)/20 μL NaBH4 (5 mol L−1)/H2O. | |
Factors affecting the formation of gold sol
Since the aggregation and dispersion properties of nano-sized colloidal particles control their optical, electronic and catalytic applications,33,34 the forces that govern the colloid stability and how to control it are important issues and should be considered. The colloid stability of aqueous dispersions is most often explained using the Derjaguin–Landau–Verwey–Overbeek (DLVO) theory.35 In DLVO theory, the height of the energy barrier determines whether a colloid will be stable or undergo coagulation. Factors such as the Hamaker constant, surface potential and electrolyte concentration influence the height of the energy barrier and can be used to control colloid stability. Moreover, hydrophobic, steric and solvation forces are commonly encountered as non-DLVO forces. These additional forces can be much stronger than either of the two DLVO forces at both small and large surface to surface separations to influence the stability of the nano-sized colloidal particles.36,37
Thus, in our present study, in order to explore the mechanism of the formation of a stable gold sol, firstly, we fixed the concentration of HAuCl4 and changed the concentration of NaBH4 to vary the molar ratio of NaBH4 to HAuCl4 (n(HAuCl4/NaBH4)). Ten samples were investigated when n(HAuCl4/NaBH4) = 1
:
8, 3
:
8, 8
:
8, 20
:
8, 40
:
8, 60
:
8, 80
:
8, 160
:
8, 1600
:
8 and 8000
:
8. It can be observed that when (n(HAuCl4/NaBH4)) ≤5
:
1 (40
:
8), they can not form a stable gold sol while when (n(HAuCl4/NaBH4)) >5
:
1, they can. According to the reaction equations for NaBH4 and HAuCl4: 8HAuCl4 + 3NaBH4 + 12H2O = 8Au + 3NaB(OH)4 + 32HCl, although when n(HAuCl4/NaBH4) = 3
:
8, the reaction happens entirely, they can’t form a stable gold sol. This phenomenon indicates that the formation of a stable gold sol must be in excess of NaBH4 to a certain value.
Then, we removed the microemulsion template and just used [C14-4-C14im]Br2 aqueous solution (0.024 g [C14-4-C14im]Br2 with 2 mL water) to synthesize the Au nanoparticles. It can be seen that although the spherical Au nanoparticles can be obtained, their diameters are not uniform, Au nanoparticles tend to aggregate and can not form a stable gold sol. These results further confirmed the conclusion of the previous study that the microemulsion is a favorable microenvironment to synthesize metal nanoparticles which can be directly formed and stabilized in reverse microemulsions with the size and shape controlled by the near-spherical water droplets dispersed in the continuous organic phase (Fig. 6).
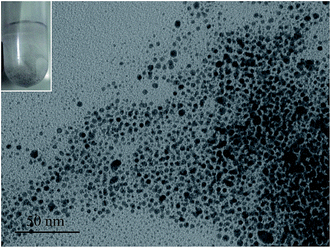 |
| Fig. 6 TEM image of the gold nanoparticles that were synthesized using 0.024 g [C14-4-C14im]Br2/20 μL HAuCl4 (0.25 mol L−1)/20 μL NaBH4 (5 mol L−1)/2 mL H2O. Inset is the photo of a typical sample of unstable gold sol which formed a deposition of gold nanoparticles at the bottom of the test tube. | |
Next, to further confirm the possible mechanism of the formation of the Au NPs, we retained the microemulsion template but changed the types of surfactants with the same hydrophobic chain but different head groups as capping agents to exert exquisite control on the growth and aggregation of the gold nanocrystals. Indeed, in addition to the role of capping agents, surfactants which undergo strong interactions with gold can also significantly influence the growth kinetics of the gold nanocrystals, which considerably influences the morphology of the final products.38 The results revealed that except the [C14-4-C14im]Br2/n-heptane/pentanol/HAuCl4/NaBH4 microemulsion system, other microemulsion systems can not be used to obtain stable gold sols because the gold nanoparticles easily agglomerate and grow and the dimensions are even more than 1 μm in size. TEM images of Au nanostructures produced by replacing [C14-4-C14im]Br2 with [C14min]Br or TTAB are shown in Fig. 7. If we changed the double chains of [C14-4-C14im]Br2 to a single chain of [C14min]Br, large globular or irregular particles were formed. By replacing [C14-4-C14im]Br2 with CTAB, the larger Au polyhedrons are only obtained while when replacing [C14-4-C14im]Br2 with sodium tetradecyl sulfate, one-dimensional nanowires were formed. Thus, it is demonstrated that the structure of the capping agent may affect the patterns formed by the nanoparticles.39,40 In the present study, it can be concluded that the π–π interaction and the steric hindrance of [C14-4-C14im]Br2 are the key factors for the formation of small sized monodisperse spherical gold nanoparticles.
