DOI:
10.1039/C6RA02422J
(Paper)
RSC Adv., 2016,
6, 33569-33579
Multi-modal imaging of HeLa cells using a luminescent ZnS:Mn/NaGdF4:Yb:Er nanocomposite with enhanced upconversion red emission
Received
27th January 2016
, Accepted 29th March 2016
First published on 30th March 2016
Abstract
The multi-modal luminescence properties of the ZnS:Mn/NaGdF4:Yb:Er nanocomposite were investigated. The ZnS:Mn/NaGdF4:Yb:Er nanocomposite was synthesized using a single-step hot injection method. Its crystallinity was confirmed using X-ray diffraction, and was compared with the crystallinities of NaGdF4:Yb:Er nanoparticles and ZnS:Mn quantum dots synthesized by thermal decomposition. Composite formation and morphology changes were investigated by transmission electron microscopy. Enhanced upconversion red emission (654 nm, 4F9/2–4I15/2) was observed for NaGdF4:Yb:Er in the presence of ZnS:Mn. Analysis of the excitation and downconversion emission spectra of ZnS:Mn/NaGdF4:Yb:Er indicated modified emission at lower wavelengths. Cathodoluminescence measurements indicated that the ZnS:Mn/NaGdF4:Yb:Er nanocomposite had a broad visible emission from green to red wavelengths. Cytotoxicity and stability measurements were executed to ensure the toxicity of the synthesized composite and their luminescence stability in water as well as phosphate buffered solution. The nanocomposite had multi-modal characteristics, and was used in the upconversion and downconversion optical imaging of living He–La cells. The multi-modal luminescence capability of the nanocomposite was suitable for upconversion and downconversion imaging.
Introduction
Inorganic luminescent nanostructures have gained much attention in biomedical research, because of their potential clinical bio-imaging and targeted drug therapy applications.1 Many inorganic nanostructures have been developed with enhanced bioimaging performance in the last few decades.2,3 The evolution of multi-modal bioimaging diagnostics has progressed towards hybrid nanostructures with multiple properties.4 Imaging research has focused on synthesizing imaging probes with two or more diagnostic abilities. Examples include multiple excitation wavelengths for emission in fluorescence imaging, magnetic properties for magnetic resonance imaging (MRI), and thermionic sensitive probes.5,6 Many luminescent nanomaterials have been used for bio-imaging. These include doped silica, hydrophilic and hydrophobic organic polymers, semiconducting polymer dots, carbon dots, metal chalcogenide quantum dots (QDs), upconversion nanoparticles, noble metal nanoparticles, dendrimers, lipid drops, and micelles.7,8 Downconversion QDs and upconversion nanomaterials have been intensively investigated as promising nanomaterials in bio-medicine, such as in imaging and photo-controlled drug delivery.9,10 Hybrid nanostructures have recently been developed for multi-modal bio-imaging. These can be excited by upconversion and downconversion, by combining two different luminescent nanomaterials.
Binary semiconductor QDs are efficient downconversion luminescent probes for bio-imaging, because of their favorable luminescence properties.11 Some of these chalcogenide QDs have been based on toxic Cd and Te ions. ZnS QDs have attracted much attention, because of the intoxication of Zn2+ and S2− in the human body. ZnS QDs can be doped with luminescent ions at Zn2+ sites, which many other chalcogenide QDs cannot. Manganese-doped materials have been used in many bio-medical applications, and Mn2+ emission has been investigated in many host materials for bio-imaging.12 ZnS is an ideal host for Mn2+, which leads to orange luminescence arising from the 4T1–6A1 transition.13,14
Fluoride-based upconversion nanoparticles have been widely investigated in bio-imaging.9,15,16 LaF3, YF3, BaYF4, NaYF4 and NaGdF4 have been used as host lattices for upconversion lanthanide ions, because of their low photon energy (<400 cm−1) and high chemical stability.17,18 β-NaGdF4 (NGF) is an efficient host for Yb3+ and Er3+/Tm3+ upconversion, resulting in bright luminescence.19 NGF host materials also exhibit magnetic properties, and thus can act as contrast agents in MRI with positive relaxation times.20 Several studies have investigated upconversion fluoride nanoparticles for in vivo imaging; however, the development of multi-modal imaging has led to more accurate imaging and diagnostics. New model imaging probes are being developed to improve image quality and multiple diagnostic capabilities.
Composite nanostructures have been synthesized from pre-prepared ZnS:Mn and NaGdF4:Yb:Er particles assembled in different matrices.21,22 In the present study, we synthesized a ZnS:Mn/NaGdF4:Yb:Er nanocomposite in a single-step hot-injection method. The nanocomposites growth morphology was compared with those of ZnS:Mn QDs and NaGdF4:Yb:Er nanoparticles synthesized by thermal decomposition. The ZnS:Mn/NaGdF4:Yb:Er nanocomposite was then applied in the downconversion and upconversion optical imaging of living HeLa cells.
Materials and methods
Materials
Zinc acetate (Zn(O2CCH3)2, 99%), elemental sulfur, manganese(II) acetate (Mn(O2CCH3)2, 99%), gadolinium oxide (Gd2O3, 99.9%) ytterbium oxide (Yb2O3, 99.9%), erbium oxide (Er2O3, 99.99%) cyclohexane, and acetone were purchased from Wako Pure Chemical Industries Ltd., Japan. CF3COONa (98%), oleylamine (technical grade, 70%), trifluoroacetic acid (TFA, 99%), phosphate buffered saline (PBS), and Dulbecco's modified eagle's medium (DMEM) were purchased from Sigma Aldrich Company, USA. 10% fetal bovine serum (FBS), and HeLa cells were obtained from MP Biomedicals Inc., Japan, and European Collection of Authenticated Cell Cultures (ECACC), United Kingdom, respectively. All chemicals were used as purchased without further purification.