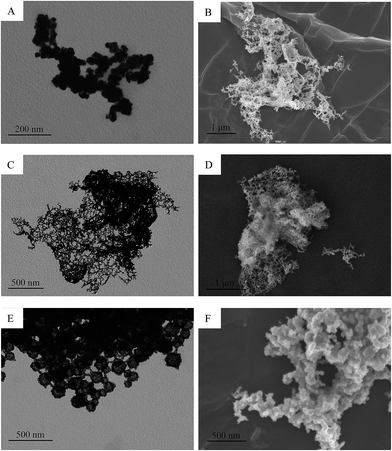 |
| Fig. 7 TEM (A, C and E) and SEM (B, D and F) images of the gold nanostructures with different morphologies that were synthesized using the water-in-oil microemulsion method in the presence of [C14min]Br (A and B), sodium 1-tetradecanesulphonate (C and D) and TTAB (E and F). | |
Thus, according to the experimental results above, a plausible mechanism of the formation of stable monodisperse colloidal gold nanoparticles using the [C14-4-C14im]Br2/1-heptane/1-pentanol/H2O microemulsion template is schematically shown in Scheme 1. After the addition of NaBH4, the water emulsion droplets containing the Au NPs were suspended in 1-heptane. These Au NPs then spontaneously absorbed at the emulsion interface driven by the minimization of the total interfacial free energy where [C14-4-C14im]Br2 were also adsorbed,41,42 and hence a single layer of Au NPs was trapped at the water/1-heptane interface. As the time went on, the second and third layers of Au NPs slowly formed. At last, the Au NPs were closely packed on the entire droplet surface which aggregated to a rotund shape like a micro emulsion template.16
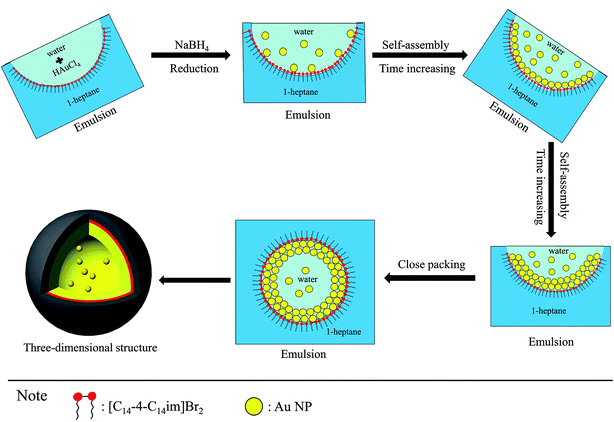 |
| Scheme 1 Schematic illustration of the formation of stable monodisperse colloidal gold nanoparticles using the [C14-4-C14im]Br2/1-heptane/1-pentanol/H2O microemulsion template. | |
Catalytic activity of the gold nanoparticles
The catalytic efficiency of our gold nanoparticles was evaluated by using the reduction of 4-NA by KBH4 in aqueous solution. Fig. 8 shows the time-dependent absorption spectra of the reduction of 4-NA by KBH4 in the presence of Au NPs (Fig. 8A). It has been reported that the absorption spectrum of a mixture of 4-NA and KBH4 shows a band at 380 nm corresponding to the intermolecular charge transfer of 4-NA which is similar to other aromatic nitro compounds.43 The results indicated that without the catalyst, the absorption peak will not change with time which implied that the reduction reaction can not occur. After the introduction of the Au NPs, the mixed solution can be quickly changed from light yellow to colorless, indicating that the Au NPs prepared by using the micro-emulsion template can successfully catalyze the reduction reaction. According to Liang et al.’s assumptions43, the approximate number of nanoparticles in our system is about 5.1 × 1011 mL−1 that is, 8.47mol mL−1. The number of nanoparticles in our catalytic reaction system is similar to the previous reports.43,44 The gold nanoparticles can be used repeatedly at least 3 times and the ka for the three cycles were 0.1042 min−1, 0.0872 min−1 and 0.0524 min−1, as measured from the plots of ln(At/A0) vs. time shown in Fig. 8B, D and F. To make the catalytic efficiency clear, the TOF (turnover frequency)45 