Synthesis of ZnS:Mn QDs
Manganese-doped ZnS QDs were synthesized by thermal decomposition. Zinc and manganese acetates and sulfur were dissolved in 10 mL of oleylamine, which was then degassed at 160 °C under vacuum. The thoroughly degassed oleylamine solution was heated to 270 °C and maintained at this temperature for 1 h, to decompose the acetates and form ZnS:Mn QDs. The solution was then cooled to room temperature, and washed thoroughly with acetone. The ZnS:Mn QDs were then dispersed in cyclohexane.
Synthesis of NaGdF4:Yb:Er nanoparticles
Precursor rare-earth trifluoroacetates (Gd(CF3COO)3, Yb(CF3COO)3 and Er(CF3COO)3) were prepared by a recrystallization method.23 Rare earth oxides were dissolved in 1
:
1 TFA
:
water at 80 °C for 12 h. After dissolution, undissolved minor oxides were removed by filtration, and the solution was dried at 60 °C to remove superfluous liquid. The synthesized rare earth trifluoroacetates and commercially available CF3COONa were dissolved in oleylamine, which was heated to 160 °C and degassed for 30 min under vacuum. The solution was further heated to 330 °C and maintained at this temperature for 2 h, to synthesis single phase NaGdF4:Yb:Er. The solution was then cooled to room temperature and washed with ethanol.
Synthesis of the ZnS:Mn/NaGdF4:Yb:Er nanocomposite
Composite ZnS:Mn/NaGdF4:Yb:Er nanoparticles were synthesized by the precursor hot injection method. Zinc and manganese acetates and elemental sulfur were dissolved in 15 mL of oleylamine in a three-neck flask. The mixture was degassed at 160 °C under vacuum for 30 min, to remove water and oxygen. The flask was then filled with N2 gas, and heated to 280 °C for 60 min. Meanwhile, trifluoroacetates of sodium (2 mmol), gadolinium (0.798 mmol), ytterbium (0.20 mmol) and erbium (0.02 mmol) were mixed together in 5 mL of oleylamine in a separate three-neck flask. The mixture was degassed at 160 °C under vacuum. The degassed solution was loaded into a glass syringe, and injected into the ZnS:Mn particle-containing flask at 280 °C. The resulting mixture was heated to 330 °C for 120 min under an Ar stream with constant stirring. The resulting mixture was cooled to 80 °C, and diluted with ethanol. The centrifuged materials were washed twice with acetone, and then dispersed in cyclohexane.
Surface modification from hydrophobic to hydrophilic
The hydrophobic oleylamine-capped ZnS:Mn/NaGdF4:Yb:Er nanocomposite was rendered hydrophilic using a reported procedure. 100 mg of synthesized ZnS:Mn/NaGdF4:Yb:Er nanocomposite particles were mixed with 20 mL of acetic acid. The mixture was heated at 75 °C for 2 h with constant stirring. The solution was then diluted with acetone and centrifuged. The obtained nanocomposite was washed twice with acetone, and then dispersed in deionized water.
Cytotoxicity and stability measurements
HeLa cells were cultured on a sterilized glass Petri dish at 37 °C and kept it for 24 h for incubation to ensure the adherence of the cells on the glass plate. 100 μL (4 μg mL−1) of surface modified ZnS:Mn/NaGdF4:Yb:Er composite particles were added to the HeLa cell cultured plates and again incubated for another 24 h. Cytotoxicity of the composite particles was evaluated using calcein – ethidium homodimer – 1 viability/cytotoxicity kit. For stability measurements, surface modified composite particles were aged in water and phosphate buffered saline (PBS) for different time period (1 h, 3 days and 1 week). Aged particles were dispersed on the glass plates and luminescence stability measurements were performed in microscope under 365 nm excitation and washed with deionized water for morphology stability measurements.
Bioimaging experiments
HeLa cells were cultured on a glass Petri dish in DMEM medium at 37 °C with FBS under humidified 5% CO2 conditions. Hydrophilic ZnS:Mn/NaGdF4:Yb:Er nanocomposite dispersion (4 μg mL−1) was mixed with the adhered HeLa cells, and the resulting mixture was incubated for 30 min. Prior to observation, the cells were washed several times with PBS to remove excess nanocomposite. The HeLa cells containing ZnS:Mn/NaGdF4:Yb:Er were examined in upconversion (970 nm) and downconversion (365 nm) imaging experiments. The results were compared with images of HeLa cells without ZnS:Mn/NaGdF4:Yb:Er.
Instrumentation
Crystal structures were determined by powder X-ray diffraction (XRD), with a scan rate of 0.04° s−1 in the 2θ range of 10–80°, using a Rigaku (Japan) diffractometer (RINT-2200, Cu Kα radiation, λ = 1.54178 Å). Particle morphologies and sizes were examined by transmission electron microscopy (TEM) and high-resolution TEM (HRTEM), using a JEOL TEM 2100F microscope (Japan) at an accelerating voltage of 200 kV. Downconversion luminescence spectra were measured with a JASCO FB-8600 fluorescence spectrophotometer, Japan. Upconversion spectra were captured with a custom-built luminescence system, consisting of an OceanOptics USB4000 spectrometer (USA) with excitation at 980 nm provided by a continuous laser (Changchun New Industries Optoelectronics Technology Co., Ltd.). Cathodoluminescence (CL) measurements were carried out using a JEOL JSM-7001F field emission scanning electron microscope (FE-SEM), Japan. Emission from samples was collected by a parabolic mirror, and an attached optical fiber leading to an Acton SP2300 spectrograph (Princeton Instruments). Downconversion optical images were acquired by an Olympus (IX71) microscope. Upconversion images were obtained using a purpose built optical setup consisting of a 970 nm laser (THORLABS, M970L3), LUSPlan FL N X40 eye piece, and HAMAMATSU ORCA-flash 4.0 CMOS camera (Japan).