was calculated. The TOFs are 4.65 × 106 mol (mol−1 min−1), 2.53 × 106 mol (mol−1 min−1), and 1.84 × 106 mol (mol−1 min−1) for the three cycles, which indicated that some of the Au NPs lost their catalytic activity in recirculation. This good catalytic activity could be attributed to the higher surface areas of these small-size spherical gold nanoparticles. Thus, it can be seen that the gold nanoparticles showed high and stable catalytic activity for the reduction of 4-nitroaniline by KBH4 at room temperature and may be used as a reusable catalyst in some heterogeneous catalytic reactions.46
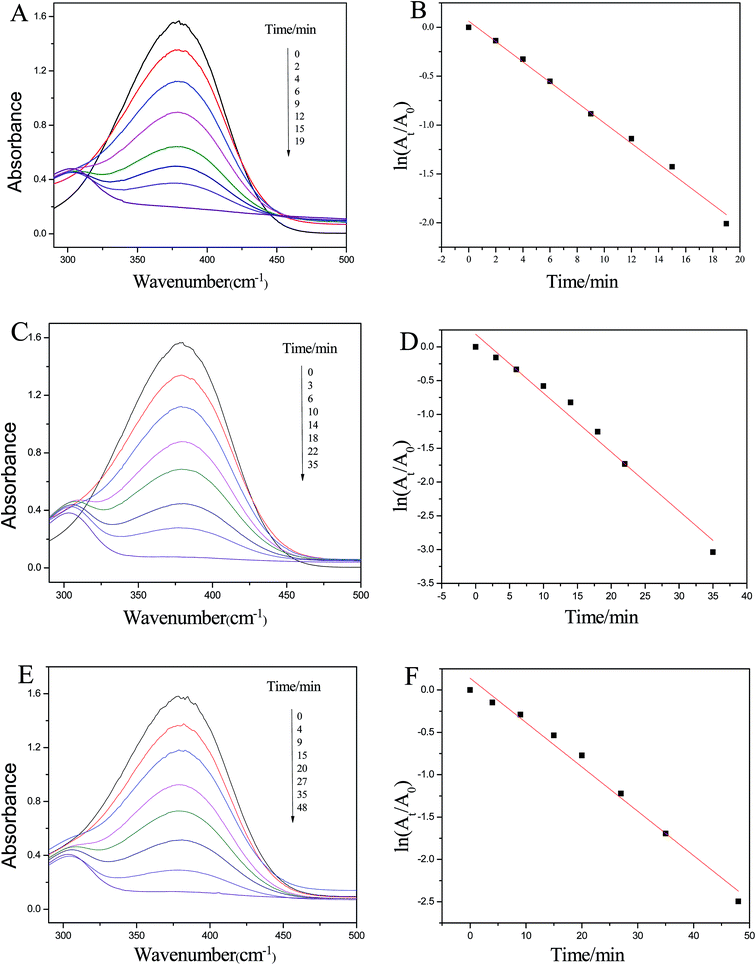 |
| Fig. 8 Time-dependent absorption spectra for the catalytic reduction of 4-nitroaniline by KBH4 in aqueous solution in the presence of the Au NPs prepared by using the micro-emulsion template for the first (A), second (C) and third cycle (E), and (B, D and F) the corresponding linear relationship between ln(At/A0) and reduction time. | |
Electrocatalytic behavior towards H2O2
The electrocatalytic activity of the as-prepared Au nanoparticles towards the oxidation/reduction of H2O2 was studied because detection of H2O2 is practically important in the field of biosensor development.47 For H2O2, the CVs were measured in a 5 mmol L−1 H2O2/0.0667 mol L−1 phosphate buffer solution (PBS, pH 7.0). Fig. 9 shows the CVs of glassy carbon electrodes modified with the Au nanoparticles in the absence and presence of 5 mmol L−1 H2O2. Obvious increases in the oxidation and reduction currents are observed on the Au nanoparticles on addition of H2O2. Therefore, it can be seen that the as-prepared Au nanoparticles may be promising for the fabrication of effective H2O2 sensors.48
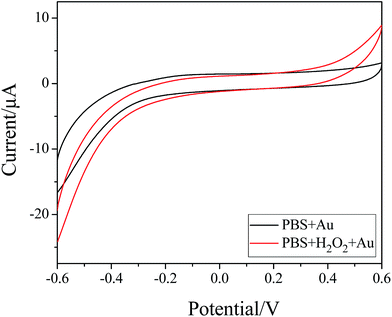 |
| Fig. 9 Cyclic voltammograms of Au NP modified electrodes in N2-saturated PBS without (black) and with (red) 5 mM H2O2. | |
Conclusions
Stable monodisperse colloidal gold nanoparticles were prepared using a [C14-4-C14im]Br2/1-heptane/1-pentanol/H2O water-in-oil microemulsion template and the properties of these Au nanoparticles were characterized using TEM, HR-TEM, SEM, XRD, and UV-vis measurements. Moreover, the catalytic efficiencies of the gold nanoparticles were also evaluated. The results indicated that the spherical Au NPs with an average diameter of 3.8 ± 0.6 nm were closely packed on the entire droplet surface which aggregated to a rotund shape like a micro emulsion template and they possessed good catalytic performances for the catalytic reduction of 4-nitroaniline and electrochemical catalysis of H2O2. The simplicity, good morphology and catalytic performance of the stable monodisperse colloidal gold nanoparticles may be quite promising in applications for catalysis such as for the development of effective H2O2 sensors.