Results and discussion
The crystallinity of the ZnS:Mn QDs, NaGdF4:Yb:Er nanoparticles, and ZnS:Mn/NaGdF4:Yb:Er nanocomposite was investigated by powder XRD. Their XRD patterns and comparison with standard JCPDS files are shown in Fig. 1. The pattern of ZnS:Mn was consistent with a zinc blende structure and the standard ZnS structure of JCPDS no. 01-0792.24 The XRD pattern of NGF:Yb:Er was consistent with the standard hexagonal NaGdF4 crystal structure (JCPDS no. 027-0699).25 Peaks in the XRD pattern of ZnS:Mn/NaGdF4:Yb:Er were indexed to hexagonal NaGdF4 (JCPDS no. 27-0699) and ZnS (JCPDS no. 01-0792). The peaks associated with ZnS:Mn in the XRD pattern of the composite were sharp. The full-width half-maximum of the significant plane (111) of ZnS:Mn (2.26° 2θ) and ZnS:Mn/NaGdF4:Yb:Er (0.96° 2θ) suggested that the ZnS:Mn particle size increased during the high-temperature synthesis of the NaGdF4:Yb:Er composite. During one pot synthesis, ZnS:Mn particles were synthesized at 280 °C and the precursors of NaGdF4:Yb:Er were injected and further increased 330 °C for the growth of the ZnS:Mn/NaGdF4:Yb:Er. The temperature increment from 280 to 330 °C leads increased particle size of the ZnS:Mn. The high-temperature growth of NaGdF4:Yb:Er and the long heating time resulted in an increase in crystallinity of the ZnS:Mn particles.
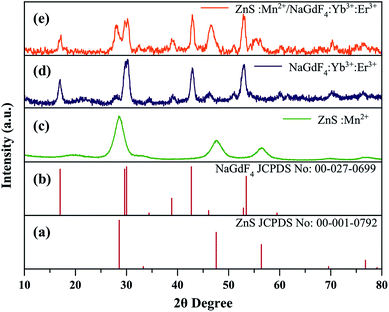 |
| Fig. 1 XRD patterns of the standard cards of ZnS (a) and NaGdF4 (b), and as-synthesized ZnS:Mn QDs (c), NaGdF4:Yb:Er nanoparticles (d), and ZnS:Mn/NaGdF4:Yb:Er nanocomposite (e). | |
Fig. 2 shows TEM and HRTEM images of the ZnS:Mn QDs. The TEM images showed that the QDs were well dispersed and nearly spherical in shape (Fig. 2a and b). The HRTEM image revealed the mono-crystalline nature of the QDs. The lattice spacing of 3.02 Å was consistent with the (111) plane of zinc blende ZnS (Fig. 2c).26 The diameters of the QDs were 2–6 nm, with most being ∼3 nm, as shown by the histogram in Fig. 2d.
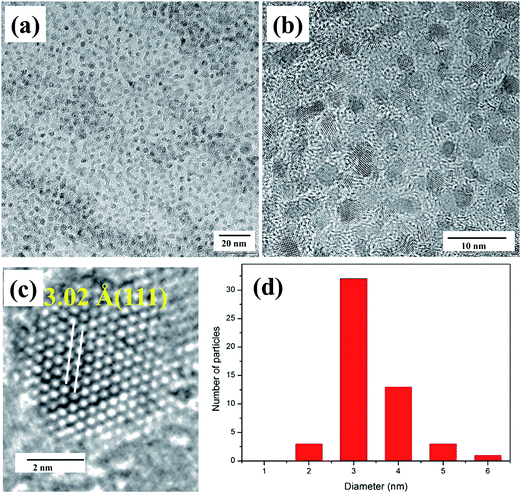 |
| Fig. 2 TEM (a and b) and HR-TEM (c) images and particle size distribution (d) of the ZnS:Mn QDs. | |
TEM and HRTEM images and the particle size distribution of the NaGdF4:Yb:Er nanoparticles are shown in Fig. 3. TEM images in Fig. 3a and b show well-dispersed spherical particles at various magnifications. The HRTEM image in Fig. 3c shows a lattice spacing of 5.42 Å oriented along with (100) plane of the hexagonal NaGdF4:Yb:Er. The histogram in Fig. 3d shows that the particle size was 8–20 nm. TEM images indicated that the NaGdF4:Yb:Er nanoparticles grown in oleylamine were spherical and uniform in shape.
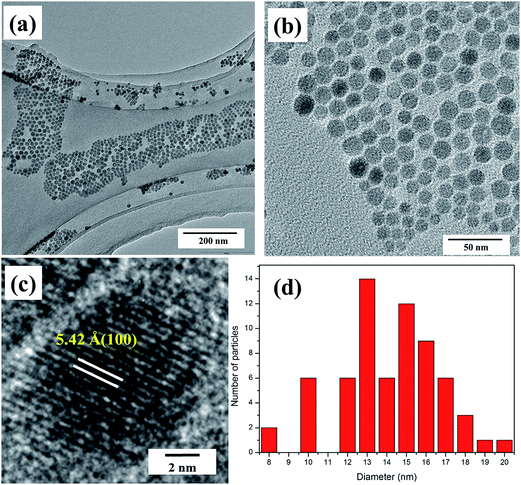 |
| Fig. 3 TEM (a and b) and HR-TEM (c) images and particle size distribution (d) of the NaGdF4:Yb:Er nanoparticles. | |
Fig. 4 shows TEM and HR-TEM images of the ZnS:Mn/NaGdF4:Yb:Er nanocomposite. The ZnS:Mn/NaGdF4:Yb:Er nanocomposite particles appeared as bulged sphere-like shapes of different sizes (Fig. 4a). Uneven growth of the NGF:Yb:Er nanoparticles was observed in the presence of the ZnS:Mn QDs (Fig. 4b). The HR-TEM images in Fig. 4(c and d) showed the hybrid nature of the ZnS:Mn/NaGdF4:Yb:Er nanocomposite, and the composite growth originated from the ZnS:Mn seeds was confirmed. Composite particle has two types of crystalline planes. One is the crystalline planes of ZnS (111) with an interlayer spacing of 3.12 Å and the other is crystalline planes related to NaGdF4 (d(101) = 2.81 Å and d(100) = 5.10 Å). This suggested that the ZnS:Mn particle size had increased to 7 nm, and was consistent with the above XRD results. Thus, NaGdF4:Yb:Er growth was strongly affected by the presence of the ZnS:Mn QDs. This resulted in spherical particles of different sizes, compared with the growth of NaGdF4:Yb:Er nanoparticles in the absence of the ZnS:Mn QDs.
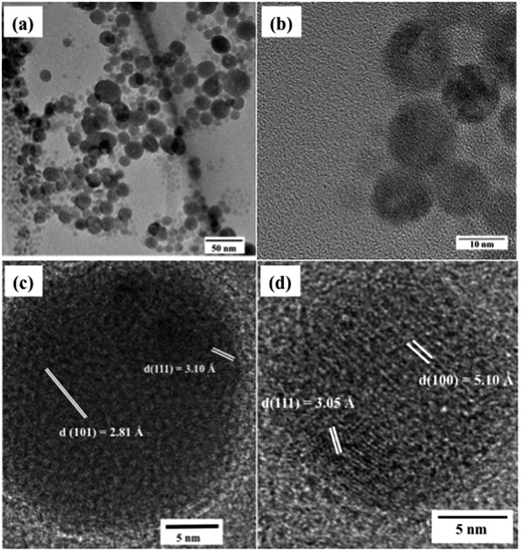 |
| Fig. 4 TEM (a and b) and HR-TEM (c) images of the ZnS:Mn/NaGdF4:Yb:Er nanocomposite. | |
Upconversion luminescence spectra of NaGdF4:Yb:Er and ZnS:Mn/NaGdF4:Yb:Er under excitation at 980 nm are shown in Fig. 5. The emission spectrum of ZnS:Mn/NaGdF4:Yb:Er was compared with that of NaGdF4:Yb:Er, to identify emission differences arising from the presence of the ZnS:Mn QDs. Fig. 5a shows the upconversion spectrum for NaGdF4:Yb:Er, which contained three major bands in the green and red regions. Green emissions at 522 and 542 nm corresponded to de-excitation of the 2H11/2–4I15/2 and 4S3/2–4I15/2 transitions, respectively. Red emission at 654 nm was ascribed to de-excitation of the 4F9/2–4I15/2 transition of Er ions in the NaGdF4 host.27 Similar emission bands were observed for different mole concentration of ZnS:Mn in the synthesis of ZnS:Mn/NaGdF4:Yb:Er composite, as shown in Fig. 5b–d. Differing intensities of the green and red emissions were observed in the spectra of NaGdF4:Yb:Er and ZnS:Mn/NaGdF4:Yb:Er. This indicated that energy transfer and retransfer occurred between erbium and manganese ions. Energy transfer between Mn2+ and Er3+ in the same host has been suppressed the green emission and enhancement in red emission was observed in previous studies.28 The increase of mole concentration of ZnS:Mn in ZnS:Mn/NaGdF4:Yb:Er composite periodically enhanced intensity of the upconversion red emission, which suggested that more number of Mn2+ ions in ZnS enhanced the red emission. Inter-band energy transfer and retransfer was observed in two different host lattices under upconversion excitation. The energy levels of 4F7/2, 2H11/2 and 4S3/2 of Er3+ occurred at the same energy as the 4T2(4G) and broad 4T1(4G) transitions of Mn2+, as shown in Fig. 6. According to Förster, inter-particle energy transfer can be occurred between luminescent particles closer less than 10 nm. ZnS:Mn/NaGdF4:Yb:Er composite formation is confirmed by TEM that NGF:Yb:Er particles have grown from the ZnS:Mn seeds. Based on this, there is a possible energy transfer between ZnS:Mn and NaGdF4:Yb:Er particles in ZnS:Mn/NaGdF4:Yb:Er composite, which enhanced the red upconversion emission upon the excitation of Yb3+.29–33
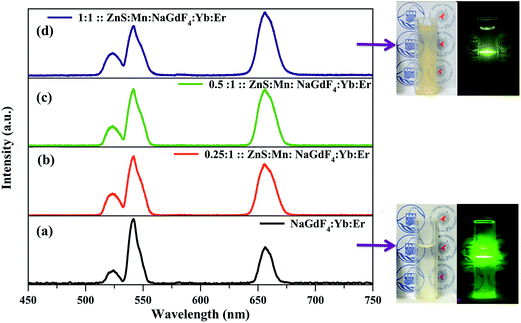 |
| Fig. 5 Upconversion luminescence spectra of NaGdF4:Yb:Er (a) and 0.25:1:ZnS:Mn/NaGdF4:Yb:Er (b), 0.5:1:ZnS:Mn/NaGdF4:Yb:Er (c), 1:1:ZnS:Mn/NaGdF4:Yb:Er (d), and corresponding particle dispersions under white light and 980 nm excitation. | |