Acknowledgements
We gratefully acknowledge the financial support from the National Natural Science Foundation of China (21476129, 21203109) and the Ji’nan Youth Science and Technology Star Program (2013040).
References
- C. M. Cobley, J. E. Chen, C. L. Cho, V. Wang and Y. Xia, Chem. Soc. Rev., 2011, 40, 44 RSC.
- H. W. Liang, S. Liu and S. H. Yu, Adv. Mater., 2010, 22, 3925 CrossRef CAS PubMed.
- J. F. Zhou, J. Ralston, R. Sedev and D. A. Beattie, J. Colloid Interface Sci., 2009, 331, 251 CrossRef CAS PubMed.
- H. Jia, X. T. Bai, L. J. Shi, F. Lu and L. Q. Zheng, Nanoscale, 2012, 4, 3162 RSC.
- M. Brust and C. J. Kiely, Colloids Surf. A. Physicochem. Eng. Asp., 2002, 202, 175 CrossRef CAS.
- P. Huang, J. Lin, W. Li, P. Rong, Z. Wang, S. Wang, X. Wang, X. Sun, M. Aronova and G. Niu, Angew. Chem., Int. Ed., 2013, 52, 13958 CrossRef CAS PubMed.
- J. Lin, S. Wang, P. Huang, Z. Wang, S. Chen, G. Niu, W. Li, J. He, D. Cui and G. Lu, ACS Nano, 2013, 7, 5320 CrossRef CAS PubMed.
- J. Song, J. Zhou and H. Duan, J. Am. Chem. Soc., 2012, 134, 13458 CrossRef CAS PubMed.
- J. Song, L. Pu, J. Zhou, B. Duan and H. Duan, ACS Nano, 2013, 7, 9947 CrossRef CAS PubMed.
- F. X. Chen, G.-Q. Xu and T. S. A. Hor, Mater. Lett., 2003, 57, 3282 CrossRef CAS.
- B.-H. Sohn, J.-M. Choi, S. I. I. Yoo, S.-H. Yun, W.-C. Zin, J. C. Jung, M. Kanehara, T. Hirata and T. Teranishi, J. Am. Chem. Soc., 2003, 125, 6368 CrossRef CAS PubMed.
- M. C. Daniel and D. Astruc, Chem. Rev., 2004, 104, 293 CrossRef CAS PubMed.
- K. Lemke, C. Prietzel and J. Koetz, J. Colloid Interface Sci., 2013, 394, 141 CrossRef CAS PubMed.
- M.-L. Wu, D.-H. Chen and T.-C. Huang, Chem. Mater., 2001, 13, 599 CrossRef CAS.
- M. L. Curri, A. Agostiano, L. Manna, M. D. Monica, M. Catalano, L. Chiavarone, V. Spagnolo and M. Lugarà, J. Phys. Chem. B, 2000, 104, 8391 CrossRef CAS.
- D. Liu, F. Zhou, C. Li, T. Zhang, H. H. Zhang, W. P. Cai and Y. Li, Angew. Chem., Int. Ed., 2015, 54, 9596 CrossRef CAS PubMed.
- S. Mandal, S. K. Arumugam, S. D. Adyanthaya, R. Pasricha and M. Sastry, J. Mater. Chem., 2004, 14, 43 RSC.
- L. Zhang, X. Sun, Y. Song, X. Jiang, S. Dong and E. Wang, Langmuir, 2006, 22, 2838 CrossRef CAS PubMed.
- A. Swami, A. Kumar and M. Sastry, Langmuir, 2003, 19, 1168 CrossRef CAS.
- M.-C. Daniel and D. Astruc, Chem. Rev., 2004, 104, 293 CrossRef CAS PubMed.