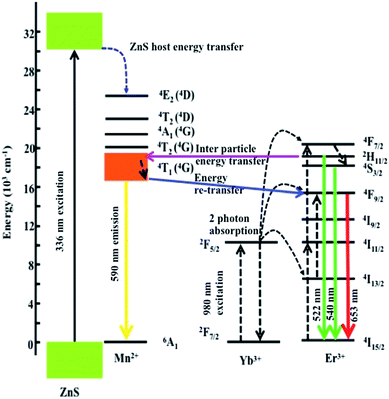 |
| Fig. 6 Mechanism of energy transfer between ZnS:Mn and NaGdF4:Yb:Er in ZnS:Mn/NaGdF4:Yb:Er. | |
Fig. 7 shows the downconversion photoluminescence excitation and emission spectra of ZnS:Mn and ZnS:Mn/NaGdF4:Yb:Er. In Fig. 7a, the band excitation centered at 336 nm was attributed to the principle excitation of the ZnS host lattice. Bands at 515 and 532 nm corresponded to the 6A1–4T2(4G) and 6A1–4T1(4G) excitations of Mn2+, respectively.34 Two significant emission bands at 404 and 587 nm were observed upon excitation at 336 nm (Fig. 7b). Blue emission was observed from the host ZnS, and orange emission resulted from energy transfer from ZnS to Mn2+.35 Fig. 7c shows broad excitation between 250 and 400 nm, and an overlapped small hump at ∼532 nm arising from Mn2+ excitation. A broad emission spectrum was observed for ZnS:Mn/NaGdF4:Yb:Er upon excitation at 336 nm (Fig. 7d).
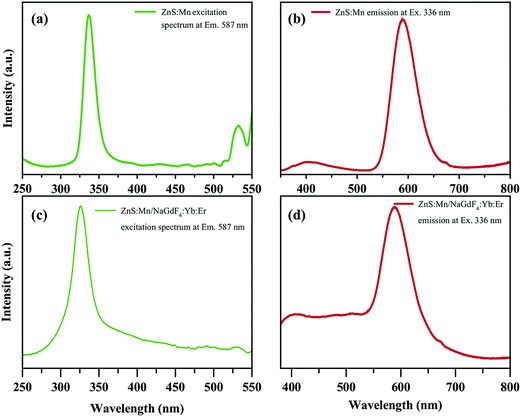 |
| Fig. 7 Excitation and downconversion emission spectra of the ZnS:Mn QDs (a, b) and ZnS:Mn/NaGdF4:Yb:Er nanocomposite (c, d). | |
CL measurements were performed to investigate the electroluminescence of the samples. ZnS:Mn, NaGdF4:Yb:Er and ZnS:Mn/NaGdF4:Yb:Er were coated on silicon substrates and allowed to dry, and were then used for experiments. The CL spectrum of the uncoated bare silicon substrate was subtracted from measurements. All samples were measured at 5 keV at ×200 magnification, and the recorded spectra are shown in Fig. 8. The CL spectrum of ZnS:Mn showed a broad emission at 590 nm, as shown in Fig. 8a. The CL spectrum of the NaGdF4:Yb:Er nanoparticles showed significant green (520 and 544 nm) and red (658 nm) emission, which were similar to the normal upconversion PL emission.36 Three low intensity bands at 310, 407 and 615 nm were also observed, corresponding to de-excitation of the 2G11/2–4I15/2, 2H9/2–4I15/2 and 4F7/2–4I15/2 transitions, respectively. High-energy excitation due to electron bombardment resulted in the blue and ultraviolet emission shown in Fig. 8b. The CL spectrum of ZnS:Mn/NaGdF4:Yb:Er in Fig. 8c shows the overlapped principle emissions of ZnS:Mn/NaGdF4:Yb:Er.37
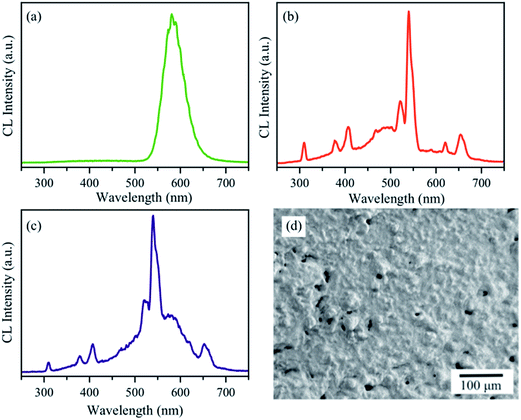 |
| Fig. 8 Cathodoluminescence spectra of ZnS:Mn (a), NaGdF4:Yb:Er (b), and ZnS:Mn/NaGdF4:Yb:Er (c) at ×200 magnification, and SEM image of the coated ZnS:Mn/NaGdF4:Yb:Er nanocomposite (d). | |
The cytotoxicity measurement of the synthesized samples was analyzed using Calcein and EthD-1 optimal dye to identify dead and alive cells by exciting suitable wavelength 490 and 550 nm, respectively. Obtained results of ZnS:Mn/NaGdF4:Yb:Er loaded HeLa cells were compared with blank HeLa cells as shown in Fig. 9. The results showed that the ZnS:Mn/NaGdF4:Yb:Er nanocomposite particles were bio compatible and viability of the HeLa cells with composite particles was 99.4%. Luminescence stability of the synthesized composite particles was measured in water and PBS liquid for different aging period such as 1 h, 3 days and 1 week. Luminescence of the aged ZnS:Mn/NaGdF4:Yb:Er composite particles in water and PBS liquid was examined in microscope under 365 nm excitation and luminescence intensities were compared in Fig. 10. The intensity/HWHM of the measured luminescence of aged particles were initially decreased by aging and stabilized after three days. Three days aged particles in water as well as PBS solution were examined with TEM analysis as presented in Fig. 11. TEM images indicated that the particles were gathered together due to soft agglomeration.
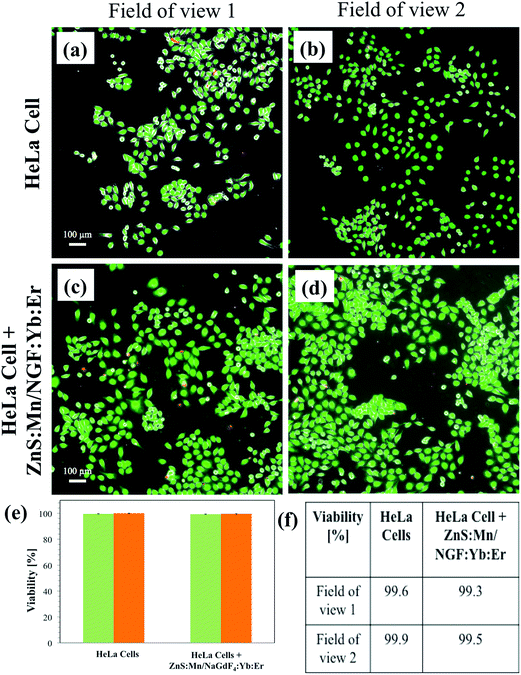 |
| Fig. 9 Cytotoxicity measurement of ZnS:Mn/NaGdF4:Yb:Er nanocomposite using calcein/EthD-1 optimal dyes, blank HeLa cells (a, b), HeLa cells with ZnS:Mn/NaGdF4:Yb:Er (c, d), viability comparison (e, f). | |
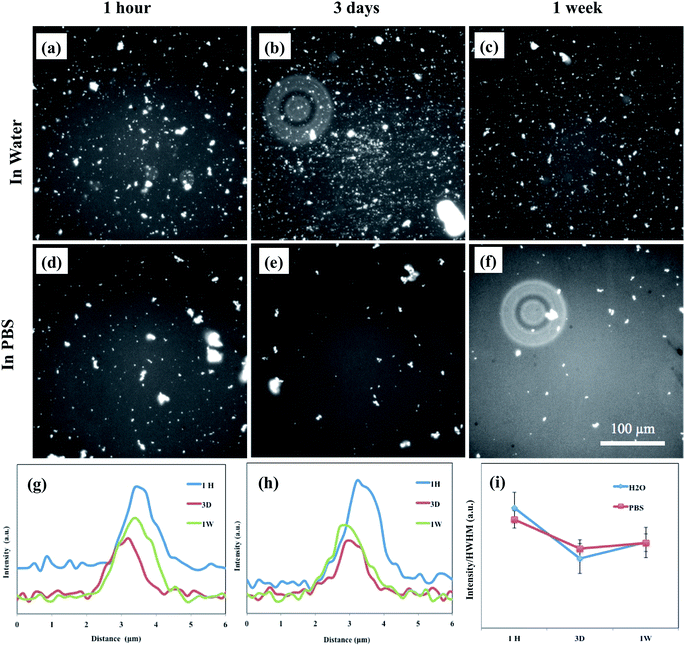 |
| Fig. 10 Luminescence stability measurement of ZnS:Mn/NaGdF4:Yb:Er in water (a–c), PBS (d–f), intensity comparison upon aging in water (g), in PBS (h) and intensity/HWHM with aging (i). | |
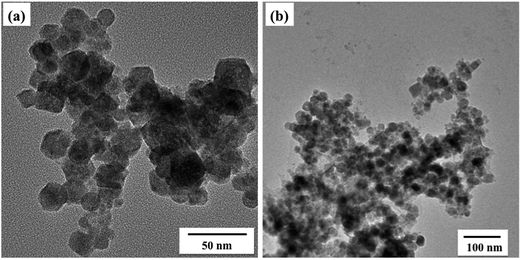 |
| Fig. 11 TEM image of ZnS:Mn/NaGdF4:Yb:Er nanocomposite particles after in water (a) and PBS (b) after three days aging. | |
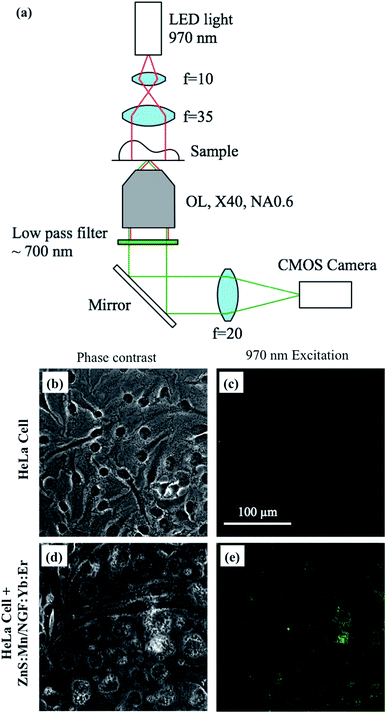 |
| Fig. 12 Schematic of upconversion optical apparatus involving excitation at 970 nm (a), phase contrast (b) and under 970 nm excitation images of HeLa cells without ZnS:Mn/NaGdF4:Yb:Er (c), and phase contrast (d) and upconversion luminescence images of HeLa cells incubated with ZnS:Mn/NaGdF4:Yb:Er (e). | |
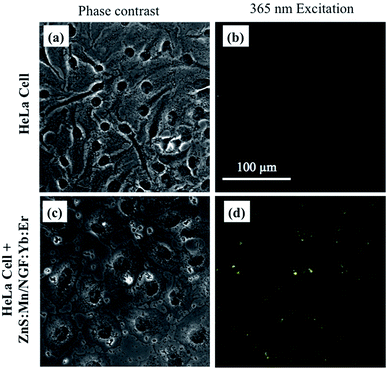 |
| Fig. 13 Phase contrast (a) and optical images under 365 nm excitation (b) of bare HeLa cells, and phase contrast (c) and downconversion luminescence images of HeLa cells incubated with ZnS:Mn/NaGdF4:Yb:Er (d). | |
The in vitro upconversion luminescence (970 nm excitation) imaging of cultured bare HeLa cells and HeLa cells incubated with the ZnS:Mn/NaGdF4:Yb:Er nanocomposite were examined, using a purpose-built optical setup. Fig. 12a shows a schematic of the upconversion luminescence imaging apparatus. Bare HeLa cells were excited at 980 nm for comparison. Fig. 12b and c show phase contrast and optical images under excitation at 980 nm, confirming no significant emission from the bare HeLa cells. Fig. 12d and e show clear emission from HeLa cells incubated with ZnS:Mn/NaGdF4:Yb:Er, upon excitation at 970 nm. Distinguished emission centers around HeLa cell nuclei indicated the presence of the composite nanoparticles. An outline of the nucleolus was evident in the image recorded under excitation at 980 nm. The same procedure was used for downconversion imaging, using a commercially available Olympus (IX71) microscope and excitation at 365 nm. Fig. 13a and b show phase contrast images of bare HeLa cells excited at 365 nm, in which no emission was observed. However, particles packed around the nucleus were observed in the cell images in Fig. 13c and d. Clear emission was observed under excitation at 365 nm. These results suggested that the ZnS:Mn/NaGdF4:Yb:Er nanocomposite could be used as an upconversion and downconversion luminescence imaging agent.