- H. Bonnemann and R. M. Richards, Eur. J. Inorg. Chem., 2001, 2455 CrossRef CAS.
- K. Watanabe, D. Menzel, N. Nilius and H.-J. Freund, Chem. Rev., 2006, 106, 4301 CrossRef CAS PubMed.
- P. C. Hiemenz and R. Rajagopalan, Principles of Colloid and Surface Chemistry, Marcel Dekker, New York, 3rd edn, 1997 Search PubMed.
- Y. J. Ren, Y. H. Zhao, Y. J. Zhang, W. Y. Tang, X. Xin, J. L. Shen and L. Wang, Colloids Surf. A. Physicochem. Eng. Asp., 2015, 486, 14 CrossRef CAS.
- Y. J. Ren, X. Xin, W. Y. Tang, Y. J. Zhang, J. L. Shen and L. Wang, Colloid Polym. Sci., 2015, 293, 1695 CAS.
- M. S. Bakshi, Langmuir, 2009, 25, 12697 CrossRef CAS PubMed.
- J. Xu, L. Zhou, H. Liu and Y. Hu, J. Exp. Nanosci., 2006, 1, 103 CrossRef CAS.
- M. Q. Ao, G. Y. Xu, Y. Y. Zhu and Y. Bai, J. Colloid Interface Sci., 2008, 326, 490 CrossRef CAS PubMed.
- M. Q. Ao, P. P. Huang, G. Y. Xu, X. D. Yang and Y. J. Wang, Colloid Polym. Sci., 2009, 287, 395 CAS.
- A. K. Ganguli, A. Ganguly and S. Vaidya, Chem. Soc. Rev., 2010, 39, 474 RSC.
- B. Messer, J. H. Song, M. Huang, Y. Wu, F. Kim and P. Yang, Adv. Mater., 2000, 12, 1526 CrossRef CAS.
- J. Y. Xiao and L. M. Qi, Nanoscale, 2011, 3, 1383 RSC.
- Y. Hu and J. Dai, Miner. Eng., 2003, 16, 1167 CrossRef CAS.
- D. R. E. Snoswell, J. Duan, D. Fornasiero and J. Ralston, J. Colloid Interface Sci., 2005, 286, 526 CrossRef CAS PubMed.
- P. C. Hiemenz and R. Rajagopalan, Principles of Colloid and Surface Chemistry, Marcel Dekker, New York, 3rd edn, 1997 Search PubMed.
- J. Israelachvili, Intermolecular & Surface Forces, Academic Press Limited, London, 2nd edn, 1991 Search PubMed.
- J. H. Lyklema, Fundamentals of Interface and Colloid Science, in: Particulate Colloids, Elsevier/Academic Press, Amsterdam, 2005, vol. IV Search PubMed.
- H. Jia, X. T Bai, N. Li, L. Yu and L. Q. Zheng, CrystEngComm, 2011, 13, 6179 RSC.
- X. T. Bai, H. C. Ma, X. W. Li, B. Dong and L. Q. Zheng, Langmuir, 2010, 26, 14970 CrossRef CAS PubMed.
- H. Jia, X. T. Bai and L. Q. Zheng, CrystEngComm, 2012, 14, 2920 RSC.
- Z. Mao, H. Xu and D. Wang, Adv. Funct. Mater., 2010, 20, 1053 CrossRef CAS.
- Z. Niu, J. He, T. P. Russell and Q. Wang, Angew. Chem., Int. Ed., 2010, 49, 10052 CrossRef CAS PubMed.
- S. Kundu, S. Lau and H. Liang, J. Phys. Chem. C, 2009, 113, 5150 CAS.
- C. J. Murphy, T. K. Sau, A. Gole, C. J. Orendorff, J. Gao, L. Gou, S. Hunyadi and T. Li, J. Phys. Chem. B, 2005, 109, 13857 CrossRef CAS PubMed.
- A. M. Azevedo, J. M. S. Cabral, T. D. son and L. P. Fonseca, J. Mol. Catal. B: Enzym., 2004, 28, 45 CrossRef CAS.
- H. Jia, X. P. Gao, Z. L. Chen, G. Q. Liu, X. Zhang, H. Yan, H. T. Zhou and L. Q. Zheng, CrystEngComm, 2012, 14, 7600 RSC.
- A. Kuhn and D. Martel, Electrochim. Acta, 2000, 45, 1829 CrossRef.
- Z. H. Li, R. Li, T. C. Mu and Y. X. Luan, Chem.–Eur. J., 2013, 19, 6005 CrossRef CAS PubMed.
|
This journal is © The Royal Society of Chemistry 2016 |