Conclusions
Manganese-doped ZnS QDs and NGF:Yb:Er nanoparticles were synthesized by thermal decomposition, and a ZnS:Mn/NaGdF4:Yb:Er luminescent nanocomposite was synthesized using a single-step hot injection method. The ZnS:Mn/NaGdF4:Yb:Er nanocomposite consisted of hexagonal NaGdF4 and zinc blend ZnS structures. Formation of the ZnS:Mn/NaGdF4:Yb:Er composite was confirmed from TEM and HRTEM images. Upconversion luminescence of the ZnS:Mn/NaGdF4:Yb:Er nanocomposite under excitation at 980 nm showed enhanced red emission, compared with the NaGdF4:Yb:Er nanoparticles. Downconversion emission experiments of the ZnS:Mn/NaGdF4:Yb:Er nanocomposite showed that the ZnS:Mn in the presence of NaGdF4:Yb:Er nanoparticles exhibited broad excitation and emission bands. CL spectra of the nanocomposite showed overlapping significant emissions of Mn2+ and Er3+. This indicated that high-energy electrons in the composite resulted in luminescence ranging from ultraviolet to infrared wavelengths. Downconversion and upconversion luminescence imaging results of HeLa cells incubated with ZnS:Mn/NaGdF4:Yb:Er showed that the nanocomposite is a useful multi-modal imaging probe.
Acknowledgements
This study was financially supported by a Grant-in-Aid for JSPS Fellows related to a JSPS Postdoctoral Fellowship for Foreign Researchers (No. 26-04050) from the Ministry of Education, Culture, Sports, Science and Technology of Japan. The authors thank Mr T. Koyama, Research Institute of Electronics, Shizuoka University, for assistance with XRD analysis.
References
- V. Biju, Chem. Soc. Rev., 2014, 43, 744–764 RSC.
- N. Erathodiyil and J. Y. Ying, Acc. Chem. Res., 2011, 44, 925–935 CrossRef CAS PubMed.
- R. Liang, M. Wei, D. G. Evans and X. Duan, Chem. Commun., 2014, 50, 14071–14081 RSC.
- D. E. Lee, H. Koo, I. C. Sun, J. H. Ryu, K. Kim and I. C. Kwon, Chem. Soc. Rev., 2012, 41, 2656–2672 RSC.
- M. Rai, S. K. Singh, A. K. Singh, R. Prasad, B. Koch, K. Mishra and S. B. Rai, ACS Appl. Mater. Interfaces, 2015, 7, 15339–15350 CAS.
- F. Erogbogbo, K. T. Yong, R. Hu, W. C. Law, H. Ding, C. W. Chang, P. N. Prasad and M. T. Swihart, ACS Nano, 2010, 4(9), 5131–5138 CrossRef CAS PubMed.
- O. S. Wolfbeis, Chem. Soc. Rev., 2015, 44, 4743–4768 RSC.
- A. Nagai, J. B. Miller, P. Kos, S. Elkassih, H. Xiong and D. J. Siegwart, ACS Biomater. Sci. Eng., 2015, 1, 1206–1210 CrossRef CAS.
- G. Chen, H. Qiu, P. N. Prasad and X. Chen, Chem. Rev., 2014, 114, 5161–5214 CrossRef CAS PubMed.
- B. A. Kairdolf, A. M. Smith, T. H. Stokes, M. D. Wang, A. N. Young and S. Nie, Annu. Rev. Anal. Chem., 2013, 6, 143–162 CrossRef CAS PubMed.
- R. Gill, M. Zayats and I. Willner, Angew. Chem., Int. Ed., 2008, 47, 7602–7625 CrossRef CAS PubMed.
- R. M. Amit, P. Sharbari, S. K. Basiruddin, S. K. Niladri, S. Suresh, P. Narayan and R. J. Nikil, Nanoscale, 2013, 5, 5506–5513 RSC.
- W. Zhang, Y. Li, H. Zhang, X. Zhou and X. Zhong, Inorg. Chem., 2011, 50, 10432–10438 CrossRef CAS PubMed.
- S. Cao, C. Li, L. Wang, M. Shang, G. Wei, J. Zheng and W. Yang, Sci. Rep., 2014, 4, 7510 CrossRef PubMed.
- F. Wang, D. Banerjee, Y. Liu, X. Chen and X. Liu, Analyst, 2010, 135, 1839–1854 RSC.
- W. Feng, X. Zhu and F. Li, NPG Asia Mater., 2013, 5(12), e75 CrossRef CAS.
- M. Haase and H. Schäfer, Angew. Chem., Int. Ed., 2011, 50, 5808–5829 CrossRef CAS PubMed.
- S. Wu, G. Han, D. J. Milliron, S. Aloni, V. Altoe, D. V. Talapin, B. E. Cohen and P. J. Schuck, Proc. Natl. Acad. Sci. U. S. A., 2009, 106, 10917–10921 CrossRef CAS PubMed.
- C. Liu, Z. Gao, J. Zeng, Y. Hou, F. Fang, Y. Li, R. Qiao and L. Shen, ACS Nano, 2013, 7, 7227–7240 CrossRef CAS PubMed.
- N. J. J. Johnson, W. Oakden, G. J. Stanisz, R. S. Prosser and F. C. J. M. Veggel, Chem. Mater., 2011, 23, 3714–3722 CrossRef CAS.
- S. Huang, M. Bai and L. Wang, Sci. Rep., 2013, 3, 3–7 Search PubMed.
- M. Y. An, J. B. Cui, Q. He and L. Y. Wang, J. Mater. Chem. B, 2013, 1, 1333–1339 RSC.
- J. C. Boyer, F. Vetrone, L. A. Cuccia and J. A. Capobianco, J. Am. Chem. Soc., 2006, 128, 7444–7445 CrossRef CAS PubMed.
- E. Sotelo-Gonzalez, L. Roces, S. Garcia-Granda, M. T. Fernandez-Arguelles, J. M. Costa-Fernandez and A. Sanz-Medel, Nanoscale, 2013, 5, 9156 RSC.
- S. Zheng, W. Chen, D. Tan, J. Zhou, Q. Guo, W. Jiang, C. Xu, X. Liu and J. Qiu, Nanoscale, 2014, 6(11), 5675 RSC.
- Z. Quan, Z. Wang, P. Yang, J. Lin and J. Fang, Inorg. Chem., 2007, 46, 1354–1360 CrossRef CAS PubMed.
- F. He, N. Niu, L. Wang, J. Xu, Y. Wang, G. Yang, S. Gai and P. Yang, Dalton Trans., 2013, 42, 10019–10028 RSC.
- G. Tian, Z. Gu, L. Zhou, W. Yin, X. Liu, L. Yan, S. Jin, W. Ren, G. Xing and S. Li, Adv. Mater., 2012, 24, 1226–1231 CrossRef CAS PubMed.
- A. Bednarkiewicz, M. Nyk, M. Samoc and W. Strek, J. Phys. Chem. C, 2010, 114, 17535–17541 CAS.
- D. Tu, L. Liu, Q. Ju, Y. Liu, H. Zhu, R. Li and X. Chen, Angew. Chem., Int. Ed., 2011, 50, 6306–6310 CrossRef CAS PubMed.
- S. Sarkar, B. Meesaragandla and C. Hazra, Adv. Mater., 2013, 25, 856–860 CrossRef CAS PubMed.
- S. Jiang and Y. Zhang, Langmuir, 2010, 26, 6689–6694 CrossRef CAS PubMed.
- F. Wang, R. Deng, J. Wang, Q. Wang, Y. Han, H. Zhu, X. Chen and X. Liu, Nat. Mater., 2011, 10, 968–973 CrossRef CAS PubMed.
- X. J. Wang, R. J. Xie, B. Dierre, T. Takeda, T. Suehiro, N. Hirosaki, T. Sekiguchi, H. Li and Z. Sun, Dalton Trans., 2014, 43, 6120–6127 RSC.
- J. H. Yu, S. H. Kwon, Z. Petrášek, O. K. Park, S. W. Jun, K. Shin, M. Choi, Y. Park, K. Park and H. B. Na, Nat. Mater., 2013, 12, 359–366 CrossRef CAS PubMed.
- Z. L. Wang, J. H. Hao and H. L. W. Chan, J. Mater. Chem., 2010, 20, 3178 RSC.
- M. Galceran, M. C. Pujol, J. J. Carvajal, X. Mateos, P. Formentín, J. Pallarès, L. F. Marsal, K. H. Park, F. Rotermund and K. Kim, Nanoscale Res. Lett., 2013, 8, 385 CrossRef PubMed.
|
This journal is © The Royal Society of Chemistry 2016 |